1 Introduction
We learn from modern neurobiology that complex biological systems, such as the vertebrate brain, are built from similar, repeated units. In the central nervous system (CNS), these units, or modules, are composed of networks that have opposite effects: excitation and inhibition. Initially, excitation was assumed to play the dominant role in information processing, whereas inhibition served mainly to eliminate excessive activity. From this point of view, local inhibitory neurons appeared to be simple elements that regulate excitatory neuronal activity. Thus, as in Yin and Yang philosophy, the activity of a large network of principal excitatory (glutamatergic) neurons was purposefully balanced by local-circuit inhibitory neurons.
Today, it is clear that the inhibitory interneurons occupy distinct positions within brain microcircuits where they mainly form local connections. One of the most popular cell types studied so far in the brain is the γ-aminobutyric acid (GABA)-releasing neuron, also referred to as the local-circuit inhibitory interneuron. Recent investigations have indicated that the initial concept of interneuron is an oversimplification that requires to be readjusted to accommodate for cellular types and functions that would not strictly fit the definition above cited. For instance, combined anatomical, electrophysiological, and pharmacological approaches have recently allowed a better insight into the roles played by interneurons at several distinct levels. At the cellular level, such functions range from governing action potential generation, firing pattern, precise timing of the discharge of individual principal cells in relation to the emergent behaviour of the entire cell assembly, membrane potential oscillations and dendritic calcium spikes. At the network level, inhibitory interneurons are considered important in controlling synaptic strength and plasticity as well as the generation of large-scale synchronous oscillatory activity (reviewed in [1]).
Bearing in mind such a key role played by inhibitory interneurons for normal brain functioning, it is anticipated high impact of the continuous interneurons renewal in the adult olfactory bulb. Indeed, there is a growing interest in studying inhibitory action in olfactory plasticity. The reasons for such interest are manifold. First, olfaction is a sensory modality already functioning at early embryonic stages. It is a primary mechanism for receiving information about the world, and early olfactory experience and learning are crucial for survival. Second, the high dimensionality of the system offers multiple sites for regulation. Finally, the olfactory system exhibits lifelong turnover of both peripheral sensory neurons and inhibitory bulbar interneurons. We will summarize the current knowledge of this neurogenesis in the adult olfactory system. We will see that adult neurogenesis comprises a complex process, beginning with the division of a precursor cell (or potentially even before) and ending with the existence of a new functioning neuron integrated into the olfactory system. Here, we present adult neurogenesis as a process, not an event, being much more than just the division of a precursor cell. Our general hypothesis underlying this review is that adult neurogenesis is a particularly prominent manifestation of a far more general principle of neurobiology: the idea that the brain development never ends and that plasticity can be taken as continued development. Due to space constraints, this review only focuses on adult neurogenesis of mammals.
2 Building a neuronal network during embryogenesis
During embryogenesis, the migration of postmitotic neurons from their site of origin to their final destination, where they make synaptic connections, is a fundamental cellular event essential for building large neuronal assemblies [2]. As illustrated in Fig. 1, cell migration within the developing forebrain can roughly be subdivided into two categories, radial and non-radial.
