1 Introduction: weathering and erosion in the Amazon Basin
With an overall surface of 6.1 × 106 km2, the Amazon Basin plays a major role in the environmental evolution of the Earth due to the importance of the evergreen forest, the broad extent of low elevation lands and flooded areas, and the great diversity of soil and water systems (Radam Brasil, 1972–1978). World-scale fluxes of water (209,000 m3.sec−1) and suspended-solid matter (13.5 t.sec−1) are exported to the ocean and result from rainfalls, physical erosion of Andean soils and the reworking of alluvial terraces (Gibbs, 1977; Irion, 1991; Putzer, 1984; Richey et al., 1986). Losses of matter also come from chemical erosion of laterites (Gaillardet et al., 1997; Lucas et al., 1987, 1996), which are characteristic soil formations of tropical regions (Tardy, 1993; Tardy and Roquin, 1998). In this regard, the intense leaching of alkali and alkaline-earth elements and silica leads to a residual accumulation of Fe and Al in the freely drained parts of lateritic landscapes and, as pointed out in this paper, to their remobilization under waterlogged, reducing and acidic conditions. In contrast with western Africa, iron accumulation in the Amazon Basin leads to limited formation of iron pans (ferricretes or cuirasses) due to the low elevation of the lands. As a result, most laterites of the Basin consist of loose soil and saprolitic materials.
The development of lateritic soils on various sediments and rocks of the Amazon Basin (Fig. 1a) and their ultimate transformation into waterlogged, clay-depleted and organic-rich podzolic soils (Fig. 1b) have recently been replaced in the framework of major Cenozoic events (Fig. 1c) that have affected this part of the South American plate (Campbell et al., 2006; Do Nascimento et al., 2004; Fritsch et al., 2007). Alternating lateritization and erosion cycles first occurred during a period where the drainage system of the Amazon Basin discharged sediments into a Pacific embayment and built up continental deposits of Paleozoic, Mesozoic and Cenozoic ages (Fig. 1a). This is consistent with paleomagnetic data that attributed average ages of 60, 50 and 40 My to the lateritization cycles from Guyana paleo-surfaces (Théveniaut and Freyssinet, 2002). During the formation of the Andean cordillera, deeply weathered formations, developed as a result of intense weathering of the Guyana and Brazilian shields under warm and hot climates, were left in situ or transported during the Quechua I and II major orogenic events (Fig. 1c). During Quechua I, the erosion of deeply weathered products of the shields built up the world largest Cenozoic continental deposit, known in Brazil as the Solimões formation (Sampaio and Northfleet, 1973). With the ultimate orogenic events of Quechua II in the mid-Cenozoic and the definitive uplift of the Andes, deeply weathered, intensively faulted laterites (Franzinelli and Piuci, 1988; Fritsch et al., 2002; Iriondo and Suguiro, 1981) were partly washed out by erosion to form the Pan-Amazonian Ucayali Peneplain (Campbell et al., 2006). Ultimately, less weathered and thinner lateritic soils (less than 2 m deep) formed on the Late Tertiary sediments of the Iça formation, which likely originates from the erosion of the Andes (Fritsch et al., 2007; Sombroek and Camargo, 1983). Sediments of the Iça formation (Fig. 1a) accumulated in a huge lacustrine area of the upper Amazon Basin, following the closure of the gates to the Pacific ocean, the west to east switch of the Amazon drainage system, and the birth of the modern Amazon River network, i.e. ca 2.5 My ago (Campbell et al., 2006). River incisions into the Amazon lowlands during the Quaternary have formed the low elevation plateaus we know today and built up the alluvial terraces. Weathering on the plateaus has either formed old and deep laterites on various geological formations of the Ucayali Peneplain or younger and thinner ones on the Iça formation and developed waterlogged, clay-depleted and podzolic soils in the poorly drained areas of these plateaus (Do Nascimento et al., 2004; Fritsch et al., 2002, 2007; Klinge, 1965).

Broad scale (a) geological and (b) soil maps of the Brazilian Amazon Basin (reduced and simplified from Radam Brazil maps at 1:2,500,000) showing the location of the investigation sites (from I to IV) and (c) major events of the Cenozoic (adapted from Campbell et al., 2006).
Cartes régionales (a) des formations géologiques et (b) des sols du Bassin amazonien brésilien (réduites et simplifiées, d’après les cartes Radam Brazil au 1:2 500 000) montrant les quatre sites d’étude (de I à IV) et (c) principaux événements du Cénozoïque (adapté d’après Campbell et al., 2006).
This article summarizes the molecular and mineralogical-scale mechanisms and the geochemical trends governing the formation of laterites and their ultimate transformation into hydromorphic or podzolic soils. Results, presented and discussed in detail in previous articles, are synthesized here to suggest an integrated model of soil formation and evolution in humid tropical regions. Because soil forming and soil change processes (Fanning and Fanning, 1989) act under contrasted hydrological regimes of the low elevation plateaus of the upper Amazon Basin (i.e. vadose vs. saturated zones), they have a driving control in tropical regions on major geochemical cycles (mainly carbon and metal cations, such as Fe and Al), and thus on climate.
