1. Introduction
Sea-level rise is one of the most certain consequences of anthropogenic climate change (Fox-Kemper et al., 2021). Due to global warming, glaciers and ice-sheets are melting and adding mass to the ocean, and the ocean is accumulating heat and expanding. Additionally, surface water and groundwater management also contributes to sea-level changes, albeit to a lesser magnitude. Globally, sea level is projected to rise at rates between 5 to 30 mm/year by the end of the 21st century depending on greenhouse gas emissions and the response of ice-sheets to climate change (ibid.). In fact, sea levels are projected to continue rising for decades and centuries. Hence, mitigating climate change can only increase the probability of slower rates of sea-level changes, but sea-level rise itself cannot be stopped over the foreseeable future.
Contemporary sea-level change is taking place in an unprecedented context in human history because the ongoing urbanization of coastal zones has resulted in higher than ever exposure to coastal hazards such as coastal flooding, erosion and salinization. Globally, approximately 900 million people, or 11% of the world’s population, are currently living below 10 m above sea levels (Haasnoot et al., 2021). In France, the focus of this article, the population density in coastal municipalities is 2.5 times greater than the national average (MTECT, 2023a). Exposure to sea-level rise is not only a concern for coastal regions: it is also projected to affect human and economic activities indirectly due to trade being highly dependent on ports and harbors (Hanson and Nicholls, 2020; Verschuur et al., 2022).
To summarize, both the certainty of future sea-level rise and the growing exposure to coastal hazards contribute to increase coastal risks and adaptation needs.
To support coastal adaptation planning, sea-level scenarios are increasingly considered (Nicholls, Hanson, et al., 2014). In Europe, such scenarios support a large panel of adaptation strategies, including the production of maps to limit urban expansion in high-hazard areas or virtual stress tests of critical coastal infrastructures such as estuarine barriers (Bednar-Friedl et al., 2022; McEvoy et al., 2021). Designing and adopting such scenarios is not that simple. On the one hand, sea-level rise displays regional and local variability due to ocean density and circulation changes, atmospheric pressure variations and due to the gravitational, rotational and deformational effects of water and ice mass redistributions as well as vertical land motions that can affect some areas locally (Slangen, Adloff, et al., 2017). On the other hand, the utility of sea-level scenarios can be increased by considering their intended application. For example, adaptation planning of nuclear plants may consider scenarios involving an early collapse of the west Antarctica ice-sheet, even if their likelihood is very low, because damages to such infrastructures cannot be accepted. Recognizing the variety of needs, previous national scenarios have considered a spread of scenarios rather than a single value (McEvoy et al., 2021; Palmer et al., 2024; Weeks et al., 2023).
In this paper, we present sea-level scenarios in support of the third French adaptation plan to climate change. Since 2011, France is considering a unique sea-level scenario of 60 cm with respect to the end of the 21st century in order to support the implementation of its coastal risk prevention plans (Le Cozannet and Cazenave, 2024). To support its third adaptation plan, the Ministry in charge of Environment requested an update of this unique scenario considering regional sea-level variations. Interestingly, the ministry requested a unique scenario aligned with global warming levels (GWL) reaching 3 °C above the preindustrial era by 2100 (MTECT, 2023b). One reason for selecting a specific scenario instead of a range of values could have been to simplify the message for users, as suggested in the document presenting the proposed national scenario (ibid.). Thus, this choice could aim to maximize stakeholder engagement in adaptation planning, including those without advanced knowledge of climate change.
A first research question is therefore to understand, contextualize and refine this national scenario of 3 °C of global warming (Cabana et al., 2023), in order to design a method that responds to the needs of this policy. This is addressed in Section 2.
A second question is to develop the sea-level scenario itself, which requires regionalizing sea-level rise scenarios dependent of global temperatures, including beyond 2100. While such scenarios have been delivered in the 6th Assessment report (AR6) of the IPCC (Intergovernmental Panel on Climate Change), their projections are not directly useable for two reasons: (1) first, vertical land motions other than those due to the Glacial Isostatic Adjustment are computed using the non-climate signal in tide gauges; this approach misses local ground motions not captured by tide gauges and reduces the confidence in coastal projections, as acknowledged page 1301 of the Working Group 1 report (Fox-Kemper et al., 2021); (2) the processing of uncertainties in AR6 is not similar before and after 2100, leading to breaks in rates and uncertainties of sea-level rise projections at this time horizon. This difficulty needs to be addressed as the French regulation explicitly requires to consider sea-level rise over the coming century, that is, up to 2125. The methods used to produce sea-level scenarios are presented in Section 3 and the results are shown in Section 4.
A final question related to the production of these scenarios is to understand to what extent they can support coastal adaptation effectively. The utility of sea-level scenarios can be compromised due to their intrinsic limitations or by ambiguities in their intended use. In Section 5, we discuss the decision and policy context in which they will be used and show that the lack of shared and explicit objectives of coastal adaptation is a major issue that will require policy decision.
2. Methods and data
2.1. Refining the French climate scenarios
The third national adaptation plan of France is being prepared since 2022. One of the elements supporting this plan is a climate scenario (TRACC, for “trajectoire de réchauffement de réference pour l’adaptation au changement climatique” or “reference warming scenario supporting climate change adaptation”, hereafter “French climate scenario” or “TRACC”). The TRACC is currently described in the technical document supporting the consultation on the French climate scenario that was openned in 2023 (MTECT, 2023b). In this document, two scenarios were considered:
- A first scenario in which global climate warming is stabilized at 1.5 °C GWL above preindustrial levels. This scenario is aligned with the objectives of the Paris agreement.
- A second scenario in which global climate warming reaches 3 °C GWL above preindustrial levels by 2100 and then is stabilized at this level. This scenario is presented as an average between the commitments made by the countries and the policies in place in 2020. It is referred to as the “3 °C scenario” hereafter, though the ministerial communication also refers to a “4 °C scenario”, which is approximately the level of warming in France for 3 °C of global warming.
This 3 °C scenario was selected by the ministry to serve as a reference for adaptation.
It can be noted that as it is presented in the consultation document (ibid.), the 3 °C scenario is not fully aligned with the current scientific knowledge. First, the Working Group III of the 6th Assessment report of the IPCC and the 2022 emission gap report shows that unconditional nationally determined contributions, and, a fortiori, the policies in place, are not sufficient to reach net-zero emissions by 2100 (IPCC, 2022; UNEP, 2022). Consequently, the climate will continue to warm after 2100 if these policies and contributions are implemented. This also poses practical issues to design sea-level scenario because most climate scenarios available today that reach 3 °C GWL in 2100 assume further warming beyond 2100. Based on these arguments, we removed this constraint of a stabilization of climate change at 3 °C GWL and developed sea-level scenario that make no assumption regarding warming beyond 2100.
One assumption underlying these scenarios is that impacts of climate change will be similar for a given level of warming whatever the precise time horizon by which that level of warming is reached and as long as climate change is stabilized at that particular global warming level. This assumption is true for many impacts of climate change, as illustrated by the burning embers in the IPCC reports (Zommers et al., 2020), but it does not hold for sea-level rise due to the latency of the response of the ocean and ice to global warming. To resolve this issue, we tied each level of global warming to specific time horizons. The refinements to these scenarios are presented in Table 1 below.
Climate scenarios used in this study
Time horizon | Global warming above the pre-industrial period (1850–1900) | |||
---|---|---|---|---|
“1.5 °C” scenario | French “3 °C” adaptation scenario | |||
Global mean | Mainland France | Global mean | Mainland France | |
2030–2040 | 1.5 °C | 2 °C | 1.5 °C | 2 °C |
2050 | 1.5 °C | 2 °C | 2 °C | 2.7 °C |
2100 | 1.5 °C | 2 °C | 3 °C | 4 °C |
2150 | 1.5 °C | 2 °C | Not specified | Not specified |
These scenarios are refined from the French climate scenarios. Because the ministerial and media communication has used levels of warming in mainland France instead of global warming levels, we present both global warming levels and their implications in France to help the French readers find their way.
