Version abrégée
Les opérations menées dans le cadre du programme Joint Global Ocean Flux Study (JGOFS), ont révélé le rôle essentiel joué par les diatomées dans l'exportation de carbone organique vers l'océan profond et de façon ultime vers les sédiments, autrement dit dans le contrôle de la pompe biologique de dioxyde de carbone. À la différence des autres espèces autotrophes, ces algues unicellulaires ont besoin de silicium pour assurer la croissance de leur enveloppe siliceuse. La préservation de la silice biogène amorphe (= opale) dans les sédiments étant environ dix fois plus élevée que celle du carbone organique, l'opale est potentiellement un excellent traceur de la paléoproductivité primaire. Aussi, pour mieux comprendre le cycle du carbone dans l'océan moderne et ses variations dans le paléocéan, est-il particulièrement utile de bien décrire et modéliser le cycle biogéochimique du silicium. Récemment, la résolution de deux problèmes importants, l'un à l'échelle régionale (océan Austral), l'autre à l'échelle globale, permet d'illustrer ce point de vue.
Les sédiments abyssaux de l'océan Austral (figure 1) sont connus pour leurs abondants dépôts d'opale (>50 % du total mondial). Paradoxalement, la production photosynthétique de l'océan Austral, essentiellement supportée par les diatomées, était située jusqu'à présent au plus faible niveau de l'océan mondial (figure 1). Comment accumuler en abondance de la silice biogène dans les abysses antarctiques si la production est à ce point limitée à la surface de l'océan ? Les chercheurs supposaient que l'opale était exceptionnellement bien conservée dans l'Antarctique par rapport au reste de l'océan mondial. Qu'en est-il en fait ?
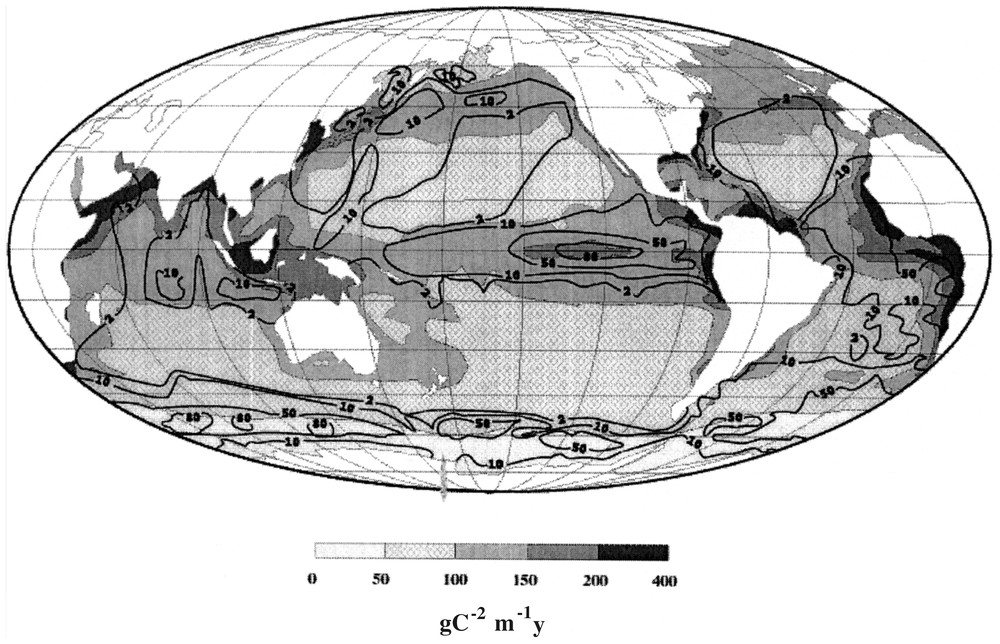
World ocean: distribution of primary productivity [1] in the surface reservoir (see legend) and of opal deposits (%) in decarbonated sediments [4]. Different from the east equatorial Pacific, the opal rich sediments of the Southern Ocean are located beneath poor productive waters: this is the so-called ‘opal paradox’.