2 Vertical development of laterites
In the Manaus region, thick lateritic soils (up to 25 m deep), developed over Cretaceous sediments of the Alter do Chão formation (I in Fig. 1a,b), belong to the deeply faulted and incised landscapes of the Ucayali Peneplain (Campbell et al., 2006; Fritsch et al., 2002; Lucas et al., 1996). Laterites contain kaolinite, hematite, goethite and gibbsite as secondary minerals. Different populations of the dominant kaolinite, but also of the less abundant Fe-oxides were identified in the investigated profiles. Their proportion changes gradually from the bottom to the top of these profiles indicating therefore different steps in weathering. Such weathering, assigned here to a long-term lateritization process, led to the in situ formation of poorly differentiated and superimposed soil horizons differing from each other in gradual changes of texture and colour.
Changes in the kaolinite structural properties along lateritic profiles have been quantitatively investigated by Fourier-transform infrared spectroscopy (FTIR) and Electron Paramagnetic Resonance (EPR) (Balan et al., 2005, 2007; Lucas et al., 1996). Both techniques consistently show the presence of two types of kaolinite in various proportions. The progressive decrease of kaolinite order from the bottom to the top of the lateritic profiles results from the gradual replacement of an old population of well-ordered kaolinite, inherited from the sedimentary kaolin, by a more recent generation of poorly ordered soil kaolinite with a high proportion of dickite-like stacking faults (Fig. 2a,c) (Balan et al., 1999, 2000, 2007). Much progress has been made using information on crystal chemistry and structural properties of Fe-oxides provided by optical and Mössbauer spectroscopy (Fig. 2b,c) and quantitative XRD (Fritsch et al., 2005; Malengreau et al., 1994). All three methods reveal similar and complementary trends along the lateritic profiles, corresponding to an upward decreasing proportion and size of hematite and increasing content of structural Al in finely divided Fe-oxides, predominantly goethite. As for kaolinites, cyclic dissolution and precipitation reactions lead to the progressive replacement of older populations of weakly Al-substituted hematite and goethite by a younger population of aluminous goethite.
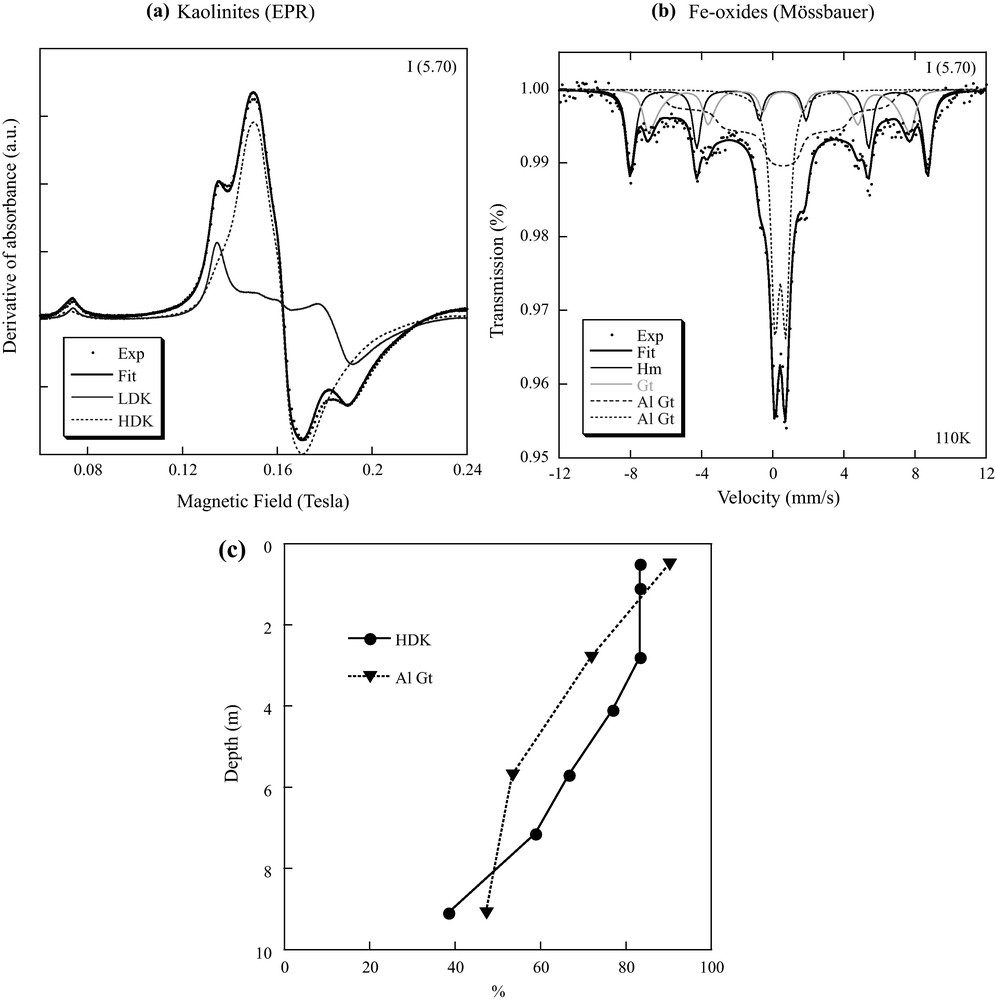
Spectroscopic analysis of lateritic materials: (a) evidence from EPR spectra of low- (LDK) and high-defect (HDK) kaolinite; (b) contribution of hematite (Hm), goethite (Gt) and aluminous goethite (Al Gt) to Mössbauer spectra; (c) evolution of the proportion of high-defect kaolinite and aluminous goethite in the pool of kaolinites and Fe-oxides, respectively, as a function of depth in a lateritic profile of the Manaus region (adapted from Balan et al., 2007 and Fritsch et al., 2005).
