1 Introduction
Metal and metalloid pollutants are of particularly critical environmental concern as they can accumulate throughout the food web and induce severe health problems (e.g., Boyd, 2010). Some mineral phases abundant in nature offer huge reactive surfaces and can sorb or release metal pollutants depending on chemical conditions prevailing in the environment (e.g., Stumm et al., 1994). They can also catalyze redox reactions modifying the speciation of inorganic pollutants, and hence their mobility (e.g., Borch et al., 2010; Gadd, 2010). One goal of environmental mineralogists is to understand which mineral phases can impact the mobility of pollutants in various environmental settings, such as freshwater or soil systems, how they form, and finally how stable they are under changing environmental conditions. As a result it is possible to predict how modifications of environmental conditions may foster pollution events or to design efficient remediation strategies. Understanding interactions between minerals and metal pollutants has long been restricted to studies of abiotic systems.
However, it has been increasingly shown over the last 20 years that microorganisms, in particular bacteria, can impact significantly the mobility of metals as well (e.g., Warren and Ferris, 1998). Microorganisms are widespread even in the most extreme environments, including those with high metal concentrations (e.g., acid mine drainage, AMD) (Belnap et al., 2010; Denef et al., 2010; Xie et al., 2009). They comprise a high biomass including extracellular polymers offering very large reactive surface areas and high turnover rates (Nealson, 1997; Nealson and Stahl, 1997; Warren and Haack, 2001). In addition, they can catalyze a wide diversity of reactions, including redox reactions that can alter dramatically the speciation of metals and enhance or reduce their mobility (Borch et al., 2010; Newman and Banfield, 2002). As a consequence, microorganisms can stimulate or reduce pollutions. Two emblematic examples are the generation of acid mine drainages involving microbially catalyzed oxidation of metal sulfides in mine tailings (e.g., Elberling et al., 2000; Fowler et al., 2001) and massive contamination of groundwaters in SE Asia by arsenic released by microbially mediated reductive dissolution of As-bearing iron oxides (Islam et al., 2004; Polizzotto et al., 2008). Alternatively, microorganisms can form minerals by a process called biomineralization, which offers an interesting bioremediation strategy for capturing pollutants within relatively stable solid phases. Biomineralization occurs naturally in very diverse environments but may be artificially stimulated for bioremediation purposes as proposed in several past studies (e.g., Beazley et al., 2007; Senko et al., 2002; Yabusaki et al., 2007). However, in order to optimize this approach, assess its potential limitations and avoid possible drawbacks, we need to better understand mineral nucleation and growth processes in the presence of microorganisms, better assess the importance of biomineralization in natural environments and determine which specific microbial actors are involved, and consider the impact of biomineralization on the biology of microorganisms.
The present review, far from being exhaustive, highlights some recent advances in the field and provides non-specialists with an introduction to some of the main approaches and some of the unanswered questions
2 Mechanisms of microbial biomineralization in polluted environments
Mechanisms involved in biomineralization have been extensively covered by many previous reviews to which readers are referred for additional details (Bäuerlein, 2000; Lowenstam, 1981; Mann, 2001; Weiner and Dove, 2003). Notably, eukaryotes such as diatoms (Poulsen et al., 2003; Sumper and Brunner, 2008), foraminifers (Erez, 2003), and corals (Cuif and Dauphin, 2005; Meibom et al., 2008;Stolarski, 2003) have received much more attention than prokaryotes (i.e., both bacteria and archaea) in the biomineralization literature. This partly results from the common assumption that eukaryote biomineralizing systems are usually determined genetically and form more complex, hence interesting, biomineral structures, while prokaryotes have a passive indirect role in mineral precipitation. However, prokaryotes, owing to the diversity of metabolic reactions they can catalyze, are able to induce the formation of minerals that are much more diverse chemically including oxides, oxi-hydroxides, carbonates, phosphates, sulphates, and sulphides, which results in the trapping of various metal and metalloids. As a result, their impact on the global mobility and biogeochemical cycling of metals in the environment is likely stronger than that of eukaryotes, although the latter can contribute to some extent (e.g., Brake and Hastotis, 2008; Gelabert et al., 2007).