Océan mondial : répartition de la production primaire [1] dans la couche de surface (voir légende) et du dépôt d'opale (en %) dans les sédiments décarbonatés [4]. Dans le Pacifique est-équatorial, les sédiments riches en opale (= silice biogène) correspondent à une zone productive en surface. En revanche, dans l'océan Austral, les sédiments riches en opale sont localisés à des eaux de surface peu productives : c'est le « paradoxe de l'opale».
La production primaire et la production de silice biogène dans la couche de surface étaient déterminées jusqu'à présent à l'échelle journalière, à partir de mesures locales réalisées à l'aide de techniques isotopiques ; de ces mesures locales sont déduites des estimations de la production primaire annuelle ou de la production de silice annuelle, à l'échelle régionale, par extrapolations « raisonnables » dans l'espace et dans le temps. Depuis quelques années, nous disposons, pour estimer la production dans la couche de surface, de techniques plus adéquates. D'une part, grâce aux capteurs de la couleur de la mer (ex : SeaWIFs) embarqués à bord de satellites, nous pouvons déterminer une meilleure distribution de la teneur en chlorophylle à la surface de l'océan et en déduire des estimations régionales plus réalistes de la production primaire annuelle, même si la couverture nuageuse importante pour l'océan Austral limite l'accès à ce type de données. D'autre part, nous disposons, grâce notamment aux campagnes océanographiques Antares France–JGOFS (1993–1999), de bilans saisonniers de carbone inorganique dissous et de sels nutritifs (nitrates, phosphates, acide silicique = silicates) dans la couche de surface. Corrigés de l'advection horizontale, de la diffusion turbulente verticale et du recyclage dans la couche de surface, ces bilans permettent de déduire la production primaire de carbone, d'azote, de phosphore et de silicium à l'échelle annuelle. Enfin, nous disposons des sorties de modèles couplés physiques-biogéochimiques, validés grâce à la série temporelle Kerfix dans l'océan Austral, pour chiffrer les flux biogènes dans la couche de surface. Ces trois approches méthodologiques montrent sans ambiguı̈té que la production primaire de l'océan Austral avait été sous-évaluée d'un facteur 2 à 3 environ dans le passé et qu'elle est proche de 100 g C·m−2·an−1. La même conclusion est valable pour la production de silice biogène dans l'océan Austral.
Des bilans régionaux des flux d'opale enfouis dans les sédiments abyssaux au niveau et au sud du front polaire (en moyenne au sud de 50°S) ont été établis à partir de carottes de sédiments. En général, ces carottes sont prélevées dans des vallées sous-marines, plus faciles à échantillonner que les sommets ; aussi l'extrapolation de mesures locales à des zones au relief tourmenté peut poser problème, surtout quand les courants de fond redistribuent les sédiments, les accumulant dans les dépressions naturelles aux dépens des sommets. Dans ce cas, le flux de matériel biogène enfoui dans un site donné n'est pas nécessairement corrélable à celui généré dans la couche de surface sur la verticale du site. Nous disposons aujourd'hui d'une méthode pour corriger les effets de cette redistribution (ce que les Anglo-Saxons appellent le focusing). En effet, lors de leur sédimentation rapide depuis la couche de surface, les matières particulaires adsorbent des métaux tels que le thorium radioactif, généré à partir de l'uranium dissous, naturellement présent dans l'eau de mer. Au cours de ce processus, les matières particulaires en sédimentation « nettoient » (les Anglo-Saxons parlent de scavenging = ébouage) la colonne d'eau du thorium dissous, qui se retrouve donc en phase particulaire, s'accumulant finalement dans les sédiments. Sur la verticale d'un site donné, dans un volume d'eau d'une colonne de 1 m2 de large, un sédiment ne doit normalement pas accumuler plus de thorium qu'il n'en a été produit par l'uranium présent dans ce volume d'eau. Si c'est le cas, c'est que les courants de fond ont apporté par advection du matériel sédimentaire non généré sur la verticale du lieu. L'excès de radioactivité dû au thorium permet donc de corriger le flux sédimentaire de cette redistribution par advection, c'est-à-dire de le corriger du focusing. Les flux sédimentaires abyssaux mesurés pour le secteur indien de l'océan Austral pendant les campagnes Antares ont été revisités et corrigés grâce à cette technique. Les conclusions sont particulièrement importantes : les flux de matériel sédimentaire accumulé pendant l'Holocène, en particulier les flux d'opale, avaient été surévalués d'un facteur 4 à 5. Finalement, les rapports de préservation de l'opale (rapports du flux d'opale dans les sédiments sur le flux de production de silice biogène à la verticale du lieu) dans les sédiments antarctiques ne sont pas, pour l'océan moderne, plus élevés qu'ailleurs ; il n'y donc pas de paradoxe antarctique de l'opale.