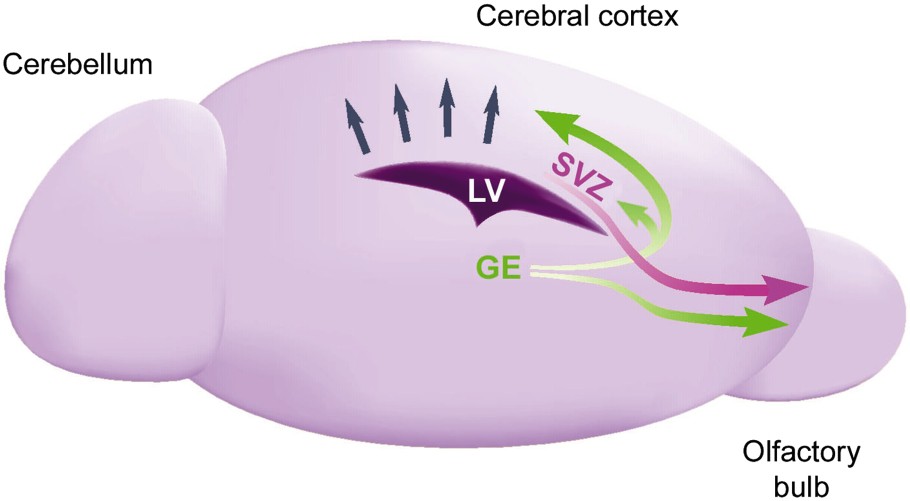
Migration of neuroblasts during embryogenesis and adulthood. Radial migration of cortical projecting neurons from the ventricle to the developing cortex is indicated by blue arrows. Tangential migration of neuroblasts, during embryogenesis, from the ganglionic eminence (GE) to the neocortex, the olfactory bulb, and the subventricular zone (SVZ) of the lateral ventricle (LV) is indicated by green arrows. The pink arrow indicates cell migration from the SVZ to the adult olfactory bulb.
The substrate for radial migration is the radial glia, which in the developing neocortex extends long, unbranched processes from the ventricular zone lining the lateral ventricle out to the cortical pial surface. All cortical neurons in primates, including humans, are generated during the first half of gestation within the proliferative zone lining the surface of the cerebral ventricle. After their final mitotic division, cortical neurons migrate away from their place of birth, toward the pial surface, where each successive generation passes the other and settles in an inside-out pattern within the cortical plate. Radially migrating cells primarily give rise to projection neurons that express the neurotransmitter glutamate (Fig. 1; blue arrows). In contrast, non-radially migrating cells that are generated in the ganglionic eminence give rise to a majority of the interneurons, and possibly other cell types, such as oligodendrocytes, in the dorsal telencephalon (Fig. 1; green arrows). It is noteworthy that not only does the ganglionic eminence send interneurons dorsally to the overlying cortex but it also provides the subventricular zone (SVZ) of the postnatal lateral ventricle with proliferating neural stem cells (indicated by the small green arrow in Fig. 1). In this zone, they constitute a germinal region that persists into adulthood. The SVZ, once thought to provide the overlying cortex with glia late in development, is now also considered to constitute a steady supply of newly generated interneurons for the adult olfactory bulb (pink arrow in Fig. 1).
Below we summarize how early studies in this field has provided the framework for our current understanding of how newborn interneurons reach the adult olfactory bulb microcircuits. We will also review the more recent findings that have dramatically reshaped the view of how newborn neurons are integrated within adult neuronal networks. At first glance, this neurogenesis may be seen as a putative response to the death and turnover of the primary olfactory receptor neurons that also occurs throughout life. We will demonstrate that in fact adult neurogenesis does not simply recapitulate embryogenesis but rather endows the bulb with unique properties.
3 Neurogenic permissiveness
Adult neurogenesis exemplifies an unforeseen regenerative (see Appendix A) capacity of the mature mammalian CNS and raises an intriguing question: why is active neurogenesis only retained and restricted to few regions in adult mammals? In the embryo, interneurons of the olfactory bulb are derived from neuronal precursor cells that migrate from the ganglionic eminence [3]. Postnatally, they are derived from ‘neurogenic’ region. Neurogenic implies two things: first, the presence of immature precursor cells from which new neurons can develop, and second, a certain type of microenvironment that is permissive for neurogenesis to occur. In the adult mammalian brain, there are two known neurogenic regions, the hippocampus, and the SVZ/olfactory system. We refer to the rest of the brain as ‘non-neurogenic’, although this categorization might ultimately turn out to be premature.
Currently, the key factor considered for neurogenic permissiveness is whether the local microenvironment allows an implanted neural precursor cell to develop into a neuron. There is one ultimate test for qualifying a region as neurogenic: a neural precursor cell implanted in a neurogenic region should develop into a neuron and when grafted onto a non-neurogenic region it should become a glial cell or die. Thus, the definition of ‘neurogenicity’ is based on a general and physiological neurogenic permissiveness. It is not based on the presence or absence of neural precursor cell alone, and it is not influenced by the possibility that such a promotion could be induced under certain unusual conditions. The key questions become, then, what makes a brain region neurogenic? How is neurogenic permissiveness defined on a molecular and cellular level?