2.2. Methods to produce sea-level scenarios consistent with the French climate scenarios
The approach to develop sea-level scenarios presented in this paper is shown in Figure 1. The overall principle is to select model outcomes that meet the assumptions of Table 1 for each contribution to sea-level rise, to regionalize these contributions and finally sum them up (Jackson and Jevrejeva, 2016). This subsection presents the details of this approach.
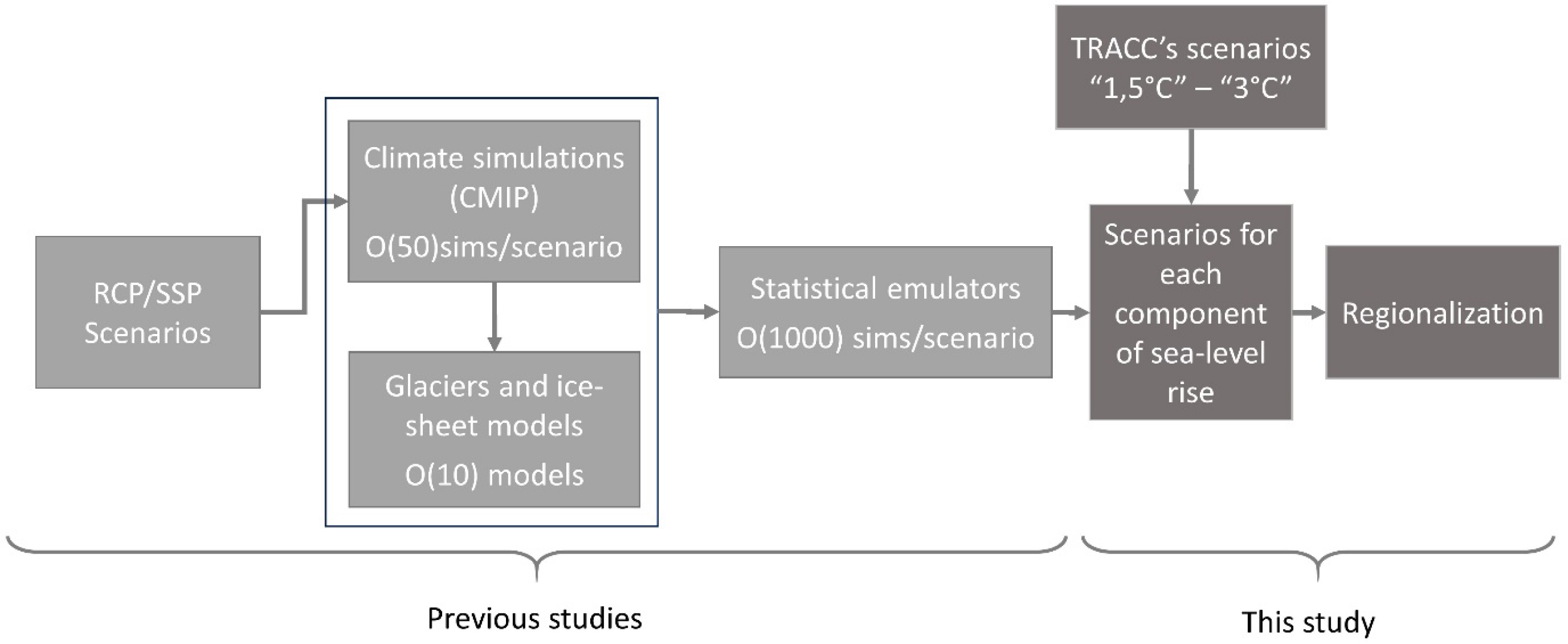
Approach used in this study to regionalize sea-level scenarios. “O(10)” models means “roughly 10” models.
2.2.1. Selection of simulations compliant with the French climate scenarios (TRACC)
Climate scenarios currently do not deliver results for a given level of global warming but follow assumptions on future greenhouse gas concentrations. Depending on the climate sensitivity of each climate model, the same concentration of greenhouse gas may result in different levels of global warmings. Hence, we proceeded by selecting climate model simulations or emulator realizations that have a global mean surface temperature trajectory consistent with those provided in Table 1, considering a margin of 0.3 °C around each temperature threshold. For the sterodynamic component of sea-level rise (i.e., ocean density and circulation changes corrected from the inverse barometric effect, which therefore includes the thermal expansion) (Gregory et al., 2019), we selected temperature trajectories compliant with the French climate scenarios. For climate-dependent mass components, an additional level of complexity results from the fact that glaciers and ice-sheet models must be forced with climate scenarios to obtain the resulting melting, which is computationally demanding. For example, the Greenland ice-sheet melting experiments of the Ice Sheet Model Intercomparison project for CMIP6 (ISMIP6) considers 14 ice-sheet models (with up to 2 or 3 different initial conditions), 2 greenhouse gas emission scenarios and 6 climate models. This is not sufficient to cover all potential climate scenarios of interest (Goelzer et al., 2020). To overcome this difficulty, emulators of ice melting models have been produced (Edwards et al., 2021). Emulators are statistical approximations of the response of glaciers or ice-sheet models trained over a limited set of computer experiments.
Figure 2 presents a selection of glacier model projections from the Emulandice emulator (ibid.), based on the French 3 °C climate scenario. Among the ∼3000 emulated simulations, about 250 reach 2 °C GWL in 2050 and 3 °C GWL in 2100. This selection of 250 emulated simulations gives a median glacier contribution to sea-level rise of 5.5 ± 1.0 cm in 2050 and 14.0 ± 2.5 cm in 2100 with respect to the reference period 1995–2014.
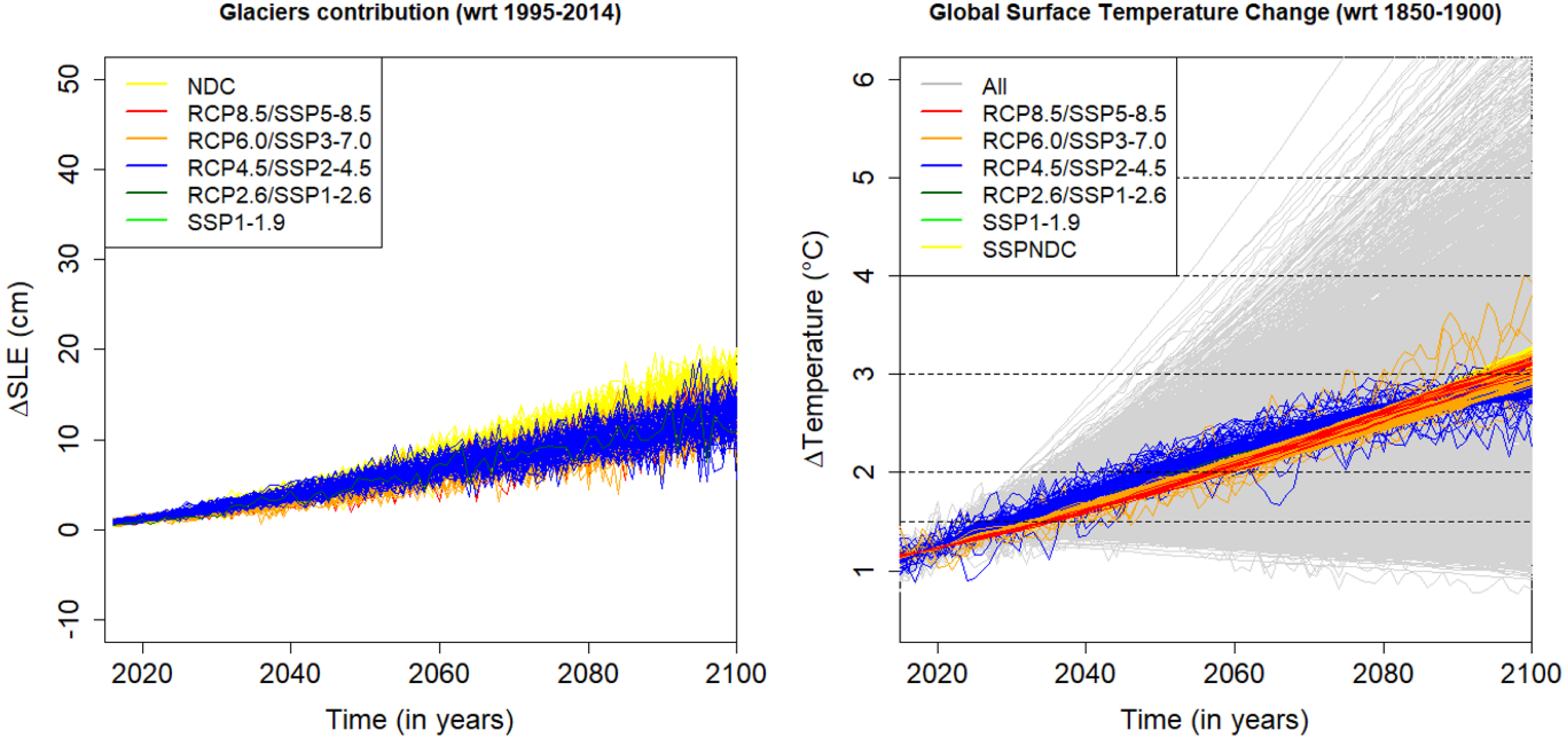
Subset of emulated glacier melting projections meeting the French climate projection assumptions. The right panel displays the projected temperature of emulated model projections meeting these assumptions. The left panel shows the resulting contribution of glaciers to sea-level rise to 2100. Note that simulations meeting the constraints are not limited to RCP4.5 or SSP2-4.5 scenarios, because some climate models have lower climate sensitivity and only reach 3 °C by 2100 despite higher greenhouse gas emissions.