De cette première étude, nous pouvons retenir qu'à l'époque actuelle la production primaire et les flux de matière exportée, support de la pompe biologique de CO2 sont importants dans l'océan Austral. Qu'en était-il au dernier maximum glaciaire et cette fois pour l'océan mondial ?
Au dernier maximum glaciaire, la teneur en CO2 atmosphérique était d'environ 40 % inférieure au niveau de l'ère moderne pré-industrielle. Pour interpréter ce fait, plusieurs mécanismes, faisant appel aux interactions océan–atmosphère sont proposés. Nous retiendrons une interprétation biogéochimique. En 1988, John Martin proposait « l'hypothèse du fer » pour expliquer le faible niveau du CO2 atmosphérique. Des carottes collectées dans la calotte polaire antarctique révèlent, en effet, que le dernier maximum glaciaire coı̈ncide avec une augmentation spectaculaire des apports éoliens de fer à l'océan (figure 2). Cette hypothèse paraissait d'autant plus solidement établie que les preuves d'une limitation en fer de la production primaire s'accumulent, au moins pour certains régions de l'océan mondial à l'époque actuelle. Pourtant, à regarder de près la figure 2, le temps de relaxation du CO2 atmosphérique après la disparition de l'apport massif de fer océanique est lent, de l'ordre de la dizaine de milliers d'années. Or, si le fer commande la pompe biologique de CO2, c'est un temps de relaxation de l'ordre de la centaine d'années (temps de résidence du fer dans l'océan) qui est attendu. Toujours en privilégiant l'hypothèse biogéochimique, on se souvient alors que le temps géochimique de renouvellement du silicium dans l'océan mondial est précisément de l'ordre de la dizaine de milliers d'années. Et si un apport massif de silice dissoute (acide silicique) par voie éolienne était responsable du phénomène ? « L'hypothèse du silicium » paraı̂t au moins aussi fondée que celle du fer : une fraction du matériel lithogénique silicaté transporté par voie éolienne peut se dissoudre dans la couche de surface de l'océan, augmentant d'autant la teneur en silicium dissous. À noter qu'un apport fluvial accru de silicates, également possible à cette époque, a le même effet. Cette disponibilité accrue en silicium favorise les diatomées (algues microscopiques à frustule siliceux), au détriment des coccolithophoridés (algues microscopiques à carapace de carbonate de calcium). Or, la formation du carbonate de calcium à partir d'hydrogénocarbonate et de calcium dissous aboutit à la libération de dioxide de carbone. Un apport éolien massif de matériel lithogénique et/ou un apport fluvial accru de silicates se traduit indirectement par une diminution du flux net de CO2, de l'océan vers l'atmosphère, et donc par une décroissance de la teneur en CO2 atmosphérique. Cette diminution de CO2 atmosphérique n'exige nullement une augmentation de la production primaire totale de l'océan : elle est simplement due à un changement dans la dominance des espèces phytoplanctoniques. Du fait de la production accrue de diatomées, principal support de l'exportation de carbone vers l'océan profond, elle s'accompagne également d'une grande exportation de carbone, augmentant d'autant la pompe biologique de CO2.