To sum up, neurogenic regions are defined by (1) the presence of neural precursor cells and (2) the presence of a microenvironment consisting of cell–cell contacts and diffusible factors, that promotes neural development from intrinsic precursor cells, and whose neurogenic potential can be tested by the implantation of neural precursor cells into this region. Consequently, non-neurogenic regions may contain precursor cells but lack distinctive germinative cell clusters as well as the permissive microenvironment that under physiological conditions would promote neurogenesis from local or implanted precursor cells. So far, transplantation into the adult brain has yielded neurons only in the olfactory system and the hippocampus, although the same precursor cells could give rise to neurons under appropriate in vitro conditions [4]. This makes matters even more complex: how much of adult neurogenesis lies in the precursor cells, and how much is dictated by the cellular microenvironment?
At the core of the concept of neurogenic permissiveness is the assumption that precursor cells in the neurogenic zones are embedded into a microenvironment with which they form a functional unit, the so-called stem cell niche (see Appendix A). The idea that somatic stem cells reside within specific anatomical locations termed ‘niches’ was first suggested on the basis of transplantation studies of haematopoietic progenitors in the 1970s. Recent studies in several model systems, such as Drosophila germline and mammalian skin, intestine and bone marrow, have provided cellular and functional descriptions of niches as microenvironments that not only anatomically house stem cells but also functionally control their development in vivo. In the adult mammalian brain, we are just beginning to identify these cellular and molecular elements that characterize specifically neurogenic niches either in the SVZ or in the hippocampus, and the mechanisms by which the full range of adult neural stem cells development is regulated. In both cases, these niches consist of the precursor of cells, astrocytes, endothelial cells, microglia or macrophages, extracellular matrix, and close contact with the basal membrane, but all of these elements are present in the SVZ and the hippocampus with unique proportions.
We now know that germinative niches serve two functions: the maintenance of the stem and progenitor cell activity (see Appendix A), and the promotion of neuronal differentiation (see Appendix A). In non-neurogenic regions, precursor cells appear to be maintained in a different, niche-independent manner and no neuronal development occurs.
4 Development and function of newborn interneurons in the olfactory bulb
Neuronal precursor cells that migrate into the olfactory bulb through the mean of a rostral migratory stream (RMS), originate from the SVZ in the lateral ventricles of the forebrain [4]. Within the SVZ, the neural precursor cells (see Appendix A) are considered stem cells since they proliferate and give rise to several different cell types [4]. Unlike other immature neurons, precursor cells of the SVZ migrate tangentially without the aid of radial glial cells, and continue dividing during their migration to populate the olfactory bulb where they differentiate into local interneurons (Fig. 2). Analysis of in vitro results gave rise to the current view that the adult SVZ generates stem cells capable to produce the three major cell types of the CNS (e.g., oligodendrocytes, astrocytes, and neurons). When the neuroblasts reach the olfactory bulb, they migrate radially and of those that survive, approximately 95% and 3% differentiate into GABAergic and dopaminergic interneurons, respectively. Below we discuss some of the recent findings that support functional implications for producing and integrating newborn neurons into the adult brain.