2.2.2. Climate and emulated ice-melting projections and the case of Antarctica
The specific datasets used in this study are listed in Supplementary Material 1. Specifically, we use CMIP6 and the emulated sterodynamic component (Fox-Kemper et al., 2021), GlacierMIP simulations for glaciers (Marzeion et al., 2020), ISMIP6 emulated projections of Greenland ice-sheet melting (Edwards et al., 2021; Goelzer et al., 2020), projections of land water contributions from the AR6 (Fox-Kemper et al., 2021) and the GIA model results of Caron et al. (2018). For Antarctica, two datasets are used: emulators of ISMIP6 simulations (Seroussi et al., 2020) and LARMIP-2 simulations (Levermann et al., 2020). In addition, we use separately simulations considering the potential for an early onset of the Antarctica ice-sheet (De Conto et al., 2021). These simulations consider the possibility of an onset of Marine Ice-Cliffs Instability (MICI), a low-likelihood but high impact event that cannot be excluded according to the 6th assessment report of the IPCC (Arias et al., 2021).
2.2.3. Projections beyond 2100
Coastal risk prevention plans in France consider coastal impacts of sea-level rise over the coming 100 years, that is, 25 years beyond 2100. Yet many datasets used in this study are only available until 2100. Illustrative model simulations beyond 2100 start to be available in the literature, including for intermediate scenarios extending the SSP2-4.5 scenario (Turner et al., 2023). However, given that the policy need holds only until 2125, we use a quadratic extrapolation of Emulandice and LARMIP-2 simulations to deliver projections beyond 2100. This approach allows avoiding discontinuities in the trends around 2100. Note that the emulator uncertainties are also extrapolated to avoid a spurious reduction of the uncertainty envelop beyond 2100 (see Supplementary Material 2 for details). Notwithstanding, the confidence in the results decrease as the projections are extrapolated farther in the future. Hence, we limited these projections to 2120 and referred to SSP projections of the AR6 to deliver indicative values to 2150 (see Supplementary Material 3).
2.2.4. Regionalization and probabilistic aggregation
The methods to regionalize sea-level rise have been established about 10 years ago (Slangen, Katsman, et al., 2012; Slangen, Carson, et al., 2014; Kopp, Horton, et al., 2014) and taken over in the 5th and 6th assessment reports of the IPCC. The principle is to regionalize the impacts of each component first, then sum these components to deliver regional sea-level projections. The physical mechanisms to be considered are different for sterodynamic sea-level changes, for sea-level changes involving a mass component, and for relative sea-level changes due to vertical ground motions (Gregory et al., 2019):
- Sterodynamic sea-level changes representing the thermal expansion plus the ocean dynamic corrected from the inverse barometer effects are obtained from climate model simulations (i.e., CMIP6) with adjusted climate sensitivities as in the 6th Assessment Report of the IPCC.
- For mass components, that is, those related to glaciers and ice-sheet melting as well as land water, the principle is to consider the gravitational, rotational and deformational effects induced by the mass redistributions.
- Vertical ground motions include the glacial isostatic adjustment (GIA), the response of the solid Earth to the last deglaciation, for which models exist already (Caron et al., 2018), as well as other subsidence and uplift processes due to, e.g., groundwater pumping, seismic processes or variations of the water content in recent sedimentary layers (Nicholls, Lincke, et al., 2021).
In this work we follow this standard approach, using codes developed in R whose details have been presented in previous articles (Dayan et al., 2021; Le Cozannet, Thiéblemont, et al., 2019; Thiéblemont, Le Cozannet, Toimil, et al., 2019). The main change is that we use the static fingerprints used in AR6 to regionalize the mass components (Fox-Kemper et al., 2021; Kopp, G. G. Garner, et al., 2023), instead of those used in previous report (Church et al., 2013; Oppenheimer et al., 2019). As in previous work, we exclude local vertical ground motions (besides GIA), because they can be assessed in various ways, e.g., using tide gauge records as in the 6th Assessment Report of the IPCC, using Global navigation satellite system GNSS data, levelling data or satellite Interferometric synthetic aperture radar (Woppelmann and Marcos, 2016; Thiéblemont, Le Cozannet, Nicholls, et al., 2024). These processes are typically best understood and quantified locally where geologists can interpret the processes taking place in their region of interest, and more research is needed to be able to include them in regional projections without decreasing the confidence in these projections (Slangen, Palmer, et al., 2023).
We apply a Monte-Carlo approach to provide probabilistic projections. Specifically, we randomly select (sampling with replacement) simulations out of those available, for example one out of the 250 simulations available from Emulandice for the 3 °C scenario. Dependencies between components can have an impact to uncertainties (Le Bars, 2018), especially at the tail of the distribution (Le Cozannet, Thiéblemont, et al., 2019). Here we assume perfect dependence between the different glaciers regions and independence with other contributions. Since we select models reaching the same levels of global warming, this choice has limited impacts to the uncertainties of projections, especially within the 5–95th range of percentiles. For the Antarctic component of the TRACC scenarios without MICI, two datasets are available: LARMIP-2 and Emulandice. We extract the simulation that has the greatest deviation from the mean of the medians of the two datasets. This procedure leads to maximizing the uncertainties of the Antarctica ice-sheet component, which is in line with the high uncertainties surrounding Antarctica’s response to global warming. A total of 5000 random samples was carried out for each global warming level and time horizon.
3. Results
The sea-level scenarios corresponding to a stabilization of climate change at 1.5 °C GWL, and a warming leading to 2 °C GWL by 2050 and 3 °C GWL by 2100 are presented in Figures 3 and 4 bellow, respectively, as well as in Table 2. These scenarios are consistent with the assumptions of the French climate scenarios presented in Section 2.1 above.