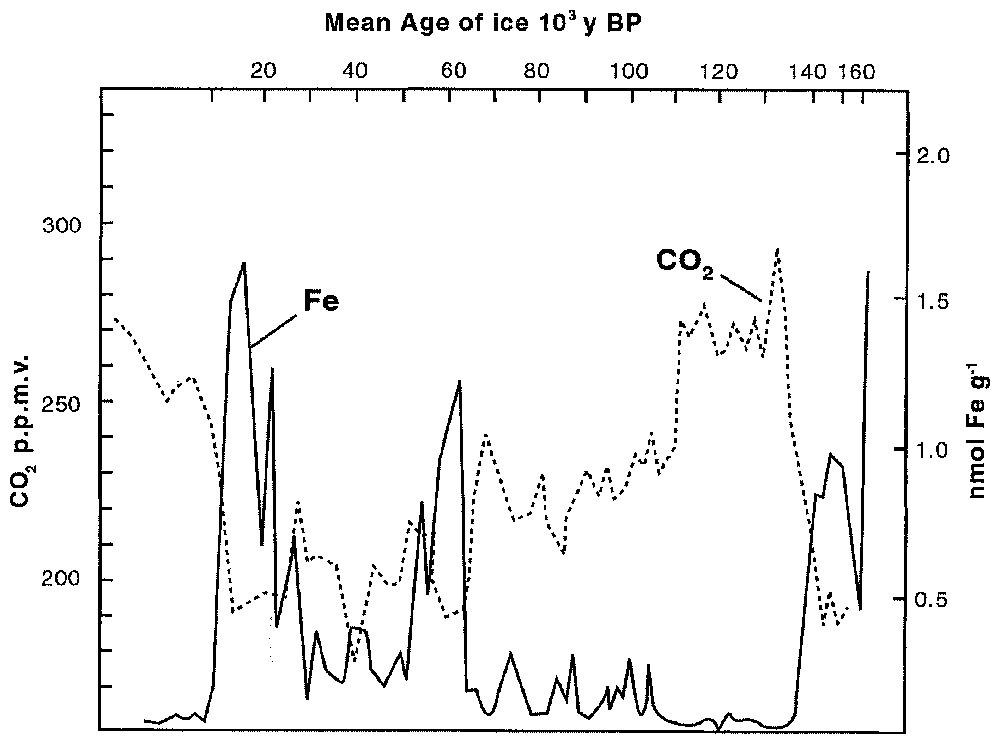
The variations of atmospheric CO2 (parts per million volume) and of wind-borne iron inputs (nanomol Fe per gram of ice) versus time, as recorded by the Antarctic ice cores at the Vostok site [18]. Note that during the Last Glacial Maximum (about 18 000 years) the concentration of CO2 was about 40 % lower than during the pre-industrial age. This coincided with anomalously high iron inputs.
Variations de la teneur atmosphérique en CO2 (en parties par million en volume) et des apports éoliens en fer (en nanomoles de fer par gramme de glace) en fonction du temps, enregistrés par les glaces de l'Antarctique (station Vostok), d'après [18]. Noter que le dernier maximum glaciaire (vers ans) correspond à des concentrations en CO2 de 40 % inférieures au niveau de l'époque préindustrielle. Ceci a coı̈ncidé avec des apports de fer particulièrement abondants.
1 Introduction
Silicon is the second element in relative abundance of Earth's crust (the lithosphere), but it is only a minor contributor to the chemical composition of the biosphere. Long ago, however, was silicon recognized as an essential element for life (review in [12]), its real importance in the marine cycle of carbon being revealed during the last decade [29,30]. This importance is hereafter illustrated through two case studies.
2 Resolving the Antarctic ‘opal paradox’
The Southern Ocean is well known for its circumpolar opal belt deposited in the sediments beneath and south of the Polar Front (figure 1): the total opal burial flux in the Antarctic abysses is estimated [8,29] at about 4 < Tera (=1012) mol Si·yr−1, which represents 2/3 of the total flux of the world's oceans. Sedimented opal is coming from the surface layer of the oceans, where biogenic silica is synthesised when siliceous phytoplankton (mostly diatoms) build up their frustules by pumping silicic acid (dissolved silica) from the surface layer. Where high opal deposits are found in sediments high primary production is expected in the surface layer. Paradoxically however, long ago the Southern Ocean was seen as the lowest productive area of the world's oceans. Although nutrient-rich, its total annual primary production (including siliceous + non siliceous species) was estimated at about 1–2 Gt C·yr−1 (review in [23]), i.e. only between 2 and 4 % of the marine total. This Antarctic ‘opal paradox’ was explained by assuming an exceptionally high rate of preservation for the biogenic silica deposited in the Southern Ocean sediments. In support to this view, the production of biogenic silica in the Southern Ocean was estimated [19] at only about 20 Tmol Si·yr−1. If this is correct, the preservation ratio of opal in the Antarctic sediments (burial rate/production rate of biogenic silica) is 4/20 =20 %, i.e. much more than the 3 % average typical of the world's oceans [30].