Three different types of bacterially mediated biomineralization processes have been distinguished in the literature (Dupraz et al., 2009): (1) biologically controlled biomineralization, referring to cases in which a specific cellular activity directs the nucleation, growth, morphology, and final location of a mineral; the emblematic example for that process in the case of bacteria is the formation of intracellular chemically pure magnetite crystals in magnetotactic bacteria (e.g., Bazylinski and Frankel, 2004; Blakemore, 1975; Komeili, 2007), but no significant impact on immobilization of metal pollutants has been reported so far; (2) biologically induced biomineralization resulting from indirect modification of chemical conditions, such as a pH shift or redox transformations, in the environment by biological activity; many examples are reported in the literature for this process and can lead to efficient immobilization of almost any known inorganic pollutant (e.g., Borch et al., 2010; Lowenstam, 1981); and (3) biologically influenced biomineralization, which is defined as passive mineral precipitation in the presence of organic matter, such as cell surfaces or extracellular polymeric substances (EPS), whose properties influence crystal morphology and composition. The term organomineralization encompasses biologically influenced and biologically induced biomineralization (Dupraz et al., 2009). Although by definition organomineralization involves processes that are not supposed to involve specific genes, they may have a negative or positive impact on cell viability as discussed later. An increasing number of studies have shown that microbial extracellular polymers can be involved in biomineralization, hence inorganic pollutant trapping (e.g., Benzerara et al., 2008; Chan et al., 2009; Miot et al., 2009b) (Fig. 1).
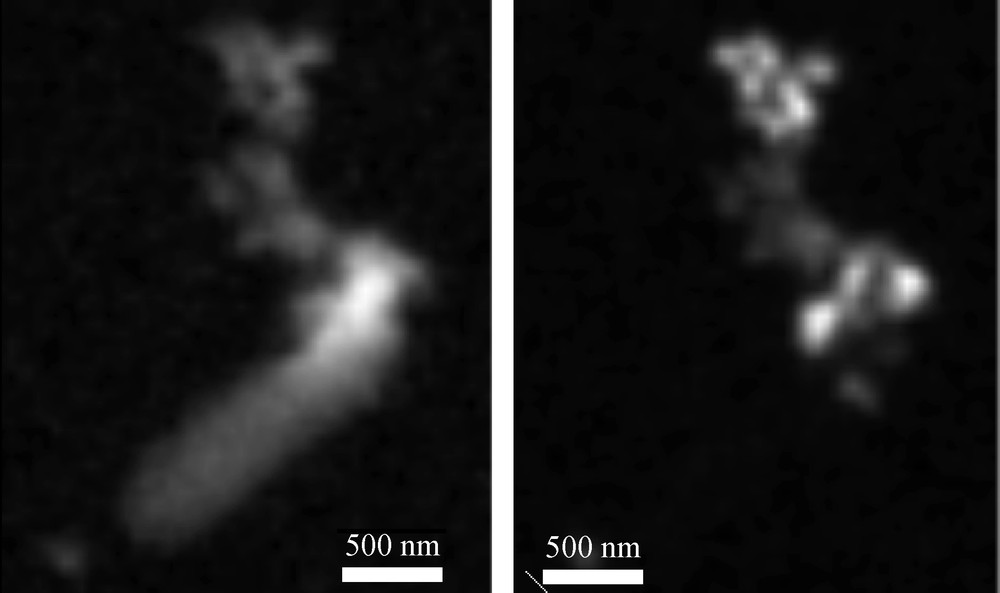
STXM analyses of a single SW2 cell with mineralized extracellular fibres (3-day old culture). Left: organic carbon map showing the cell and a filament at its tip. Right: map of Fe(III) (goethite) showing that biomineralization is restricted to the filament and does not affect the cell itself. Study of the filaments by XANES at the C K-edge suggests that they are composed of a mixture of partly unsaturated lipids and polysaccharides.
Analyse STXM d’une cellule de la souche SW2 montrant des filaments extracellulaires minéralisés (après trois jours de culture). À gauche : cartographie du carbone organique montrant la cellule et un filament à son apex. À droite : cartographie du Fe(III) (goethite) montrant que la biominéralisation est restreinte au filament et n’affecte pas la cellule. L’étude des filaments par XANES (spectroscopie d’absorption des RX au voisinage du seuil K du carbone suggère qu’ils sont composés d’un mélange de lipides insaturés et de polysaccharides.