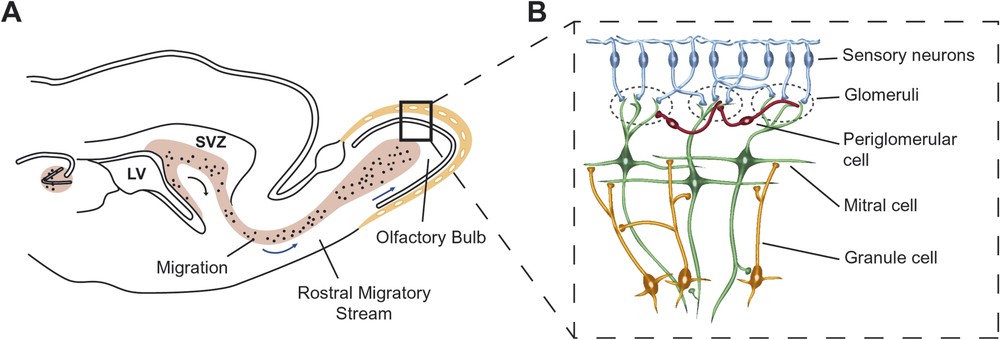
(A) The hippocampus and the subventricular zone (SVZ)-olfactory bulb pathway constitute the two neurogenic zones in the adult CNS. Blue arrows indicate the tangential migration of neuroblasts (dots) toward the olfactory bulb. Neurogenesis refers to the replacement of glutamatergic neurons in the hippocampus and of bulbar local interneurons in the olfactory bulb. LV, Lateral ventricle. (B) Wiring of the olfactory bulb. Each sensory neuron expresses only one of the 1000 odorant receptor genes and the axons from all cells expressing that particular receptor converge onto one or a few glomeruli in the olfactory bulb. The nearly 2000 glomeruli in the rat olfactory bulb are spherical knots of neuropil, about 50–100 μm in diameter, which contain the incoming axons of sensory neurons and the apical dendrites of the main input–output neuron of the olfactory bulb, the mitral cell. Mitral axons leaving the olfactory bulb project widely to higher brain structures including the piriform cortex, hippocampus, and amygdala. Processing of the olfactory message into the bulb occurs through two populations of interneurons: periglomerular cells and granule cells.
Adult neurogenesis is scientifically interesting for two reasons that to some degree are independent. In the context of brain function in health and disease, the ability of the brain to make new neurons is important. At the same time, adult neurogenesis can be used as a model system for investigating central questions in applied stem cell biology. The focus, then, is to study factors controlling the development of new neurons under conditions prevailing in the adult brain and discern what has to be achieved if stem cells or immature neurons are implanted in the brain. For most of the young history of neurobiology, the adult brain was essentially considered non-neurogenic, if not antineurogenic. However, the adult brain routinely does exactly what tissue engineers intend to do in (stem) cell-based therapy for the brain: make new neurons.
Although the ongoing neurogenesis and migration have been extensively documented, their functional consequences are still not clear. Is adult neurogenesis an atavism, an empty-running leftover from evolution? For what is adult neurogenesis good? Is there anything special about the new neurons besides their later birth? How are they integrated into the existing networks? How could they possibly contribute to brain function? How does the brain ‘know’ that more neurons are needed, and how is this demand translated into signals a precursor cell can ‘understand’? Since the largest neuronal population in the olfactory bulb consists of granule cells that outnumber the output neurons by at least 100:1 [5] and one granule cell contacts several mitral cells, and each mitral cell, in turn, contacts a large number of neurons from the piriform cortex, the upstream location of the addition of transient newborn neurons in the olfactory bulb is ideal for amplifying the neurogenic effect within the entire olfactory pathway. We postulated that if newborn interneurons generated throughout life are necessary for olfaction, then modification of the migration processes, i.e., an alteration of the recruitment of newborn interneurons, affects olfactory processing. Using transgenic mice characterized by reduced adult neurogenesis tested this hypothesis. NCAM-mutant mice were used since they show reduced neuroblast migration. Quantifying the level of bulbar neurogenesis revealed a 40% reduction in the number of newborn interneurons in adult knockout mice. This impaired neurogenesis was accompanied with altered odour discrimination [6] revealing a specific role for newborn interneurons in downstream coding of olfactory information. This observation was further supported using a different transgenic line in which GABAA receptor-mediated synaptic inhibition is altered [7] as well as a theoretical approach [8]. These data indicated that a critical level of inhibition, mediated by the activation of GABAA receptors localized on the secondary dendrites of bulbar principal neurons, is crucial for olfactory processing. In addition, since the olfactory bulb is also involved in consolidating processes associated with long-term odour memory, we proposed that changes in the number of GABAergic interneurons regulate both olfactory perception and memory.