Today new data collected in the Indian sector of the Southern Ocean during the ANTARES and KERFIX programmes of France–JGOFS show [22] that both the biogenic silica production and the primary production in the surface layer have been underestimated, on the one hand, and that the opal burial rate in sediments has been overestimated, on the second hand. Updated estimates for the biogenic silica production are based on seasonal differences of silicic acid concentrations in the surface layer (figure 3). Different from nitrate and phosphate, severe depletions of the Si(OH)4 concentrations have been evidenced for the Indian sector [21] as well as for the Atlantic and Pacific sector (e.g., [16,22,26]). These seasonal differences, corrected from the vertical transfer of silicic acid from the subsurface layer by eddy diffusivity, from the south–north lateral advection of silicic acid from the Antarctic divergence, and from the Si recycling within the surface layer due to biogenic silica dissolution, are used to calculate the biogenic silica production (the so-called ‘Pondaven's method’ [22]) for different subsystems of the Southern Ocean [28]. This production is 2.43±0.39, 3.34±0.54, and 1.62±0.58 mol Si·m−2·yr−1, for the permanently open ocean zone (POOZ), the polar front zone (PFZ) and the seasonal ice zone (SIZ), respectively. Interestingly, for the POOZ this estimate is consistent with the outputs of a physical–biogeochemical model [21], which has been validated by the data of the KERFIX time-series. Note that this new estimate of biogenic silica production for the POOZ is 3–4 times higher than the upper limit of 1 mol Si·m−2·yr−1 proposed by [19]. From nitrate seasonal differences Pondaven's method [22] also gives access to estimates of the primary production for nitrate then transformed in carbon primary production using the C/N Redfield ratio. Interestingly these new primary production estimates are also in good agreement with recent satellite based estimates [2]. Note that Pondaven's new estimate [22] for the primary production of the POOZ is 2–3 times higher than previous estimates proposed for this area [28]. So: (1) although not very productive compared to its high nutrient potential, the primary production of the Southern Ocean is significantly higher than previously thought, and (2) the biogenic silica production and the export production had been underestimated by a factor of about 3.

Indian sector of the Southern Ocean (ANTARES France–JGOFS program). a. Latitudinal variations of the surface concentration of nitrate (in μmol) and silicate (= silicic acid in μmol) from 48° to 68°S, along a 62°E transect: winter values (filled squares and circles) and summer values (squares and circles). b. Vertically integrated depletion of nitrate and silicate (= silicic acid), in mol·m−2, deduced from a (integration from the surface to the depth of the mixed layer: dashed line), see [22]. PFZ = polar front zone, PF = polar front, POOZ = permanently open ocean zone, WIC = northernmost limit of the winter ice cover, SIZ = seasonal ice zone. Zones and fronts are according to [28].
Secteur indien de l'océan Austral (programme Antares France–JGOFS). a. Variations latitudinales des concentrations des nitrates (en μmol) et silicates (= acide silicique, en μmol) dans les eaux de surface de 48°S à 68°S le long de 62°E : valeurs hivernales (carrés et ronds noirs) et estivales (carrés et ronds blanc). b. Appauvrissements saisonniers (en mol·m−2) des nitrates (cercles) et des silicates (carrés), déduits des valeurs précédentes (voir a), intégrées sur la couche de surface (voir [22]) dont la profondeur est indiquée en m (en tiretés). PFZ = zone polaire frontale, PF = front polaire, POOZ = ocean ouvert en permanence, WIC = limite nord saisonnière de la banquise, SIZ = zone saisonnière des glaces. Ces zones et fronts sont définies selon [28].