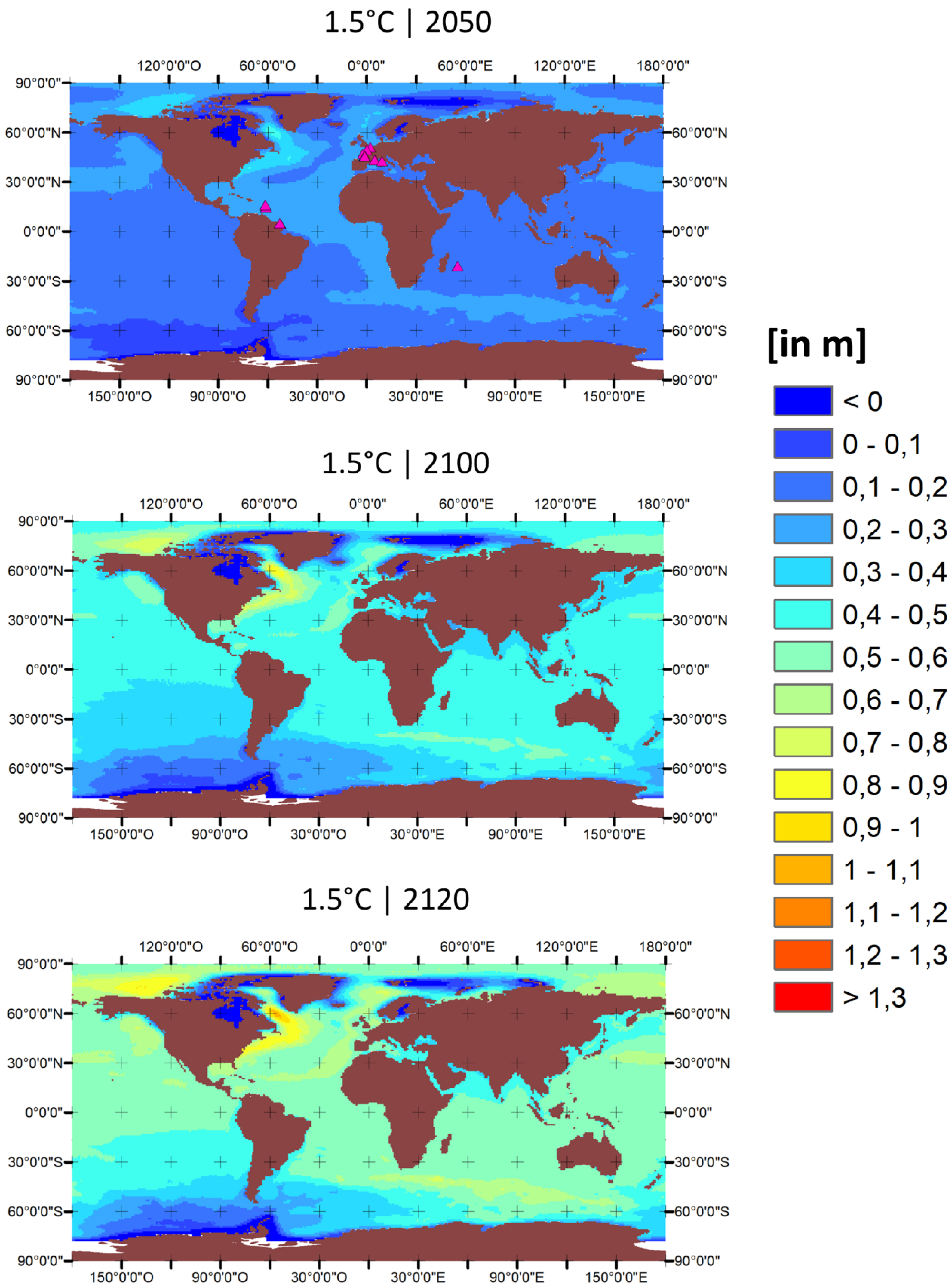
Median regional sea-level projections for a stabilization of climate at 1.5 °C GWL. Projections are given in meters with respect to the reference period 1995–2014. Pink triangles indicate the location of sites given in Supplementary Material 3. These projections follow the French climate scenario that is indicatively given to remind the climate mitigation objective of the Paris agreement (see Section 2.1).
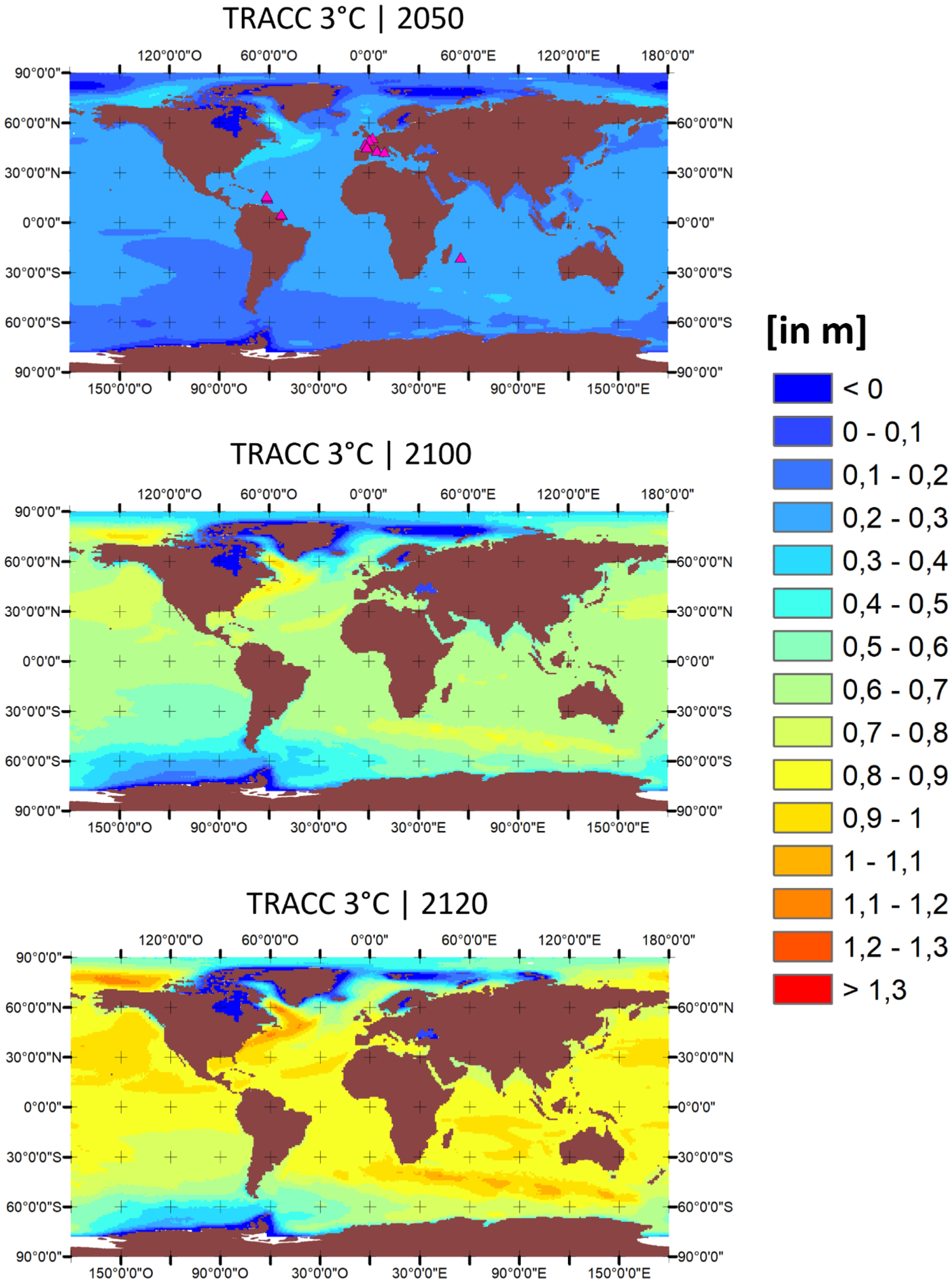
Median regional sea-level projections for climate change scenario reaching 3 °C GWL in 2100 as assumed in the French Climate Scenario (TRACC). Projections are given in meters with respect to the reference period 1995–2014. Pink triangles indicate the location of sites given in Supplementary Material 3.