Analyse spectroscopique de matériaux latéritiques : (a) présence dans les spectres RPE de deux composantes, kaolinite ordonnée (LDK) et désordonnée (HDK) ; (b) contribution de l’hématite (Hm), la goethite (Gt) et la goethite alumineuse (Al Gt) dans les spectres Mössbauer ; (c) évolution de la proportion de kaolinite désordonnée dans le pool des kaolinites et de goethite alumineuse dans celui des oxydes de Fe, en fonction de la profondeur dans un profil latéritique de la région de Manaus (adapté d’après Balan et al., 2007 et Fritsch et al., 2005). Masquer
Analyse spectroscopique de matériaux latéritiques : (a) présence dans les spectres RPE de deux composantes, kaolinite ordonnée (LDK) et désordonnée (HDK) ; (b) contribution de l’hématite (Hm), la goethite (Gt) et la goethite alumineuse (Al Gt) dans les spectres Mössbauer ; (c) évolution ... Lire la suite
Kaolinite replacement and decreasing size of kaolinite particles mainly occur at depth at the transition between the loamy sediments and the upper clayey soil materials, whereas Fe-oxides replacement prevails in the overlying yellowish and clay-depleted topsoil (Fig. 2c). This two-step mineralogical and textural evolution is consistent with the differentiation of two major geochemical trends from the bottom to the top of the lateritic profiles (Fig. 3). The first geochemical trend at depth leads to significant loss of Si but is conservative for Fe and Al (1 in Fig. 3b). At the transition between sediments and soils, this first trend results from quartz dissolution (Lucas et al., 1993) and cyclic dissolution and precipitation of secondary minerals, which preserve the Fe and Al supply but decrease with time the average size and crystalline order of kaolinites in soils. Crystal chemistry confirms the weak mobility of iron during lateritization, as the content of structural iron in kaolinites does not change significantly, from the ordered to the overlying disordered kaolinites. The second geochemical trend, closely linked to clay depletion and upward yellowing, is still conservative for Fe but associated with Al losses (2 in Fig. 3b). It is also linked to Al enrichment in Fe-oxides and the appearance of gibbsite (Fig. 3a). Accordingly, weathering conditions in the topsoil are less favourable to the crystallization of kaolinite than to the formation of aluminous goethite and ultimately of gibbsite, when the entire pool of Fe-oxides has been replenished with Al (Fritsch et al., 2005). As kaolinite dissolution prevails over crystal growth, the lateritic topsoil progressively loses with time its clay supply and become sandier (Fig. 3a).
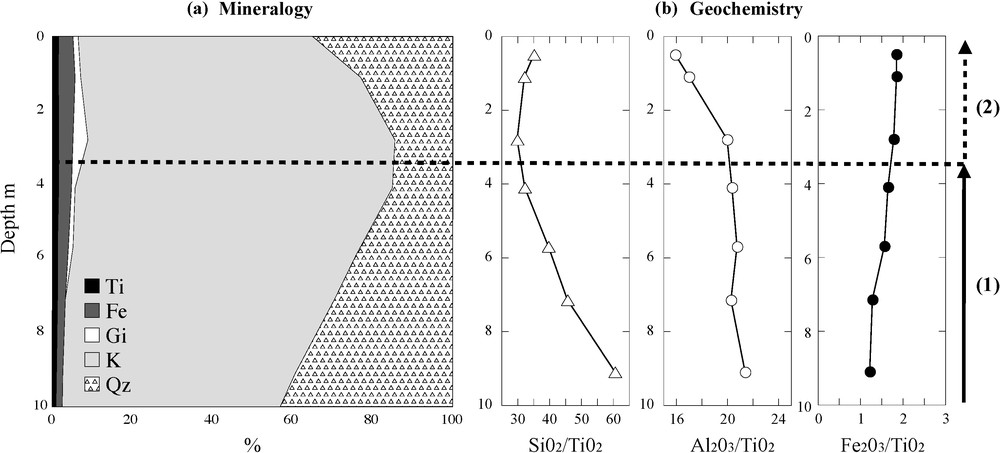
(a) Mineralogical (Qz: Quartz, K: Kaolinite, Gi: Gibbsite, Fe and Ti: Fe and Ti oxides) and (b) geochemical variations as a function of depth in laterites of the Manaus region. (1) Ferrallitisation, (2) Allitisation (associated with clay depletion and soil yellowing) (adapted from Fritsch et al., 2005).