Microorganisms can impact mineral formation in several distinct ways (De Yoreo and Vekilov, 2003). They can modify the composition of the solution so that it becomes supersaturated or more supersaturated than it previously was with respect to a specific phase. For example, iron-oxidizing bacteria oxidize metabolically Fe(II) into Fe(III). As Fe(III) is highly insoluble at neutral pH, it precipitates spontaneously, typically in the form of an oxyhydroxide such as ferrihydrite or goethite. As a consequence, inorganic metal pollutants can be trapped by co-precipitation or adsorption at the surfaces of these Fe-biominerals (Hohmann et al., 2010). Manganese oxides are also often formed by the oxidative activity of some microbes and have a significant impact on pollutant metals (Bargar et al., 2009; Francis and Tebo, 2002; Spiro et al., 2010; Tebo et al., 2005). In addition to oxidative and reductive processes, a pH increase due to metabolic activities such as photosynthesis, sulphate-reduction, or urea degradation can lead to mineral precipitation such as carbonation, for example resulting in the capture of common groundwater contaminants such as strontium, uranium, or copper (Warren and Haack, 2001). Finally, release of inorganic phosphates resulting from the degradation of organic phosphates by microbial phosphatases is another significant microbial process involved in the trapping of inorganic pollutants. It leads to precipitation of various mineral phases containing inorganic pollutants such as Cr(III)-phosphates (Goulhen et al., 2006), Fe(II)- and/or Fe(III)-phosphates (Miot et al., 2009b), U(VI)-phosphates (Beazley et al., 2007; Jroundi et al., 2007; Macaskie et al., 1992), or Pb-phosphates (pyromorphite, Templeton et al., 2001).
Such a microbial impact is sometimes referred to as indirect, but this designation does not indicate whether the resulting biominerals are directly associated with cells or not. Metabolic reactions often occur within cells or cell walls creating microenvironments that are locally highly supersaturated.
In these cases, precipitates are thus expected to form around cells and one may imagine that counting the type and number of mineral grains associated with cells may provide an effective way of quantifying the impact of microorganisms on mineral precipitation. However this approach does not work because it may first be difficult to distinguish minerals precipitated in situ on microbe surfaces from secondary minerals become attached to microbial polymers (Glasauer et al., 2001). Moreover, some microorganisms may have developed strategies to localize precipitation away from the cells either by producing extracellular enzymes or extracellular mineral templates (Chan et al., 2009; Miot et al., 2009c). Recently, Miot et al. (2009b), used a combination of transmission electron microscopy (TEM) and synchrotron-based scanning transmission x-ray microscopy (STXM) to study oxidation of Fe and the resulting biomineralization by nitrate-reducing and Fe-oxidizing bacteria cultured in the laboratory. They showed that some part of the Fe-phases precipitate within the periplasm of the bacteria, i.e., where Fe-oxidation is catalyzed (Fig. 2). This fraction of the Fe-containing precipitates would be easy to quantify even in natural samples. However, Miot et al. (2009b) also found evidence for additional Fe oxidation occurring outside the cells mediated by nitrites that are produced by reduction of nitrates at the cell surface (Miot et al., 2009a). This fraction would be in contrast much more difficult to relate to the microbial action in the environment and might be overlooked.

TEM images of biomineralizing Fe-oxidizing bacteria showing different biomineralization patterns. (A) SW2, a phototrophic strain forms extracellular precipitates under the form of vesicles. Bright field mode. (B) BoFeN1, a nitrate-reducing strain forms Fe-minerals encrusting its periplasm (bright crown all around the cell). High angular annular dark field (HAADF) mode in scanning transmission electron microscopy.
Image MET de bactéries ferroxydantes biominéralisantes avec différents schémas de biominéralisation. (A) SW2, une souche phototrophe forme des précipités extracellulaires sous la forme de vésicules. Mode champ clair. (B) BoFeN1, une souche dénitrifiante forme des minéraux de fer encroûtant le périplasme (couronne claire tout autour de la cellule). Le mode champ sombre annulaire à grand angle (HAADF) a été utilisé en microscopie électronique par transmission à balayage (STEM).