The opal accumulation rates in the opal belt around Antarctica may be affected by post-depositional redistribution by bottom currents known to be active in the Southern Ocean. This bottom redistribution results in over-accumulation of sedimented material in valleys and canyons, called ‘sediment focusing’. The 230Th method [13] provides a mean to correct from focusing. Briefly, 230Th is the daughter of 238U. Because the half-life time of 238U is much higher than that of 230Th, the activities of the two radioisotopes in seawater are equal and the two radioactive disintegrations are in equilibrium. Contrary to 238U, 230Th is easily adsorbed on biogenic particles settling down in the water column, so that they are in excess in sediments compared to 238U. Of course this 230Th excess cannot be higher than the total amount of 238U present in the water column above the sediment, except if extra re-suspended material is imputed by focusing. Normalizing the opal flux registered in the ANTARES study area by the 230Th method gives opal burial rate of 0.075±0.042 mol Si·m−2·yr−1 for the PFZ, and of 0.210±0.040 mol Si·m−2·yr−1 for the POOZ, which is two times lower than previously thought. So the new estimates for overall preservation efficiency of opal are 3.1±2.2 % for the PFZ and 6.3±2.2 % for the POOZ. Pondaven's new estimates [22] are close to the 3±1 % global mean and well below the 20 % previous estimate [30] for the Southern Ocean. Finally, the opal preservation in the Antarctic is not significantly different from the rest of the world's oceans. The take-home message is that the high opal accumulation in the Antarctic abysses is due to the high export of biogenic material to the deep ocean, supported by high production rates of biogenic silica in the surface water. In other words there is no opal paradox in the Southern Ocean!
This work has two major implications. First, in the modern Southern Ocean, the primary production and the export production of carbon are higher than expected, which means that the biological pump of CO2 is more active than previously thought, which actually is in agreement with one relevant output of Schlitzer's inverse model for the world's oceans [24]. Second, for the Last Glacial Maximum (LGM), very high opal accumulations have been shown in sediments and intense biological pump of CO2 postulated (e.g., [17]). But, if the burial rates are corrected from focusing, they might be lower than initially thought, i.e. the export production and the biological pump of CO2 might be lower than previously thought, or in the control of atmospheric CO2 the biology might not be the driving force, at least for the Southern Ocean. But what was the situation of the rest of the world ocean during the LGM? This is the second case study we would like to introduce.
3 Silica winds to control atmospheric CO2
The concentration of CO2 in the atmosphere drastically depends on CO2 exchanges between the atmosphere and the ocean (e.g., [25]), driven either by physical and/or by biological mechanisms. It is out of the scope of this paper to review these different mechanisms and we herein focus on one important biogeochemical pathway that controls the fluxes of CO2 between the atmosphere and the surface ocean CO2 reservoirs. The biological pump of CO2 is responsible for a net transfer of CO2 as particulate and dissolved organic carbon, from the atmosphere to the surface ocean reservoir, and ultimately to the ocean interior and to the sediments. Large-celled phytoplankters such as diatoms and coccolithophorids manage most of this transfer of carbon (e.g., [5]). In this transfer however the role of diatoms and that of coccolithophorids is not exactly equivalent. Indeed, like any phytoplankter coccolithophorids need CO2 for particulate organic carbon photosynthesis. But when building up their frustules to produce calcite (by reaction between dissolved calcium and hydrogen carbonate) cocolithophorids are counter-intuitively CO2 producers. This dissolved CO2 flux can escape from the ocean surface to the atmosphere. So, when diatoms predominate over coccolithophorids the net flux of CO2 from the atmosphere to the ocean surface is much larger than when cocolithophorids predominate over diatoms. Obviously predominance of diatoms over non-siliceous phytoplankters requests a sufficient concentration of silicic acid (= dissolved silica) in the surface reservoir. At time scale of 15 000 years this concentration depends on riverine inputs of silicic acid as well as of Si aeolian inputs [30]. About 5 % of the silica dust delivered to the surface ocean by winds dissolves in surface ocean mixed layers [10] and becomes available for biological uptake.
It is well established that the concentration of atmospheric CO2 rose from about 190 to 280 ppmv, i.e. increased by 40 %, during the Last Glacial to Interglacial transition. It has been suggested [18] that massive aeolian inputs of iron especially to the Southern Ocean surface waters might have dramatically boosted the marine photosynthetic activity during the Last Glacial Maximum (LGM) compared to the modern ocean, where the primary productivity is severely iron-limited (e.g., [3]). Indications of massive wind-borne dust deliveries to the Southern Ocean and to Antarctica during the LGM are supported by numerous data, based on both cores from deep-sea sediments and from polar ice sheets (figure 2), on the one hand. Abundant opal burial fluxes during the LGM have also been shown in Antarctic sediments (e.g., [4]) on the other hand, suggesting that the export of biogenic matter was more important during the LGM compared to now. In other words, the biological pump of CO2 was more active, because the iron limitation of the primary production has been suppressed during the LGM.