Variations (a) minéralogiques (Qz : Quartz, K : Kaolinite, Gi : Gibbsite, Fe et Ti : Fe et Ti oxydes) et (b) géochimiques en fonction de la profondeur, dans les latérites de la région de Manaus. (1) Ferrallitisation, (2) Allitisation (associée avec une perte en argile et un jaunissement du sol) (adapté d’après Fritsch et al., 2005).
The apparent age of kaolinites from the lateritic profiles of the Manaus region ranges from 60 to 25 My for kaolinites of the Alter do Chão formation and 10 to 5 My for those of the overlying lateritic soils according to the concentration of radiation-induced defects (Balan et al., 2005). This change of apparent age in soils is thus consistent with the rejuvenation of the soils following the dissolution/crystallization reactions that decrease the size and crystalline order of kaolinite. The persistence in the uppermost topsoil of up to 20% of ordered kaolinite (Fig. 2c) with bulk samples showing an apparent age greater than 5 My results primarily from the physical denudation of the lands following the major faulting events of Quechua II, between 15 and 9.5 My (Fig. 1c). This persistence also indicates that no “re-equilibration” occurred for kaolinite after the faulting events, which suggests either that their rate of transformation is slow or that the present-day weathering conditions in the topsoil are no longer favourable for the formation of poorly ordered kaolinite.
3 Vertical and lateral development of hydromorphic and podzolic formations
More abrupt and contrasted morphological changes can be observed in lateritic landscapes of the Amazon Basin, more specifically in the waterlogged areas of the low elevation plateaus. Such changes commonly imply a greater accumulation of organic matter likely due to restricted bio-mineralization reactions in waterlogged soils and a net predominance of dissolution reactions over precipitation ones, resulting therefore in a major transfer of organic carbon and associated metals (mainly Fe and Al) in groundwater and river systems. In lateritic landscapes, these new features belong either to the hydromorphic or podzolic formations, which can be assigned to Gleysols or Podzols, respectively, when they reach the soil surface. This dichotomy in the end products of such weathering evolution suggests two geochemical pathways for major morphological changes in lateritic landscapes of the humid tropical regions.
3.1 Hydromorphic formations
The first geochemical pathway corresponds to the development of waterlogged and reducing (aquic) conditions in clayey topsoils as well as at greater depth in loamy saprolites. Reducing conditions result in selective dissolution of Fe-oxides, which is controlled by grain size and crystal impurities (mostly Al substitution) (Bryant and Macedo, 1990; Jeanroy et al., 1991; Schwertmann, 1984; Torrent et al., 1987). They lead to the formation of faint yellowish and bleached areas that are darkened by organic matter in the topsoil. These yellowish and bleached areas may be spatially linked to intensively colored and cemented materials, such as thin iron pans or plinthites.
Hydromorphic formations were investigated in the thick, faulted and eroded lateritic plateaus inherited from the Ucayali Peneplain for the Manaus region (I in Fig. 1a,b) (Fritsch et al., 2002) and in the thin lateritic soils developed on the Iça formation for the Humaita region (II in Fig. 1a,b) (Fritsch et al., 2007). In the Manaus region, Fe-mobility affects separately the thick lateritic soils that locally display bleached areas over thin iron pans and at greater depth the sediments, which are partly or totally bleached. Bleached areas over thin iron pans result from the downward accumulation of Fe in local perched waters, whereas bleached sediments at greater depth illustrate the export of Fe to the river systems via a regional groundwater aquifer. In the plateaus of the Humaita region, shallow aquifer and sediments explain the upward accumulation of Fe, with the formation of plinthites in the uppermost sediments, just below the thin clayey and reddish lateritic topsoil. These striking patterns, referred in Brazil to groundwater laterites, are widely extended on the Iça formation (Fig. 1a,b) (Sombroek and Camargo, 1983). In the depression and channel network of the plateaus, runoff and water pounding have enhanced the export of Fe in the topsoil and sediments and formed bleached areas that exhibit double tongue-like transition with their better-drained upslope lateritic counterparts (Fritsch et al., 2007).
3.2 Podzolic formations
The second geochemical pathway corresponds to the development of waterlogged, reducing and acidic conditions in clay-depleted laterites, which cap at depth (predominantly in saprolites) more regional hydromorphic formations (Bravard and Righi, 1989, 1990; Do Nascimento et al., 2004; Dubroeucq and Volkoff, 1998; Lucas et al., 1987). Accordingly, podzolization develops as a secondary process in topsoils affected by a strong clay impoverishment (ISSS Working Group RB, 1998), which develops interconnected voids between adjoining quartz grains (Fritsch et al., 1989) that in turn enable the downward migration of organic matter (Bardy et al., 2008; Bravard and Righi, 1990; Do Nascimento et al., 2004; Fritsch et al., 2009). Podzolization then marks an ultimate stage of chemical erosion of laterites that turn bleached and sandy. It is mostly related to the downward accumulation of humus acids, the weathering of the remaining clay minerals and the formation of organo-metallic complexes (Do Nascimento et al., 2004; Lundström et al., 2000; van Hees and Lundström, 2000).