Global mean sea-level rise projections, in cm with respect to the reference period 1994–2015 (50th [17th–83rd] percentiles)
Scenario and time horizon | 2050 | 2100 | 2120 |
---|---|---|---|
1.5 °C | 18 [15–22] | 41 [29–54] | 49 [33–68] |
3.0 °C | 21 [17–25] | 60 [48–77] | 80 [61–104] |
3.0° (with models including MICI) | 20 [17–22] | 63 [53–76] | 86 [71–107] |
3.1. Regional projections by 2050, 2100 and 2120
By 2050, sea-level at 1.5 °C or 2 °C GWL are projected to rise by approximately 20 to 30 cm above 1995–2014 levels in mainland France and inhabited overseas territories. Yet, in some other regions, sea-level projections lie outside this range. For example, off the eastern coast of the United States, sea levels are projected to rise above 30 cm due to the combination of sterodynamic effects (Couldrey et al., 2021) and background subsidence due to the glacial isostatic adjustment (Love et al., 2016). Sea levels are still projected to decrease in Scandinavia due to the GIA effects. The gravitational, rotational and deformational effects caused by the melting of ice in Antarctica, Greenland and in the arctic glaciers are already visible by 2050: our projections display a drop in sea-level changes visible close to the region of mass loss by that time horizon already. By 2050, the differences between the 1.5 °C and 2 °C GWL scenarios are small, because sea-level changes for the coming three decades are very much constrained by past greenhouse gas emissions (Hinkel et al., 2019).
In 2100, the disparity between the 1.5 °C and 3 °C GWL scenarios is more evident. Under the 1.5 °C GWL scenario, the median sea-level rise for metropolitan France and inhabited overseas territories is projected to lie in the range of 40–50 cm. In contrast, the median sea-level rise exceeds 60 cm for 3 °C GWL. Even by 2120, the 1.5 °C scenario indicates that the median sea-level rise would remain below 60 cm along most of the French coastlines.
3.2. Impacts of an early onset of ice-sheet collapse in Antarctica
As explained in Section 2.2.2, we explore the potential contribution of an early collapse of the Antarctica ice-sheet by replacing the contribution of Antarctica from Emulandice and LARMIP-2 (Edwards et al., 2021; Levermann et al., 2020) by a dataset implementing the MICI (De Conto et al., 2021), that is, following the same approach as in AR6 (Fox-Kemper et al., 2021). The 83rd percentiles of these simulations are presented and compared in Figure 5. We present the 83rd percentile of simulations to be consistent with the narrative of the “low-likelihood high impact” event presented in AR6.
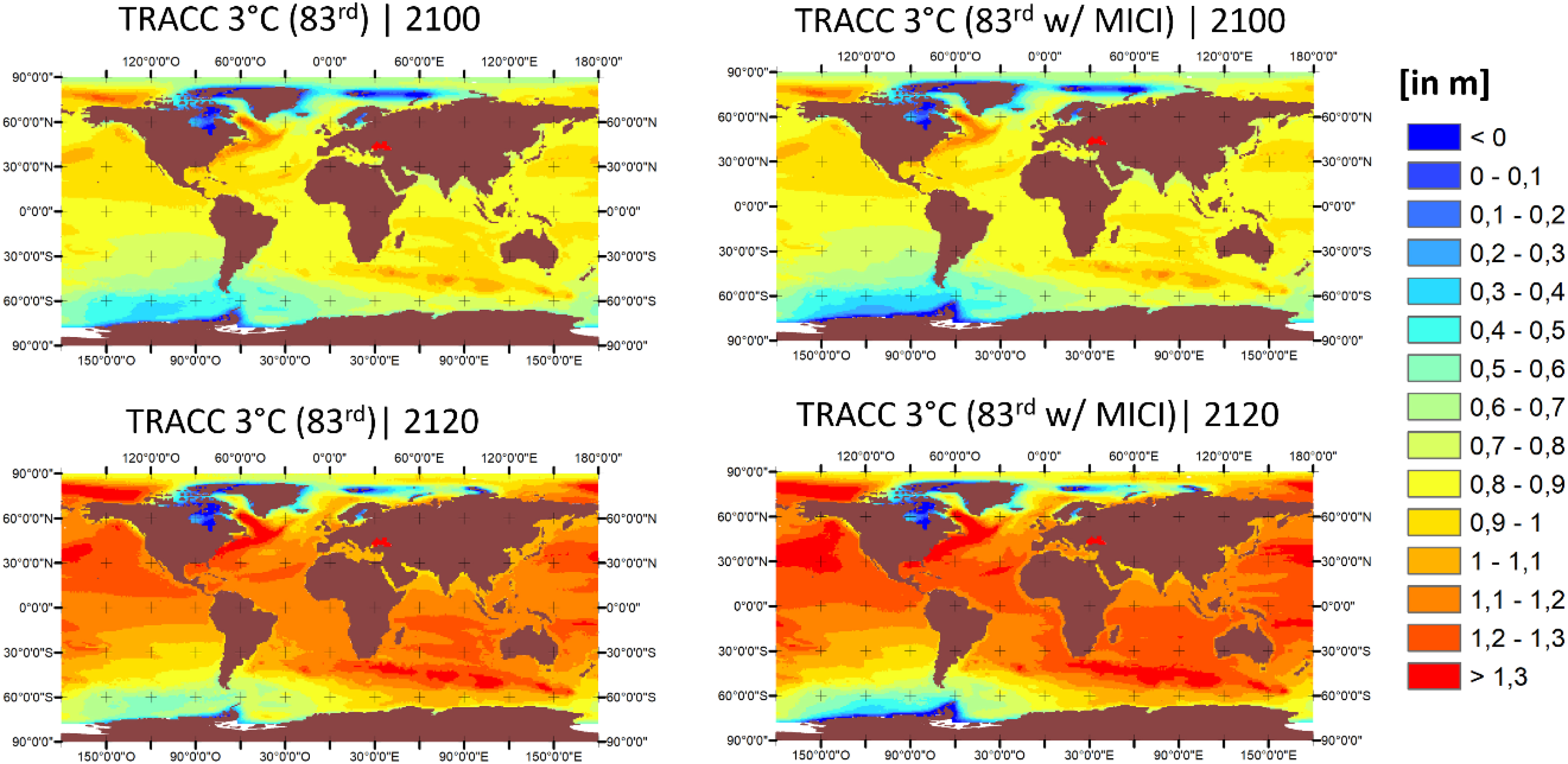
Regional sea-level projections in 2050, 2100 and 2120 (in meters) corresponding to the 83rd percentile of the 3 °C GWL level scenario with respect to 1995–2014 with MICI (left) and without MICI (right).
In 2100, sea-levels are projected to lie between 80 and 90 cm along most coastal areas of mainland France and inhabited overseas territories and regions (83rd percentile of projections). By 2120 the projections are 10 to 15cm higher in tropical overseas regions and territories than in mainland France due to the gravitational and rotational effects of ice-melting. They exceed 1.20 m in La Réunion island, where both the gravitational and rotational effects of ice melt are maximized. Importantly, the simulations with and without MICI are similar, reflecting that 3 °C GWL is too low to significantly activate the MICI in the simulations of De Conto et al. (2021) and that different statistical processing of uncertainties have been performed for the simulations with and without MICI (see Section 2.2.2).
3.3. Relation with the adaptation policy
In France, the primary adaptation policy that explicitly incorporates sea-level projections is the implementation of coastal risk prevention plans (Le Cozannet and Cazenave, 2024). These plans use numerical flood simulations to prescribe accommodation measures in urbanized areas and to limit further urbanization in the most exposed areas that have not been developed yet (DGPR, 2014). Since 2011, a sea-level scenario of 60 cm sea-level rise with respect to the end of the 20th century is considered and added in flood simulations representing potential flooding in 2100 (MEDDTL, 2011).