While the ‘iron hypothesis’ has proven to be very useful to explain the low primary productivity of the high nutrient low chlorophyll (HNLC) areas of the modern world's oceans, its validity in periods in the past is questionable. Indeed after the massive dust delivery to the Southern Ocean, it took about 8 000 years for the concentration of atmospheric CO2 to increase to about 280 ppmv. If this CO2 change was strictly under iron biological control it should have been phased with the residence time of iron in the ocean, i.e. at a time scale of a few decades [27]. Although we do not exclude iron's contribution, it is clear that iron alone cannot account for the variations of atmospheric CO2 during the Last Glacial–Interglacial transition. Silicon, whose residence time scale in the ocean is about that of the change in atmospheric CO2 during this transition, seems to be a better candidate than iron in the control of the variations in atmospheric CO2.
Harrisson points out that, according to [10] and [30], there is an aeolian pathway of the control of the concentration of silicate in the surface waters of the world's oceans. Challenging the ‘iron hypothesis’, he pushes up the ‘silica hypothesis’ [15]. Indeed, dusts transported by winds are mostly lithogenic material, i.e. are mostly composed of lithogenic siliceous materials, which dissolve at least partly [10] in the ocean and becomes available for biological uptake [30] (figure 4). So, the maximum flux of iron so typical of the LGM, actually corresponds to a maximum flux of lithogenic silica, increasing the silicate inputs as well as those of iron to the ocean wind-mixed layer. Actually during the LGM, not only was the wind-borne flux of silica dust different from that prevailing under interglacial conditions, but very likely the flux of silicate from equatorial and tropical rivers to oceans was also much higher [14]. So the two pathways contributed to the increase of the net inputs of silicate to the surface ocean.
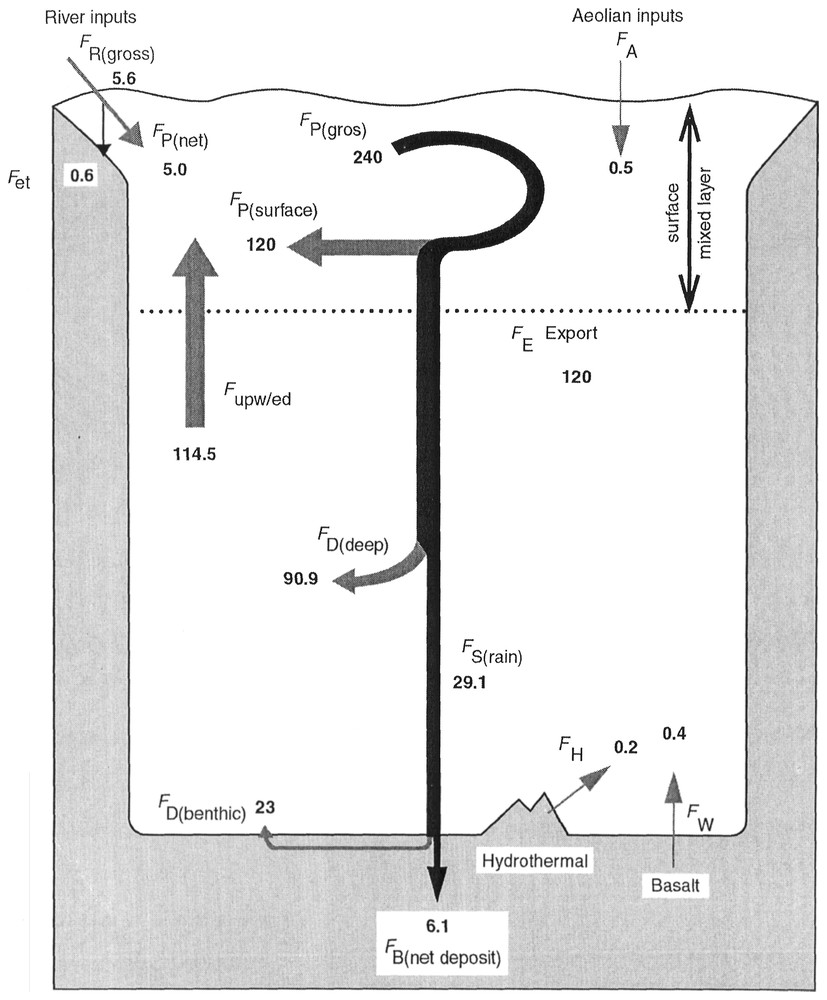
The biogeochemical cycle of Si in the world's oceans [29]: fluxes of Si in tera (=1012) mol Si·yr−1. Si inputs to the ocean: FR(gross) = river gross inputs, FR(net) = river net inputs, FA = aeolian inputs of dissolved silica, FW = seafloor weathering inputs, FH = hydrothermal inputs. Biogenic fluxes: FP(gross) = biogenic silica gross production brute, FE Export = flux of biogenic silica exported toward the deep reservoir, FS(rain) = opal rain rate, FB(net deposits) = opal burial rate in sediments. Recycling of Si: FD(surface) = Si recycled in the surface reservoir, FD(deep) = Si recycled in the deep reservoir, FD(benthic) = Si recycled at the sediment-water interface. Fupw/ed = Si flux from the deep to the surface reservoir (upwelling + diffusion).