Podzolic formations with their upslope lateritic counterparts were investigated at two locations, on the low elevation plateaus of the Negro River watershed (northern upper Amazon Basin). Downstream at the Jau site (III in Fig. 1a,b), the development of waterlogged podzols on the plateaus is restricted to depressions of various sizes and shapes, which are drained by small brooks and are not directly connected to the Jau river, a tributary of the Negro River (Do Nascimento et al., 2004). Upstream at the Curicuriari site (IV in Fig. 1a,b), podzols are, in contrast, widely spread in huge peneplains (Dubroeucq and Volkoff, 1998), incised and eroded by the Curicuriari river and its tributaries, which feed the river corridors with white sand bars.
Two major steps govern podzol development (Do Nascimento et al., 2004). The first step corresponds to the downward accumulation of humus acids into loamy sand laterites and the formation of a first generation of organo-metallic complexes in Bhs horizons of weakly differentiated podzols, also known as Humus Podzols (Thompson, 1992). The second step is associated with: (i) the appearance of bleached sands (E horizon), just below the eluviated and organic-rich topsoil (white sands with fresh organic residues); and (ii) the accumulation at greater depth of a second generation of organo-metallic complexes in superimposed Bh and Bs (or BCs) horizons of well-differentiated podzols (ISSS Working Group RB, 1998). Pore clogging by organo-metallic complexes reduces the soil permeability at the bottom of these podzols, which in return enables the settling of a perched water table during the rainfall season. Textural contrasts in lateritic profiles (for instance between the clay-depleted soil and the underlying loamy saprolite or sediment) can stop the vertical development of podzols, then initiating their lateral expansion in the landscapes (Do Nascimento et al., 2004; Sommer et al., 2001). Podzols expand upslope into their lateritic counterparts according to the dynamics of the perched water table (Do Nascimento et al., 2004; Dubroeucq and Volkoff, 1998; Lucas et al., 1987; Montes et al., 2007). They expand quicker near the surface and at greater depth into the uppermost hydromorphic zone of the upslope laterites revealing therefore a hydrologic connection between the perched water of podzols (black water) and the deep groundwater of laterites (clear water) (Do Nascimento et al., 2008).
Organic matter exhibits contrasted molecular-scale structures in podzols according to optical microscopy, Fourier-transform infrared (FTIR) spectroscopy and cross polarization/magic angle (CP/MAS) 13C nuclear magnetic resonance (NMR) (Bardy et al., 2008). Schematically, it consists of: (i) fresh organic residues in white sands with major aliphatic contributions in topsoil AE horizons; (ii) black organic colloids of high aromacity, as pellets or infills between quartz sands in Bh horizons; and (iii) dark brown and fine-grained organic coatings of low aromacity with abundant oxygen-containing functional groups (mostly carboxylic) in the less permeable Bhs and Bs (or BCs) podzolic horizons. These contrasted molecular-scale structures suggest that waterlogging favours the formation of humus acids from fresh organic residues and their fractionation during the recession of the perched water in texture-contrasted soils (Fritsch et al., 2009). Whereas the coarse fresh organic residues mostly remain in the topsoil, the black organic colloids, accumulated at the bottom of the bleached sands, build up the Bh horizons, and the much finer dissolved organic compounds (DOC) impregnate upslope and at greater depth the Bhs and Bs (or BCs) horizons at the periphery of the podzolic formations.
Metals (Al and Fe) bound to organic matter were quantitatively identified by chemical analyses, solid-state 27Al NMR spectroscopy (Bardy et al., 2007) and electron paramagnetic resonance (EPR) (Fritsch et al., 2009). Al and Fe are accumulated in distinct horizons of waterlogged podzols (Fig. 4). At the margin of the podzolic areas, Fe3+ bound to organic matter is preferentially stored in the topsoil A horizons and is also found at greater depth in the Bh horizons (Fig. 4a). In contrast, Al bound to organic matter is particularly abundant in the subsurface Bhs horizons of weakly differentiated podzols and at greater depth in the Bs (or BCs) horizons of better differentiated podzols (Fig. 4b). Such separation of secondary Fe- and Al-containing phases (Jersak et al., 1995) is in agreement with the physical fractionation of organic compounds in distinct Bh and Bs (or BCs) horizons of podzols (Fritsch et al., 2009). It also suggested that Al is mostly chelated to oxygen-containing functional groups of DOC and, in contrast, Fe is bound to organic residues and black organic colloids with abundant aliphatic and aromatic functional groups. Organo-metallic complexes are either formed in situ (e.g. in Bhs and Bs or BCs horizons) or translocated and accumulated at greater depth from AE to Bh for Fe3+ and from Bhs to Bs (or BCs) for Al, that is from weakly to well differentiated podzols. Most metal complexes concern Al, the concentration of Fe3+-humus complexes being limited by the reducing conditions prevailing in organic-rich and waterlogged environments (Fig. 4). Organo-metallic complexes are abundant at the margins of the podzolic areas in weak acidic weathering fronts (pH > 4.5) (Do Nascimento et al., 2008; Jansen et al., 2003). In contrast, they are less abundant downslope in leached and more acidic (pH < 4.5) podzols (Alo + 1/2Feo < 5 g.kg−1), likely due to the release of metals (mostly Al) to soil solutions (Boudot et al., 1996) as suggested by the decrease of the carboxylate over carboxylic contributions of the organic matter (Allard et al., 2010; Bardy et al., 2007, 2008; Do Nascimento et al., 2004). The incision of waterlogged podzols (as observed in the Curicuriari site) lowers the perched water tables, enhances the bio-mineralization of organic matter and reduces the accumulation of organo-metallic complexes in better-drained podzols. These changes also restrict the lateral development of podzols in the landscapes.