A second means by which microorganisms can impact mineral formation is through the production of organic polymers, which can impact nucleation by favoring (or inhibiting) the stabilization of the very first mineral seeds. As mentioned above, supersaturation is necessary but not always sufficient for precipitation to occur (Nesterova et al., 2003; Williams, 1984). Nucleation usually involves a significant barrier or activation energy that has to be overcome (De Yoreo and Vekilov, 2003). When this is the case, the process is referred to as heterogeneous nucleation and this explains why mineral phases may often be closely associated with organics, either cell surfaces and/or extracellular polymeric substances (EPS) produced by microorganisms. Diverse organic polymers, including peptides (Moreau et al., 2007; Schultze-Lam et al., 1996), lipids (Collier and Messersmith, 2001), polysaccharides (Chan et al., 2009) or lipo-polysaccharides (Miot et al., 2009c), can favor heterogeneous nucleation in various environmental contexts. Moreover, a continuum exists between adsorption of metal ions on functional groups of microbial EPS and biomineralization (Warren and Ferris, 1998) in a similar way to the continuum existing between adsorption and precipitation of metals at mineral surfaces. As a consequence, there is a competition between mineral and microbial surfaces for the sorption of inorganic pollutants. Templeton et al. (2003) elegantly probed the distribution of aqueous Pb(II) sorbed at the interface between hematite and microbial biofilms grown at the mineral surface. They showed that reactive sites on the metal oxide surfaces were not passivated by the formation of a monolayer biofilm consisting of Gram-negative Burkholderia cepacia and that depending on metal concentration in the solution mineral or biofilm was the dominant sink. How the association between biominerals and organic molecules affects the stability and mobility of mineral phases is a very important issue that must be considered. On the one hand, the presence of biofilms on mineral surface may not have any effect on the reactivity of mineral surfaces and hence their stability towards dissolution (Templeton et al., 2003). Alternatively, some organic molecules that bind strongly to mineral surfaces may weaken catio-ligand bonds and hence promote mineral dissolution (e.g., Johnson et al., 2005). Finally, organic polymers can promote the aggregation of nanoparticles, which may affect their reactivity and limit the dispersal of pollutant-containing nanoparticles (Gilbert and Banfield, 2005; Moreau et al., 2007). These processes will have to be better evaluated in the future.
Finally, life can impact mineral growth. Traditionally, growth takes place layer by layer. Organisms can inhibit crystal growth along certain directions by production of poisoning molecules, resulting in the formation of minerals with particular shapes (e.g., Orme et al., 2001). Alternatively, nuclei may aggregate, forming mesocrystals, sometimes with the mediation of an organic matrix (e.g., Colfen and Antonietti, 2005; Sethmann et al., 2006). This mechanism has been increasingly identified in biomineralizing systems (Gilbert et al., 2009). However, processes leading to mesocrystal formation, in particular the role of organics, have yet to be fully understood.
3 Importance of biomineralization in natural environments and identity of the microbes involved
As shown previously all microorganisms can impact the mobility of inorganic pollutants by providing surfaces where they can sorb. As mentioned previously, resulting biominerals are not always strongly connected to cells, and thus it is difficult to assess the amount of minerals precipitated by microbial mediation. Specific groups of microbes catalyzing particular metabolic reactions can impact further the mobility of inorganic pollutants by modifying their speciation (e.g., redox state). These microbes can be identified phylogenetically, with inherent difficulties, using cultivation-dependent or -independent approaches (e.g., Baker and Banfield, 2003). However, it is not clear whether few specialized species are more efficient than very diverse microbial assemblages in the trapping of inorganic pollutants. It is now well known that phylogenetic identification of the species present at a field site does not provide a definitive answer as to what mineralogical processes they mediate. Recent genomic advances, however, enable complete sequencing of the genome of a whole natural microbial population, as well as the monitoring of gene expression and determining how communities function and how they shape and are shaped by their environments (e.g., Newman and Banfield, 2002; Tyson et al., 2004). Alternatively, one recent study by Sharp et al. (2009) compared the mineral products of uranyl reduction mediated by different dissimilatory metal- and sulfate-reducing bacteria under similar laboratory conditions. They showed that these diverse strains lead to the precipitation of similar (chemically, structurally and in size) uraninite crystals, regardless of phylogenetic or metabolic diversity. Future studies of diverse sites that determine functional traits (such as quantity of removed arsenic or Fe-oxide precipitated) and biodiversity indicators would also help to assess whether a potential correlation exists between microbial diversity and the efficiency of inorganic pollutant trapping. One example is provided below.