Le cycle biogéochimique du silicium dans l'océan mondial [29] : flux en tera (=1012) moles de Si·an−1. Apports de silicium à l'océan : FR(gross) = flux fluviaux bruts, FR(net) = flux fluviaux nets, FA = flux éoliens de silice dissoute, FW = flux basaltique (lessivage des basaltes à froid), FH = flux hydrothermaux. Flux biogènes : FP(gross) = production brute de silice, FE Export = production exportée, FS(rain) = pluie d'opale au sédiment, FB(net deposits) = flux nets d'opale accumulée dans les sédiments. Recyclage : FD(surface) = flux de silicium recyclé (par dissolution de la silice biogène) dans le réservoir de surface, FD(deep) = flux de silicium recyclé dans le réservoir profond, FD(benthic) = flux de silicium recyclé à l'interface eau–sédiment. Fupw/ed = flux de silicium transféré du réservoir profond vers le réservoir de surface par résurgence ou diffusion turbulente.
Because silicate is needed by diatoms to build up their siliceous frustules, increasing silicate availability in the surface ocean during the LGM favoured the growth of diatoms, which became able to outcompete with the non-siliceous phytoplankters and especially with cocolithophorids. Assuming a constant global marine primary production, the net total inputs of silicate to the ocean needed to provoke a decrease of 40 % of the outgasing flux of CO2 engendered by the formation of CaCO3 by marine cocolithophorids, is about 9.6 Tmol Si·yr−1 [15]. This is about 60 % higher than for the modern ocean [19]. Keeping constant the riverine Si inputs requests an increase by a factor of 7.4 of the aeolian flux of dust, which is well supported by the data [15]. Actually increased riverine inputs of silicate during the LGM [14] would have similarly impacted the ocean and provoked an atmospheric CO2 drawdown. Note that phosphorus (and not by iron or by silicate) is the ultimate limiting factor of the total marine primary production; because we have no indication of changes of P inputs to the ocean, the total marine primary production was assumed to be constant from the LGM to Holocene. To account for the 40 % change in CO2 flux, only a change in the phytoplankton species dominance is requested [30].
In addition to this direct effect on the net fluxes on CO2 between the atmosphere and the surface ocean, using Tyrell's model [31] it has been shown [30] that the predominance of diatoms over the non siliceous species during the LGM also resulted in an increase by 33 % of the export production of carbon towards the ocean interior, i.e. an increase of the biological pump of CO2. So the two processes (fewer CO2-generating non-siliceous species and increased export of carbon) can explain the lower level of atmospheric CO2 during the Last Glacial Maximum.
4 Conclusions
These two case studies demonstrate that silicon is a key element for marine biogeochemists. Actually silicon is with iron a nutrient whose importance in the control of the biogeochemical cycle of carbon has been established during the last decade of the 20th century. If we want to better understand the variations of the biogeochemistry of carbon in the past and to predict its changes in the future, we have to take into consideration that the biogeochemical cycle of silicon is of major importance.
Acknowledgements
Thanks are due to P. Pondaven, O. Ragueneau, L. Dezileau, J.-L. Reyss for their valuable contributions to these case studies.