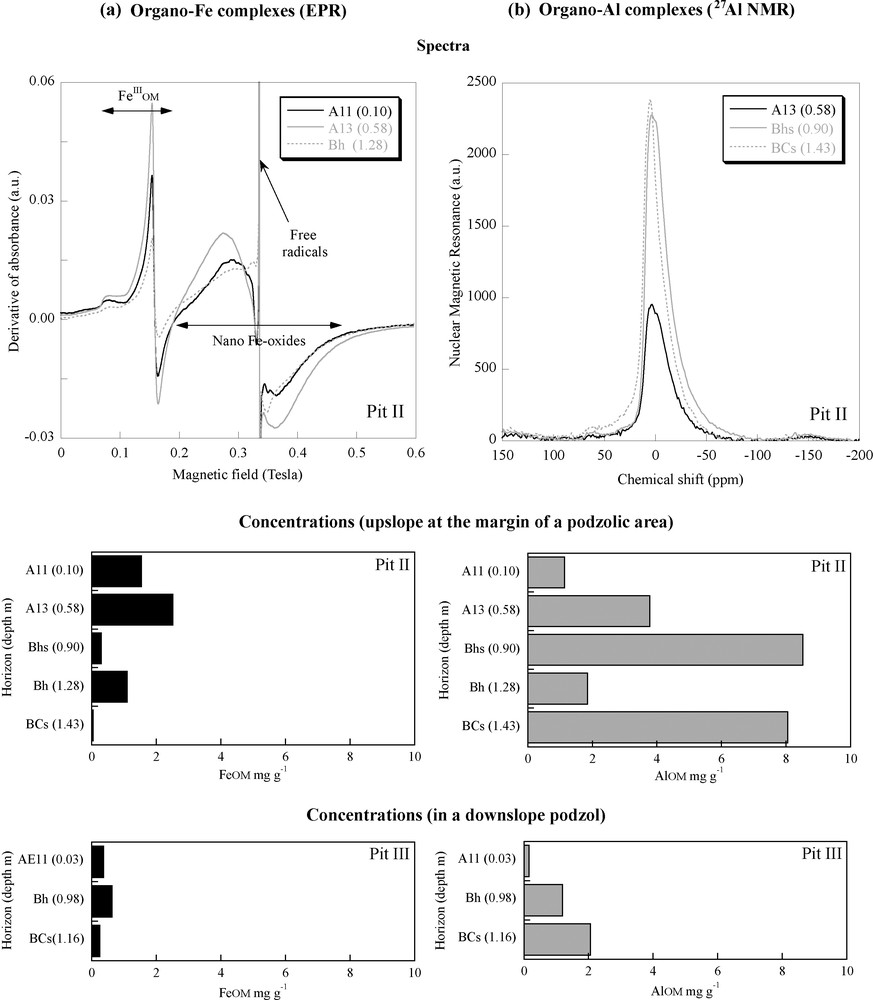
(a) EPR and (b) 27Al NMR spectra of organo-metallic complexes from clay-size fractions of podzolic horizons (removing their mineral contribution) at a margin of a podzolic area (lateral podzolic front at the Jau site). Spectroscopic data and total chemical analyses were used to assess the concentrations of Fe and Al bound to organic ligands at a margin of a podzolic area and in a downslope podzol (Jau site) (adapted from Bardy et al., 2007 and Fritsch et al., 2009). Masquer
(a) EPR and (b) 27Al NMR spectra of organo-metallic complexes from clay-size fractions of podzolic horizons (removing their mineral contribution) at a margin of a podzolic area (lateral podzolic front at the Jau site). Spectroscopic data and total chemical analyses ... Lire la suite
Spectres (a) RPE and (b) RMN de 27Al de complexes organométalliques dans les fractions argileuses d’horizons podzoliques (débarrassés de leur contribution minérale), à la bordure d’une aire podzolique (front latéral de podzolisation du site du Jau). Les données spectroscopiques et les analyses chimiques totales ont été utilisées pour estimer les concentrations en Fe et Al liées aux ligands organiques, à la bordure de l’aire podzolique et dans le podzol aval (site du Jau) (adapté d’après Bardy et al., 2007 et Fritsch et al., 2009). Masquer
Spectres (a) RPE and (b) RMN de 27Al de complexes organométalliques dans les fractions argileuses d’horizons podzoliques (débarrassés de leur contribution minérale), à la bordure d’une aire podzolique (front latéral de podzolisation du site du Jau). Les données spectroscopiques et ... Lire la suite
4 Tracing weathering processes in waters of soil and river systems
We measured the total Fe and Al concentrations and the Fe2+/Fet ratio (redox status of Fe) in the perched and ground waters of the Jau and Curicuriari sites and surface waters of the hydrologic Negro River network. We also quantified the Fe3+ bound to organic matter in suspended matters (FeOM) sampled by tangential-flow filtration and EPR spectroscopy, in order to reveal sources and evolution of organic matter and associated metals from upland soils to major river systems (Allard et al., 2010).