To shed light on this issue, we investigated whether a change in the number of newborn bulbar interneurons alters olfactory memory. To challenge the level of neurogenesis, we raised animals in a complex olfactory environment [9]. Exposure to an enriched surrounding has many positive effects on brain structure and function, including an increased number of dendritic branches and spines, enlargement of synapses, increased glial numbers, and improved performance in tests of spatial memory [10]. Exposure of adult rodents to increased environmental complexity induces neurogenesis in the hippocampus [11]. We investigated whether an odour-enriched environment increases the number of newly formed interneurons in the olfactory bulb and, if so, whether learning or memory is modified. We found that enriched mice produce twice as many newborn interneurons. This increase is specific of the olfactory bulb since the second neurogenic zone, the dentate gyrus of the adult hippocampus, remained unaltered. In addition, animals showing higher neurogenesis retain learned olfactory information for longer periods than controls [9]. In particular, enriched animals are able to recognize familiar odours in a more durable and stronger way (i.e., they express a high resistance to retroactive interference) than animals raised in standard conditions. Although the potential consequences of an enriched environment on synaptic efficacy and/or mitral cell activity were not specifically explored, these results support the existence of a correlation between newborn bulbar interneurons population size and odour memory.
5 Integration of newborn neurons into adult circuits
It has been shown that neurogenesis in the adult, as in the immature, brain includes cell division, differentiation and migration, as well as synapse formation and programmed cell death. Until recently, however, only morphological (e.g., [12]) and immunohistochemical studies (e.g., [13]) supported the idea that cells generated in the SVZ in adulthood become true bulbar neurons. Lack of direct functional evidence left open the possibility that the newly generated neurons never fully integrate into the bulbar network. In addition, whereas much work had been devoted to elucidating the birth and migration mechanisms of new SVZ neuroblasts, no information was available concerning their maturation. Such information is essential to appreciate how newly formed neurons become functionally integrated into adult neural networks. To obtain this type of information, we characterized the electrophysiological properties of newborn cells during their migration and differentiation using a replication-defective retrovirus that carries GFP. GFP-labelled neurons were visualized in acute olfactory bulb slices prepared following different times after viral injection. We demonstrated that integration of functional neurons in adult neural networks is achieved through sequential steps in a highly regulated manner. These steps include proliferation of the neural stem cell, differentiation into an immature neuron, migration to the final location, growth of dendrites and formation of synapses with other neurons in the circuit, and ultimately maturation into a fully functional neuron [14].
As early as 14 days after birth, some of the new neurons have reached the olfactory bulb and migrated radially to their final position. At this stage, they already display dendritic spines, which suggests that they are receiving synaptic inputs. Indeed, soon after new cells enter the layers of the olfactory bulb, they express functional GABA and glutamate receptor channels and display voltage-dependent potassium currents typical of more mature neurons. Spontaneous synaptic activity then emerges soon after radial migration is completed. Interestingly, GABAergic and glutamatergic synapses impinging onto newborn neurons were established sequentially, GABAergic synapses being established first. In contrast to the sequential acquisition of synapses during embryogenesis, we found NMDA responses in neurons that were unable to fire and had started to receive GABAA and AMPA inputs [14]. The rules that govern the incorporation of adult-generated neurons into mature neuronal circuits may thus differ from those previously described in the developing brain. In the adult brain, the correlated maturation of intrinsic electrical properties with synaptic activity of newborn cells may influence both maturation and integration processes and therefore represents a mechanism by which neuronal activity may regulate neurogenesis.