The importance of biomineralization in the sequestration of inorganic pollutants is obvious, especially at field sites where the metabolic activity of microorganisms has been artificially stimulated for bioremediation purposes (e.g., Adriano et al., 2004; Barkay and Schaefer, 2001). A group of studies have, for example, clearly shown that the secretion of phosphate groups due to phosphatase activity can induce a significant immobilization of uranium in the form of autunite at acidic and alkaline pH (Beazley et al., 2009; Macaskie et al., 2000; Merroun and Selenska-Pobell, 2008; Nilgiriwala et al., 2008). Phosphatase activity can be stimulated by injection of glycerol-3-phosphate as the sole carbon and phosphorus source. However, the precipitation of minerals is not always related in such a simple way to a single enzymatic process, and the involvement of microorganisms in the trapping of inorganic pollutants is then more difficult to assess.
Acid mine drainages (AMD) provide additional natural examples of mitigation of inorganic pollutants. The Carnoulès AMD (Gard, France) is strongly enriched in arsenic (80–350 mg.l−1) upstream (Leblanc et al., 1996). At 30 m downstream, 20–60% of the As has been removed by the formation of As-rich (up to 22 wt% As) sediments. These sediments are rich in ferric iron hydroxysulfates (Morin et al., 2003). The role of microbes in the formation of these minerals has been assessed using several approaches including measurements of seasonal variations of element concentrations (Casiot et al., 2003), cultivation-independent study of microbial diversity (Bruneel et al., 2006), and cultivation under biomineralizing conditions (e.g., Duquesne et al., 2003; Morin et al., 2003). These different approaches showed that the bacterial diversity is quite low, which is usual for this kind of environment and may reflect the limited number of electron donors and acceptors available in AMD and the toxicity of heavy metals (Bruneel et al., 2006). Nevertheless, several bacterial strain were identified that may promote the precipitation of Fe- and As-containing mineral phases including neutrophilic and acidophilic Fe-oxidizing bacteria such as Gallionella ferruginea, Acidithiobacillus ferrooxidans as well as Fe- and As-oxidizing bacteria such as Thiomonas sp. Moreover, it has been reported that microbial populations vary spatially and seasonally and that this might correlate with variations in minerals that are precipitated and hence As trapping rates (e.g., Casiot et al., 2003, 2005; Morin et al., 2003). These observations highlight a possible correlation between microbial diversity and efficiency of inorganic pollutant sequestration. Finally, a microscopy study was conducted on Carnoulès samples to detail the microbial processes that are involved in the mediation of Fe- and As-containing mineral phases (Benzerara et al., 2008). This study showed that some mineral phases are associated directly with microbial cells showing a variety of biomineralization patterns, forming either extracellularly or within the periplasm (Fig. 3). However, most of the mineral phases at Carnoulès were not associated with cells but with pervasive organic carbon interpreted as extracellular polymeric substance (EPS) produced by cells. Finally, abundant biomineralized organic vesicles resembling the outer membrane vesicles produced by Gram negative bacteria (Mashburn-Warren et al., 2008; Schooling and Beveridge, 2006) were documented and may represent a significant biomineralization process in AMD systems that has been overlooked so far.

TEM analysis of mineralized vesicles and microbial cells in Carnoules samples. (A) TEM images (Bright field mode) of the vesicles observed on ultramicrotomy sections of the Carnoules samples. Note that some are associated closely with bacterial cells (bottom right hand corner). (B) TEM images of a cluster of vesicles at higher magnification (deposited drop). The thick electron dense wall is visible on this picture. Elemental analyses by EDXS and characterization of the speciation of carbon by XANES spectroscopy at the C K-edge are available in Benzerara et al. (2008).
Analyse MET de vésicules minéralisées et de cellules microbiennes dans les échantillons de Carnoulès. (A) Images MET (champ clair) de vésicules observées sur des coupes en ultramicrotomie dans des échantillons de Carnoulès. Notez que certaines sont intimement associées aux cellules bactériennes (coin en bas à droite). (B) Images MET d’un agrégat de vésicules à plus fort grandissement (dépôt de goutte). La paroi épaisse dense aux électrons est visible sur cette image. Des analyses élémentaires par EDXS et la caractérisation de la spéciation du carbone par spectroscopie XANES au seuil K du carbone sont disponibles dans Benzerara et al. (2008).