Total Fe concentrations are often large in the upslope deep groundwater of laterites and also in the lateral weathering front of podzols together with large concentrations of total Al (1 and 2, respectively, in Fig. 5). Total Fe and Al concentrations are low in the perched waters of the downslope podzols (3 in Fig. 5) due to quick dilution effects by rainfalls (Do Nascimento et al., 2008). FeOM concentrations in suspended solids of black waters (mostly colloidal and organic in nature) from podzolic environments (i.e. perched waters of podzols and surface waters from their associated brooks) are also low (Fig. 6) (Allard et al., 2002, 2010) and equivalent to those measured in the A and Bh horizons of podzols (Fig. 4a) (Fritsch et al., 2009). FeOM concentrations are several times larger in the main rivers and are inversely correlated with the Fe2+/Fet ratio (Fig. 6), indicating more reducing conditions in podzols than in laterites and main rivers of the Negro River watershed (Allard et al., 2002, 2010). Low electrolyte concentrations and predominance of Fe2+ in waterlogged podzols result from the fast transit of rainfall waters in bleached sands and the development of intense reducing conditions in organic-rich soils (Allard et al., 2010; Do Nascimento et al., 2008; Peretyashko and Sposito, 2006). In the main rivers, changes result from mixing of podzolic waters with groundwaters of laterites (Allard et al., 2010). Because of such end-members mixing and the oxygenation of surface waters, the concentration of Fe3+ bound to organic matter increases.
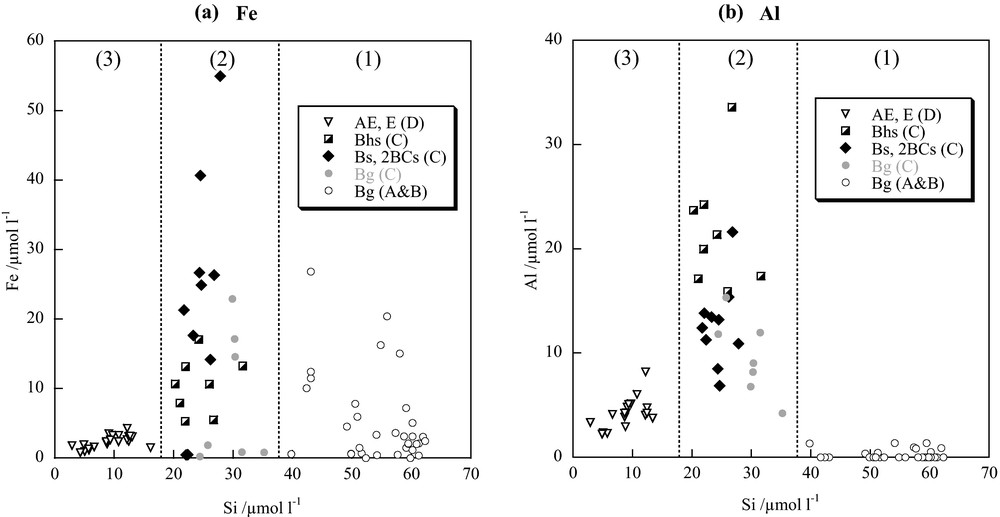
(a) Fe and (b) Al contents as a function of the Si concentration in ground and perched waters of (1) the upslope laterites, (2) the transition zone and (3) the downslope podzols at the Jau site (adapted from Do Nascimento et al., 2008).
Teneurs en (a) Fe et (b) Al, en fonction de la concentration en Si des eaux des nappes profondes et perchées (1) des latérites amont, (2) de la zone de transition et (3) des podzols aval du site du Jau (adapté d’après Do Nascimento et al., 2008).

Variation of the Fe2+/Fet ratio in solutions as a function of the concentration of Fe3+ bound to organic matter (FeOM) in suspended matters from the Negro River basin: black waters of podzolic environments (black dots), mixed waters of the Negro River and its upslope Curicuriari tributary (grey dots) and brown waters draining predominantly the upland laterites and the floodplain (Igapo) of the Jau site (white circles) (adapted from Allard et al., 2010).