Future studies will need to determine if the newborn neurons make functional synapses with their downstream target neurons and release appropriate neurotransmitters, in order to demonstrate unequivocally their integration into adult circuitries. However, our data indicate that adult-generated neurons, derived from neural stem cells in the SVZ, could become physiologically mature into olfactory bulb circuitry. Surprisingly, the spiking activity of newly generated neurons does not occur until late into the maturation process [14]. The delayed maturation of excitability may serve to prevent disruption of the adult pre-existing circuitry's function by the newborn cells. This characteristic further illustrates that maturation of adult-generated neurons does not recapitulate the embryogenesis, in part because of the high neuronal activity already occurring in the adult mature circuitry. In central sensory pathways, this activity results from external stimuli that lead to experience-dependent changes in anatomical connectivity, both during late development and in adulthood. Because these anatomical changes involve synaptic reorganization, understanding the maturation of synaptic transmission at newly formed synapses may provide important insight into how experience modifies already functioning neural circuits. The late occurrence of NMDA receptors and the maturation sequence of glutamatergic synapses we have reported may indicate unique maturation sequences adapted to experience-dependent maturation of adult neural circuits. Do newly generated bulbar neurons differ from older granule cells? Functionally, are there two types of granule cells, i.e. a larger population that is generated during development and early postnatal life, and another one generated during adulthood? Answering these questions will clearly influence the final interpretation of the role of adult neurogenesis. Finally, it should be kept in mind that only about half of the newly generated bulbar interneurons survive more than several days after having reached their mature state. We do not yet know if this survival time is sufficient for these cells to play a significant role in olfactory bulb function. Obviously, understanding the role of neuronal replacement in the adult brain implies that one has to take into account that half of the newborn neurons are transient and will be replaced through a process that has to be discovered.
6 Properties brought by adult-generated neurons
‘Loss of function’ experiments could help to determine the role of adult neurogenesis in learning and memory. A focal and non-genetic approach to eliminate selectively dividing cells is represented by irradiation. Studies in animal models have shown indeed that exposure to therapeutic doses of radiation leads to ablation of adult neurogenesis but not gliogenesis [15,16]. This dramatic reduction of adult neurogenesis results from the combined effects of acute cell death, decreased proliferation, and neuronal differentiation of the neural progenitors. Hence, gamma or X-ray irradiation induces apoptosis in dividing cells, sparing post-mitotic cells such as mature neurons. Therefore, irradiation is a very flexible technique not invasive and requires no surgery. It is an ideal tool to target adult neurogenic zones without damaging neighbouring tissue. Long-term reductions in neurogenesis within the subgranular zone (SGZ) of the dentate gyrus have previously been reported after low-dose irradiation of the heads of adult rodents [15,16]. Experiments showing that neuronal precursors are more sensitive to radiation than glial precursors [17,18] indicate that the effects of irradiation are very selective for depletion of new neurons in the brain. For instance, 2–3 fractionated, low doses of gamma or X-rays, repeated at 24–48 h, are less damaging to the brain tissue than a single large dose (reviewed in [19]). A local irradiation of the SVZ by shielding body and most of the head should not directly affect the olfactory system, the hippocampus, or other tissues. In several laboratories, experiments using irradiation are in progress to reveal the functional consequences of specific neurogenesis ablation in the SVZ (Fig. 3).
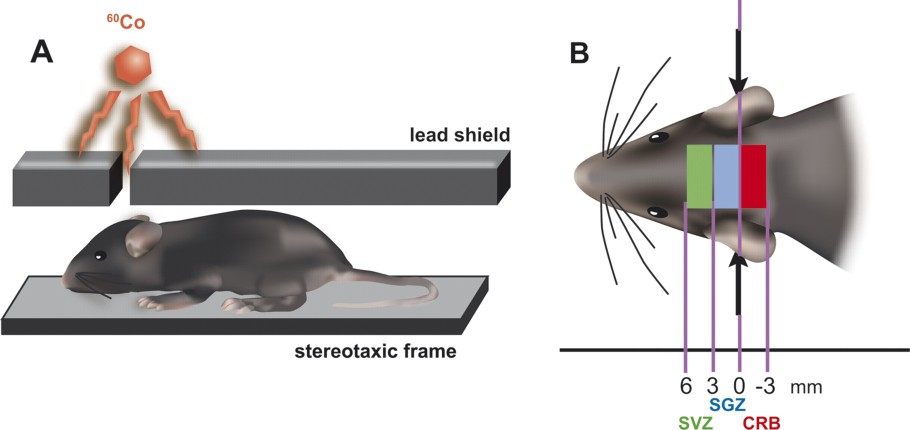
Gamma-ray treatment ablation of cell proliferation in the SVZ. Mice are anesthetized, placed in a stereotaxic frame, and exposed to cranial irradiation (A). A lead shield protects the mouse body while exposing the SVZ, the SGZ, or the cerebellum (CRB) to gamma rays (B). Five grays are delivered on days 1, 3 and 5, before behavioural tests beginning on day 28.