For national authorities, a first difficulty arises due to inconsistencies in the time horizons considered in the various articles of law, decree and technical guidance. The regulation on the coastal prevention risks (Article R562-11-5 of the Environmental Code, available https://www.legifrance.gouv.fr/codes/article_lc/LEGIARTI000038733755) requires considering coastal hazard changes in 100 years. However, the decree of 2011 gives a scenario of sea-level rise to 2100 (ibid.). Yet the two time horizons—2100 and in 100 years—have been confused in the technical guidelines of 2014 delivered to practitioners and coastal consultants in charge of implementing the regulation (DGPR, 2014). While this did not make a big difference in the early 2010s, considering a scenario in 2120 or 2125 now adds immediately about 20 cm to the national sea-level rise guidance. For national authorities, resolving these inconsistencies might be seen as a sensitive challenge due to the potential implications for land use development in the low lying coastal plains.
A second challenge for national authorities will be to decide how the new climate change scenario will be enforced in the area of coastal risk prevention. A most immediate outcome of the adoption of the TRACC as a reference scenario for coastal adaptation in France would be to update the uniform guidance of 60 cm of sea-level rise used within the coastal risk prevention plan policy. Importantly, we note that the decree that established this guidance in 2011 considered this 60 cm sea-level rise scenario as “pessimistic” (MEDDTL, 2011). Furthermore, the second national adaptation plan explicitly mentioned that its aim was to adapt to 2 °C GWL (MTES, 2018—see page 1). At that time, the 60 cm sea-level rise scenarios could still be justified by the fact that these 60 cm corresponded to the upper limit of the likely range of sea-level projections for the RCP2.6 scenario shown in the 5th Assessment report of the IPCC. This would now correspond to a 83rd percentile of probabilistic projections.
As sea-levels are projected to rise well above 60 cm sooner or later, a key question raised by the French coastal risk prevention plans is to determine when the sea-level guidance will be exceeded and the benefits of this particular policy would be lost. In fact, once the sea-level scenario will be exceeded, areas unaffected by development restrictions will start to be exposed to flooding. According to the sea-level scenarios shown above, there is approximately a 50–50 chance that the 60 cm sea-level guidance is exceeded between 2120 and 2150 in mainland France and overseas regions and territories at 1.5 °C GWL (see Supplementary Material 3). For the 3 °C GWL scenario, the probability to exceed the 60 cm sea-level guidance is above 50% in 2100. This gives little security margin, especially if we consider that global warming levels above 3 °C GWL cannot be excluded.
Based on this analysis above, we note that selecting the 83rd percentile of the 3 °C GWL scenario (without MICI) as a new guidance would preserve the same security margins that were originally anticipated when the national scenario was adopted in 2011 and when the second national adaptation plan was published in 2018. As shown in Section 3.2, the 83rd percentiles of the simulations with and without MICI are very similar at 3 °C GWL. If sea levels rise according to the median in the real world, having chosen a higher percentile as the reference scenario will provide one or two extra decades to prepare for sea levels that will be exceeded in any case. This decision belongs to the ministry in charge of Environment and has not been announced yet at the date of submission of this article.
One advantage of choosing a unique climate change scenario of 3 °C GWL could be to give a simple signal to the numerous actors who are still in the early stages of engaging into adaptation. However, for advanced users, higher scenarios (e.g., 5 °C GWL) can be more relevant if their time horizon and aversion to uncertainties justifies it (Hinkel et al., 2019).
4. Discussion
4.1. Limitations of this study
The sea-level projections developed within this study rely on state-of-the-art modeling of each component of sea-level rise and used, e.g., in AR6. These projections could be challenged in the future. Yet, it can be noticed that the likely range of sea-level projections has been remarkably consistent across assessments since 40 years (A. J. Garner et al., 2018). In contrast, high-end, or low-likelihood/high-impact sea-level projections have shown considerable variations (Piecuch and Das, 2022). Hence it cannot be ruled out that future projections of polar ice sheet melting are revised upward in the future.
The main limitations related to the regionalization are threefold: first, coastal processes at higher resolutions than 1° × 1° are not represented in our projections, although they can alter sterodynamic sea-level projections by about 20% on the continental shelf or in the Mediterranean (Chaigneau et al., 2024; Jevrejeva et al., 2024; Sannino et al., 2022). Second, the regionalization of ice mass losses could be improved a bit with a better characterization of the specific locations of melting areas in Greenland (Meyssignac et al., 2017). Lastly, vertical land motions other than GIA can also add a sizeable contribution to relative sea-level change. In mainland France, these processes are much less critical than in Asia, but they still exceed 1 mm/year over more than half of the low-lying coastal flood plains (Thiéblemont, Le Cozannet, Nicholls, et al., 2024). It can be noted that the national guidance of coastal risk prevention plans leaves the possibility to consider local knowledge on vertical land motions and revise the national sea level rise scenario upward. To our knowledge, this has never been done in a regulatory prevention plan. Based on the literature above, we estimate that the final impacts to the total mean relative sea-level projections in mainland France would be limited to 20 or 30 cm in the worst case. In overseas regions and territories, vertical land motions are less well characterized. Thus, we are not able to bound uncertainties of relative sea-level projections in these overseas regions and territories.
A final difficulty of these projections is their use for the coming decades for problems such as the adaptation to high-tide flooding events that have started to emerge due to sea-level rise (Sweet and Park, 2014; Thiéblemont, Le Cozannet, D’Anna, et al., 2023). Making sure that the projections can make sense locally and are calibrated accurately with observations will require further observations, reanalysis of historical sea levels and research.
4.2. Bridging the gap from sea-level scenarios to coastal adaptation: the need for a clear strategy
While the limitations listed above are important, they are clearly not the main barrier preventing coastal adaptation to advance in France. Understanding these barriers requires explaining the policy and regulatory context in which these scenarios are developed (Cabana et al., 2023).
Since June 2022, the Ministry in charge of environment repeatedly postponed the publication of the national adaptation plan (See Supplementary Material 4). During the preparatory work and consultations, from 2022 to 2024, the governmental communication generally focused on the levels of warming required for the national scenario supporting the plan rather than on the specific measures of the plan themselves. This is evidenced in the selection of articles shown in Supplementary Material 4 illustrating this statement. Very few objectives, political choices and financing mechanism supporting adaptation can be identified in these articles. Yet, the climate scenario itself was extensively discussed. When the national adaptation plan was finally published in March 2025, its publication was welcomed by the French Climate Council, which also raised concerns about its legal fragility and how the new national climate change scenario would be used and enforced (Haut Conseil pour le Climat, 2025).
We argue that the discussion around the choice of global warming scenarios is a technical one that is intended to support adaptation professional and requires accurate knowledge of their decision context. In the short term, a discussion around scenarios could stimulate technical measures such as infrastructure reinforcement or accommodation or adjustments in the regulation. Yet, we found no evidence that this discussion facilitated adaptation stakeholders in engaging in an informed process clearly highlighting the benefits and trade-offs involved in upcoming coastal adaptation decisions. We argues that scenarios support coastal adaptation in a useful way, but that they cannot replace a strategy with clear and realistic objectives and shared by all stakeholders.
There is a risk of locking-in coastal areas in current development patterns if no clear strategy toward sustainability is set out. This also increases the risks of social conflicts around coastal adaptation. For example the newspaper article dated 9th April 2023 in Supplementary Material 4 suggests that the stage is set for conflicts to emerge between social groups in favor of relocation and those supporting engineered protection. Such a conflict could emerge after the next major storm or while discussing any coastal adaptation decision in favor of any of these options. An additional risk is that by focusing on sea-level scenarios rather than on the policy choices involved in coastal adaptation (Perherin and Meur-Ferec, 2022), we miss the opportunity to take advantage of the inevitable land use changes induced by rising sea levels to create more resilient coastal systems, which would be ultimately beneficial for human communities and for economic activities and coastal ecosystems.