Variation du rapport Fe2+/Fet des eaux en fonction des teneurs en Fe3+ complexés à la matière organique (FeOM) dans les matières en suspension du bassin du Rio Negro: eaux noires des environnements podzoliques (points noirs), eaux de mélange du Rio Negro et son tributaire amont le Curicuriari (points gris) et eaux brunes drainant essentiellement les paysages latéritiques et la plaine alluviale inondable (Igapo) du site du Jau (cercles blancs) (adapté d’après Allard et al., 2010).
The reduction of iron initiated in the upslope laterites, the production of abundant organic matter in the downslope podzols and the oxidation and complexation of Fe3+ on organic colloids in rivers are key mechanisms in the quick transfer of iron to the Negro River. Podzolic environments in the upper Amazon Basin correspond, therefore, to a sink of carbon and chelated metals (mostly Al) for soils and a major source of organic colloids and metal cations either soluble (Al) or chelated (Fe) for river systems.
5 Conclusions
Structural and mineralogical investigations of lateritic landscapes provide a multiscale approach of the history of the Amazon Basin and suggest a coherent integrated model of soil formation and evolution, adapted to the humid tropical regions as well as to altered water regimes over geological time periods. In such a model, the formation of laterites in freely drained environments involves seasonal and alternating dissolution/crystallization of secondary minerals. In waterlogged, reducing and acidic environments of the landscapes, the dissolution of clay minerals prevails together with the organic complexation of metal cations (Fe and Al).
In freely drained lateritic environments, dissolution/crystallization reactions mostly involve kaolinites at depth and Fe-oxides near the surface. These reactions contribute to an upward decrease in grain size and an increase in crystal defects and substituted impurities. Variations in mineral crystal-chemistry result from the downward advancement of two major weathering fronts and the overprinting of two stages of laterite evolution. The first front preserves the initial supply of Fe and Al (ferrallitisation) but leads to the relative accumulation of secondary minerals through the dissolution of quartz and the replacement of ordered kaolinites by disordered ones. The second weathering front corresponds to the mobilization and further accumulation of Al in more hydroxylated minerals, i.e. aluminous goethite and gibbsite (allitisation). Weathering then results from greater water and Al activity, and lower Si activity in soil solutions, leading to soil yellowing, clay depletion and soil structure breakdown. These gradual changes of pattern in the topsoil are mostly due to soil aging or more humid climates. Consequently, they are commonly better developed in the thick laterites developed on various geological substrates of the old Ucayali Peneplain than in the thinner laterites formed on the more recent Iça formation of the upper Amazon Basin.
The spatial development in space and time of local perched waters and regional groundwaters leads to dramatic soil changes in lateritic landscapes with mineral dissolution prevailing over crystallization and the carbon cycle characterized by C-storage. In clayey laterites, the selective dissolution of Fe-oxides under reducing conditions leads to the development of bleached areas locally linked to iron-cemented formations (thin iron pans or plinthites). In yellowish and clay-depleted laterites, the downward accumulation of organic acids in reduced environments favours the weathering of clay minerals (Fe-oxides, gibbsite and kaolinite) and the formation of organo-metallic complexes, Fe and Al being preferentially bound to distinct organic functional groups. Soil patterns in well-expressed podzols illustrate the physical fractionation and accumulation of organo-metallic complexes during the cyclic recession of perched waters. Upslope at the transition between the clay-depleted laterites and podzols, the weathering of clay minerals and the sequestration of metals by organic acids are particularly efficient, whereas the release of metals (mostly Al) prevails downslope in more acidic podzols. The transit of rainfall waters in these strongly leached, reduced and acidic podzols is short. It favours the downward accumulation of black organic colloids in bleached sands, and the export of organic colloids and metals to river systems. Water mixing and oxygenation of downstream waters rapidly diminish the chemical and organic fingerprints of podzolic environments.
Poorly drained and waterlogged environments of the low elevation plateaus in the upper Amazon Basin have different origins. They are inherited from sedimentary structures, from more humid climates following the definitive uplift of the Andes and from chemical erosion of the lands and soil aging. In this regard, hydromorphic soils developed on the Iça formation are likely inherited from former drainage networks of lacustrine origin at a time where the Amazon River system was flowing towards the Pacific Ocean. Widely extended Podzols have formed on different geological formations of the Ucayali Peneplain in the highest rainfall area of the upper basin (> 3.5 m). Thus these soils belong to the former Amazon lowland whose origin likely goes back to the birth of the modern Amazon River system, c.a. 2.5 My ago, and the formation of the low elevations plateaus. Such processes are still acting today. However the incision of widely extended podzols alters their hydrological regime, limits their lateral expansion and ultimately feeds the river systems with bleached sand deposits.
Acknowledgements
The studies on laterites and podzols in the Amazon Basin have been supported by the IRD-USP DYLAT project (Dynamique des latérites) and by PRONEX (1997–2003), FAPESP (2004–2006, 2007–2009), INSU-ECCO (2004–2006) and CAPES-COFECUB (2004–2009) programs.