Although recent estimates suggest that the quantity of newly produced neurons in adulthood is much greater than previously thought, the rate of the adult neuronal production still remains lower than during development. As a result, if the functional properties brought by adult-generated neurons are similar to those brought by neurons generated in early life, then adult neurogenesis has to be considered insignificant [20]. In contrast, if adult-generated neurons have unique properties that increase their impact relative to more mature neurons, then one can hypothesize that their constant integration into the functional circuitry has tremendous effects. Recent observations made both in the hippocampus and in the olfactory bulb support the second hypothesis. Young granule cells in the adult dentate gyrus appear to exhibit robust plasticity that, in contrast to mature granule cells, cannot be inhibited by GABA. These newborn neurons may respond preferentially to modulation by stress hormones. Learning has been shown to increase the number of new neurons in the hippocampus. Running increases both the number of new dentate gyrus cells and performance on a hippocampal-dependent task whereas a decrease in the number of new granule neurons is correlated with impaired performance for such a task (reviewed in [21]). It is thus possible that since new neurons are structurally plastic, they are highly susceptible to changes in the animal's environment and to different life experiences. This suggests that adult neurogenesis is functionally important since its result is a continual influx of neurons that are, at least temporarily, immature with unique physiological properties. As described above, bulbar interneurons play an essential role in shaping the olfactory information that reaches the olfactory cortex. Since adult-generated cells born in the SVZ differentiate exclusively into bulbar interneurons, they probably contribute to essential aspects of olfactory processing. Although experimental studies and modelling have already provided arguments in favour of a role for newborn granule cells in olfactory discrimination, a more general function remains to be characterized. It is noteworthy that the functional benefit derived from adult neurogenesis cannot be acute since it takes several weeks to generate a functionally integrated new interneuron. We have shown that newborn cells extend neurites within a couple of weeks after cell birth and it is obvious that the new connections cannot constitute a response to the particular functional event that triggered neurogenesis since these connections will be in place only well after the triggering event is over. Thus, adult neurogenesis of the olfactory system has to be considered rather a long-term than a short-term adjustment of the bulbar circuitry to a higher level of experience governed by olfaction.
7 Adult neurogenesis: a neural basis for experience-induced plasticity
It is common sense to state that mind and brain benefit from an ‘active’ life; this is backed by several observations such as those from epidemiological studies. It is also well established that both physical and intellectual activities have a positive influence on the incidence of neurodegenerative disorders and on cognitive decline. Due to continuous neurogenesis in this region of adult animals, the olfactory system provides a unique model to study the role of sensory-driven activity and its benefits. In the olfactory bulb, the role of environmental-induced activity can be explored not only during the neonatal period, when refinement and stabilization of developing structures takes place, but also in adulthood, when a more delicate regulation occurs. Several studies have demonstrated that modified levels of activity do not affect proliferation and tangential migration of neuroblasts. However, other studies have shown that in adults, odour enrichment increases the number of newly generated granule cells while odour-deprivation elevates neuronal death in the olfactory bulb. This indicates that sensory-driven activity may play an important role in the survival of progenitors and/or in their guidance from their migratory stream to the bulb. This, in turn, would lead to great changes in the number of newborn interneurons and therefore alter the inhibitory–excitatory balance in the bulbar neuronal networks. Despite clear evidence that adult neurogenesis can be regulated, it should be kept in mind that most data on adult mammalian neurogenesis have been derived from laboratory animals, kept under rather artificial conditions compared to their natural habitat. Even the odour-enriched environments of our experiments are arguably much deprived compared to wildlife conditions.