4.3. The national adaptation plan: an opportunity to progress toward coastal resilient development?
At timescales of centuries, sea levels are projected to rise by several meters (Fox-Kemper et al., 2021). In low-lying areas, responses to such large sea-level changes will either involve relocation or engineered protection (Le Cozannet, Nicholls, et al., 2023; Muccione et al., 2024). This is because in the majority of cases, accommodation and nature based solutions will lose effectiveness with larger and quicker sea-level changes (Bednar-Friedl et al., 2022). Both options change will involve large costs and stringent changes in land use, but they can be anticipated to minimize the negative impacts of current decisions for future generations (Le Cozannet, Nicholls, et al., 2023).
One obvious opportunity is to take advantage of the unavoidable investments in coastal adaptation to protect and restore coastal and estuarine ecosystems and improve their management, in order to enhance their structure and functioning and then their ability to cope with sea-level rise and other anthropogenic pressures. Figure 6 provides a selection of such actions, widely grouped under the umbrella term Nature Based Solution (NBS), and their co-benefits relevant to coastal environments that can be found in mainland France and overseas regions and territories. Table 3 shows that such management actions are already implemented in many coastal locations in Europe. A challenge in this area is to upscale local experiments in order to progress toward resilient coastal zones management (Bednar-Friedl et al., 2022).
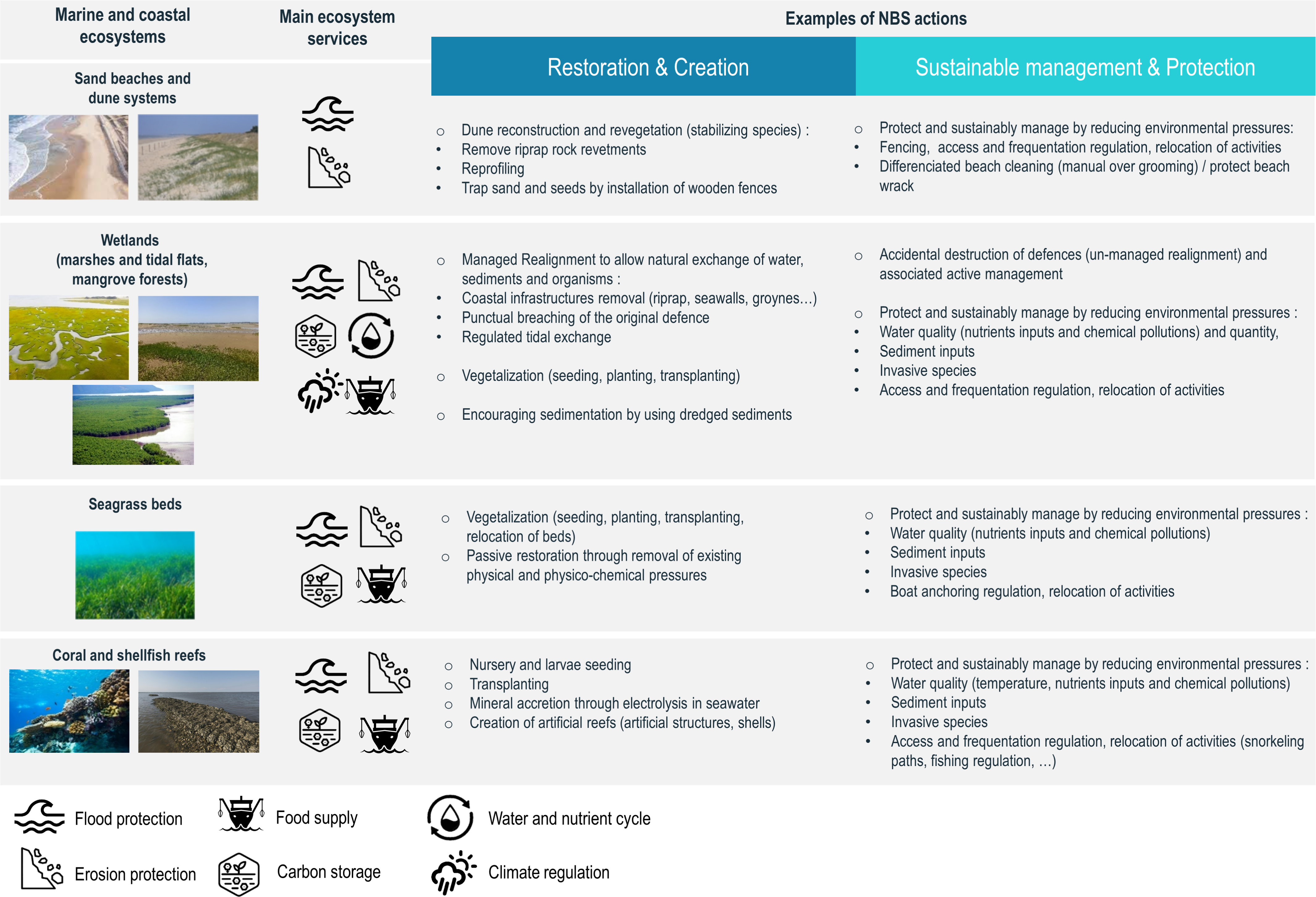
Examples of coastal Nature-Based Solutions (NBS) and their co-benefits for different types of coastal environments relevant to mainland France and overseas regions and territories. NBS refer to a range of established approaches (restoration, protection, ecosystem management) simultaneously providing societal and environmental co-benefits (Figure adapted from “Geochronique”, N°164, Société Géologique de France).
Examples of NBS already implemented across Europe and an insight of their associated level of transformative change
Project name and location | Ecosystem | Ecosystem services targeted | Co-benefits of the project | Drivers of decision | Type of actions | Current and projected effectiveness | References |
---|---|---|---|---|---|---|---|
Scheldt estuary: Hedwig-Prosper polder dike-retreat (Belgium) |
Saltmarhes and freshwater marshes | Flood risk reduction | Biodiversity Cultural (educational and recreational) |
Catastrophic floods and deaths | Flood control areas Managed realignment |
Consideration of ecosystem adaptation capacity until 2100 | https://climate-adapt.eea.europa.eu/fr/metadata/case-studies/a-transboundary-depoldered-area-for-flood-protection-and-nature-hedwige-and-prosper-polders https://www.vliz.be/imisdocs/publications/366209.pdf |
Scheldt estuary: Lippenbroek polder, Kruibeke polder (Belgium) |
Saltmarhes and freshwater marshes | Flood risk reduction | Biodiversity Cultural (educational and recreational) |
Catastrophic floods and deaths | Controlled reduced tide (temporary storages areas for overflow water) | Consideration of ecosystem adaptation capacity until 2100 | https://www.sigmaplan.be/en/projects/polders-kruibeke |
Humber estuary: Alkborough Flats Managed Realignment (United Kingdom) |
Saltmarhes and freshwater marshes | Flood risk reduction | Biodiversity: compensation site for habitat loss elsewhere in the estuary | Exposed human activities: major part of the Environment Agency’s flood risk management strategy on the estuary | Managed realignment | Reduction of effectiveness expected due to accretion at the site | https://www.therrc.co.uk/sites/default/files/projects/54_alkborough.pdf |
Hondsbossche and Pettemer Sea Dike (The Netherlands) |
Beach and dune systems | Flood risk reduction | Biodiversity Educational and recreational |
Decrease of the safety levels provided by the traditional engineering structure | Removal of dikes and creation of a “soft barrier” as a new beach dune system | Currently effective, the natural dune growth is predicted to keep up with sea level rise up to 2050 | https://www.ecoshape.org/en/cases/sand-nourishment-hondsbossche-dunes-nl/ |
Urban hybrid dunes in Barcelona (Spain) |
Beach and dune systems | Erosion risk reduction | Biodiversity Awareness |
Destruction caused by urbanization | Beach nourishment and planting dunes with pioneer grass (Ammophila sp.) | Not measured | https://oppla.eu/casestudy/17283 |
Mud motor Port of Harlingen–Koehoal (The Netherlands) |
Saltmarshes | Biodiversity: restoration, conservation | Erosion reduction | Loss of saltmarshes in the Wadden Sea | Dredging and disposing fine sediments to stimulate the growth of salt marshes | Currently effective: high sediment transport rates | https://www.ecoshape.org/en/cases/re-use-of-dredged-sediment-mud-motor-in-port-of-harlingen-koehoal-nl/ |
Coastal erosion control in Paphos (Cyprus) |
Beach and dune systems | Regulating Erosion risk reduction |
Water quality Recreation |
Local threat (resort) | Removal of groynes | Currently effective; observed accretion and a newly formed beach | https://discomap.eea.europa.eu/map/Data/Milieu/OURCOAST_172_CY/OURCOAST_172_CY_Case_CoastalErosion_OverstructuredBeach.pdf |
Mondego estuary (Portugal) |
Saltmarshes | Water quality | Erosion reduction | Increased eutrophication and ecological losses | Reduction nutrient inputs from the catchment Improving water circulation through connexion of formerly embanked channels |
Currently effective; no harmful bloom recorded, healthy seagrass beds | (Dolbeth et al., 2010) (Lepage et al., 2022) |
Mortagne polder in the Gironde estuary (France) |
Saltmarshes | Biodiversity | Water quality and nutrient cycling | Accidental depoldering after Martin storm (1999) | Willingness to let the system evolve with no other intervention | Currently habitats with important ecological functions | (Lepage et al., 2022) Life adapto project |
Adaptive restoration of the former saltworks in Camargue (Rhône delta) (France) |
Saltmarshes | Biodiversity | Flood protection Erosion reduction Tourism |
Delta vulnerable to flooding and ecological loss (intense salt production and polders) | Dyke removal and restoration of gravitational flows | Currently development of beaches but no quantitative data on erosion effectiveness | https://climate-adapt.eea.europa.eu/metadata/case-studies/adaptive-restoration-of-the-former-saltworks-in-camargue-southern-france |
Nature based solutions are often associated with projects involving some planned relocation. In fact, relocations provide space that is presently lacking for coastal sediments and ecosystems in many anthropogenized coastal zones. However, nature based solutions can also be combined with coastal engineering projects, a practice referred to as “hybrid nature-based solutions” (Sowińska-Świerkosz and García, 2022).