4 Impact of biomineralization on the biology of microorganisms.
This section focuses on a much less understood aspect of microbial biomineralization. We have mentioned that microbial diversity may play an important role in affecting the efficiency of inorganic pollutant sequestration. Alternatively, biomineralization may have an impact on microbial communities. Many studies have claimed that biologically induced biomineralization is detrimental to cells. However, when inorganic pollutants such as uranium or lead are involved, the same process is suggested to provide resistance to the toxicity of these elements (Levinson et al., 1996; Mire et al., 2004). This issue has been discussed by Phoenix and Konhauser (2008).
Biomineralization may be lethal for cells due to the formation of mineral phases that may hinder nutrient exchange between cells and the surrounding environment or due to the disruption of cellular membranes. If this lethality is high, one should expect that biomineralization is a strong stress in some highly mineralizing environments and that the microbial population inhabiting these environments may have thus developed specific strategies to localize mineralization away from cells (Hallberg and Ferris, 2004; Hegler et al., 2008). As a result some strains may be able to control biomineralization processes that may be considered to a first approximation as being “biologically-induced”.
Assessing the lethality of biomineralization is not trivial and has been poorly addressed in various studies to date. For example, BoFeN1, a nitrate-reducing, iron-oxidizing bacterial strain, promotes precipitation of Fe-phases within the periplasm, resulting eventually in a complete encrustation of most of the cells as observed by TEM (Miot et al., 2009b). Although complete encrustation seems incompatible with a viable state, Muehe et al. (2009) recently showed that BoFeN1 grows better in the presence than in the absence of Fe, although encrustation occurs in the presence of Fe. This observation suggests that encrustation is not a major stressor in such cultures. One way to reconcile these findings is to assume that encrustation starts only when cells are dead and/or that encrusted cells accumulate over time because they cannot be lysed while viable cells progressively become a limited proportion of the total amount of cells as estimated by TEM. This example shows that addressing the impact of biomineralization on cell viability is not a trivial task.
Interestingly, a number of studies have addressed the problem of the viability of microbial cells immobilized in mineral matrices (e.g., Livage and Coradin, 2006; Nassif et al., 2002). They have shown that more bacteria remain culturable in mineral gels than in an aqueous suspension and that the metabolic activity of the bacteria could also be preserved after several weeks of association with mineral matrices. Whether this kind of mineral matrix, usually silica gels, can be compared to nano-biominerals encrusting cells has to be ascertained; however, these studies clearly raise questions about how precipitation of mineral phases may impact cell viability.
5 Conclusions
In this short review, we have shown the importance of developing a better understanding of how microorganisms impact the formation of minerals in the context of developing remediation strategies for inorganic pollutants. Microbial biomineralization is not only a concern in environmental mineralogy but also in geobiology through the study of ancient biominerals that store unique information on ancient environments (e.g., Benzerara and Menguy, 2009) or in medical sciences through the study of calcifications occurring in the human body (e.g., Benzerara et al., 2006). In all these cases, one has to study minerals as well as microorganisms at the same time. This implies the combination of tools and knowledge produced by microbiology, molecular biology and modern mineralogy. Major questions remain completely open, such as: (1) the specificity and genetic control of the diverse biomineralization mechanisms; (2) the stability of biominerals, especially how their association with organic molecules might alter their reactivity and their transport in the environment, (3) finally, many studies have focused on biominerals formed under anoxic conditions, likely because of the variety of metal reactants that can be found under such conditions. Interesting complementary strategies however would consist in promoting inorganic pollutant sequestration by biomineralization under oxic conditions. These diverse fundamental issues promise a thrilling future for biomineralogy.
Acknowledgements
We gratefully acknowledge Georges Calas and Gordon E Brown Jr for soliciting us for this manuscript and managing the whole editorial process. We thank Elodie dePeretti for preparation of SW2 and BoFeN1 samples for TEM analyses. We thank Martin Obst, Florian Hegler and Andreas Kappler for past discussions on Fe-oxidizing bacteria.