Typical barriers to the implementation of coastal nature based solutions include a perceived lack of effectiveness, lack of ecological knowledge, lack of comparative economic scenarios of benefits, insufficient funding and the inability of current governance to manage such complex projects and address the related social challenges. Research projects supporting ongoing experimentations and implementations can help better assessing the effectiveness of different options and move from a reactive adaptation to a strategic planning approach anticipating the meters of sea-level rise that are projected at multi-centennial timescales while also addressing other challenges of resilient development (Le Cozannet, Nicholls, et al., 2023; Lepage et al., 2022; Moraes et al., 2022; Philippenko and Le Cozannet, 2023; Schibalski et al., 2022). Examples shown in Table 3 can inspire new projects. For example, the Sigma plan conducted in Belgium is known already by many French practitioners, but it could also inspire new adaptation actions at least in the Seine, Loire, Gironde estuaries and Rhône delta. The Sigma plan implemented a number of different adaptation options along the Scheldt estuary, including protection works, managed realignment, flood control area, temporary storage area for overflow areas (aka control reduced tide areas) while allowing for large areas of tidal wetlands and their associated ecosystem services. Such an implementation ultimately creates more space for hydro-sedimentary processes and ecosystems in the estuary while offering sufficient protection at least for a few decades (Boerema and Meire, 2017; Jacobs et al., 2018; Lepage et al., 2022). This may avoid either developing costly estuarine barriers with potentially large adverse impacts to coastal ecosystem (Orton et al., 2023) or large human and economic losses due to storm surges if sea-level rise adaptation is not sufficiently anticipated.
While there is an increasing number of such actions, including in France with the adapto project of the Coastal Conservation Agency (Conservatoire du Littoral) and the nationally-defined coastal adaptation strategy (Le Cozannet and Cazenave, 2024), the implementation of nature based solutions has been slower in marine and coastal and estuarine ecosystems than in other ecosystems so far (O’Leary et al., 2023). The forthcoming regulatory context in Europe offers opportunities to accelerate in this area and implement transformative actions that are required for scaling up coastal nature based solutions. The EU Nature Restoration Law that entered in force on August 18th 2024 has a long term objective to restore >90% of degraded ecosystems by 2050, with an immediate target in 2030 of restoring at least 30% of them. A success in this area will require specific attention to loosely defined objectives, such as those referring only to surface areas and not specific levels of quality ecosystem functioning. It will also require research to learn as these solutions are implemented (O’Leary et al., 2023).
Overall, we argue that an essential aspect of a successful coastal adaptation should be on enabling system-wide actions to reduce direct and indirect drivers of biodiversity loss and environmental degradation and restore healthy ecosystems for the benefits of all. Such a success can obviously neither be guaranteed by the publication of a sea-level scenarios such as those provided within this study, nor by any adaptation plan avoiding any substantial discussion about the strategic objectives of adaptation. On the contrary, the success of coastal adaptation will require clear objectives, a transparent debate on the opportunities of coastal adaptation and political will.
5. Conclusions
The decision on the sea-level guidance to be considered after the 3rd adaptation plan belongs to the Ministry in charge of Environment. However, the future of the 3rd adaptation plan is uncertain due to political instability in France marked by the absence of a societal consensus on climate change responses, despite their being assessed in scientific reports such as those of the IPCC. More problematically, the public debate from 2022 to December 2024 has often focused on the climate change scenarios rather than the adaptation measures themselves. The consultation of the national adaptation plan in December 2024 created an opportunity to discuss coastal adaptation measures and strategies, but then the issue of the legal fragility of the climate scenario emerged. Obviously, defining a sea-level scenario is not sufficient to decide whether, e.g., relocation or protection is more appropriate. Previous adaptation plans suggest that such political decisions could be left to regional authorities in France, but it is unsure that their current governance and finance is sufficiently robust to plan efficiently for relocation plans or infrastructures that cost billions of Euros.
As stated by the national court of auditors in its annual report 2024 (Cour des Comptes, 2024), there is a danger that a narrow vision of adaptation limited to technical choices and regulation application eludes any strategic ambition for coastal development. In the worst case, this can result in a lock-in in unsustainable practices and large damage and human losses during coastal disasters. By avoiding a clear debate on coastal adaptation, France may be missing the opportunity to take advantage of the upcoming investments in adaptation to create more resilient coastal and estuarine ecosystems and generally more resilient coasts.
Declaration of interests
The authors declare no specific conflict of interest that would result in any benefit from the publication of this research. The first author initially contributed to a study funded by the ministry in charge of Environment linked to the topic of this manuscript, withdrew from this project to resolve conflicts of interests due to him also being member of the French Climate Change Committee in charge of evaluating climate policies in France. Hence, this work is published independently of any political or administrative influence, under the provisions of the European contracts that funded this research and in accordance with the Article L411-3 of the French Research Code.
Acknowledgements
GLC, JR, and RT are supported by the CoCliCo, PROTECT and SeaClim projects. These projects received funding from the European Union’s Horizon 2020 research and innovation program under grants agreements No 869304, 101003598 and 101180125; PROTECT contribution number 85. GLC and JR are also supported by the French National Research Agency under FUTURISKS (ANR-22-POCE-0002) project. CC was supported by the Adapto project. We thank Jean Jouzel and Etienne Ghys for organizing the workshop at the Academy of Sciences in March 2024 and this special issue. We used artificial intelligence to correct grammatical errors in the manuscript.
Supplementary data
Supporting information for this article is available on the journals website under https://doi.org/10.5802/crgeos.290 or from the author.
Vous devez vous connecter pour continuer.
S'authentifier