Remarkably, when experience-induced plasticity is triggered, opposite results regarding neurogenesis are found in young and adult animals. Challenging the level of sensory activity in adults alters their olfactory memory whereas sensory deprivation performed at early stages does not affect olfactory performance. Thus, changing the level of sensory inputs may trigger homeostatic compensatory mechanisms only in newborn animals. During development, sensory activity might be important for refinement and maturation of anatomical and physiological connections established in an activity-independent manner, thus playing an instructive role on neurogenesis. In contrast, sensory activity during adulthood might play an informative role for newborn interneurons arriving at the olfactory bulb; this would allow them to reach a proper site in the mature neuronal network. Supporting this hypothesis are observations indicating that almost half of adult-generated neurons die shortly after reaching the olfactory bulb [13]. It is conceivable that an alteration in the inhibitory–excitatory balance during development might have a much more profound effect on animal survival than during adulthood. Alternatively, the inhibitory–excitatory balance may be modified by sensory activity in a different way from one life stage to another.
8 Ethological relevance of adult neurogenesis
Although it is clear that the adult olfactory bulb integrates neurons throughout life, the reason for this remains unclear. What are the new neurons used for and how can an existing functional neural network integrate and even actively recruit new neurons? In the current view, the prevailing theories of cognition are based on the assumption that the adult brain is a stable network with regard to the number of neurons. According to this scheme, structural neural plasticity occurs only at the level of synapses, dendrites, and neurites. The demonstration of adult bulbar neurogenesis, i.e. the generation of new interneurons from resident neuronal stem cells and their integration in the bulbar circuit, has called this assumption into question. In the light of results on the activity-dependent regulation of adult bulbar neurogenesis, some conclusions regarding why and how new neurons may contribute to bulbar function can be made. We suggest that neurogenesis does not simply add neurons to enhance memory, but that it also strategically inserts newborn cells with unique properties. The multiple stages in the maturation of new neurons might serve as various substrates for plasticity. The newly formed neurons could provide the olfactory bulb with a large repository of plastic cells to respond better to environmental changes and to different life experiences. For instance, new neurons could respond preferentially to the modulation mediated by hormones as well as to learning and other forms of experience-induced activity. These aspects have been well documented in different biologically relevant models that we here describe briefly.
Female mice form a memory of their mating male's pheromones, a phenomenon known as the Bruce effect. This memory prevents the newly mated female from sustaining her pregnancy when exposed to pheromones of other males [22]. Such a memory is formed during mating and requires a period of about 3 to 4 h of exposure to the male's pheromones. With a microdialysis approach, Brennan and colleagues [23] explored the level changes of a range of neurotransmitters occurring when female mice form a memory of their stud male. In the accessory olfactory bulb, the main changes are a significant increase in noradrenaline release during mating and a decrease in the glutamate/GABA ratio following memory formation in response to the mating male's pheromones. The increase in GABA release illustrates a higher inhibitory tone at the reciprocal synapses. The authors interpreted such a mechanism as a way of reducing the excitation from mitral cells, which mediate the input from the mating male's pheromones, in order to prevent a pregnancy block. In contrast, exposure to a different male would activate a different subpopulation of mitral cells, not previously submitted to an increased inhibitory control, thus eliciting central pregnancy blocking mechanisms. These processes do not only occur during formation of a memory of the mating male's pheromones. Similar events have been found in the olfactory bulb of ewes when they form a specific ability to recognize their own lamb and reject alien ones that attempt to suckle [24]. After parturition, in response to odours from her own lamb but not to odours from alien lambs, an increase in glutamate and GABA release occurs in the ewe's olfactory bulb [25]. Once the ewe has established a selective bond with its lamb, the ratio of glutamate to GABA is decreased in response to the lamb's odour, suggesting, once again, the existence of changes in the gain of reciprocal synapses between mitral and granule cells.
These two examples illustrate changes in the excitatory–inhibitory balance in the olfactory bulb occurring during the establishment of specific social preferences. In addition, similar changes have recently been shown to occur in circumstances involving olfactory learning in a broader context. For instance, a conditioning procedure in which adult mice were trained with odour added to clean wood shavings through which they dig to obtain a buried piece of sugar, has revealed interesting findings. Following conditioning, the presentation of the odour previously associated with the reward induced an increase in both glutamate and GABA levels in the olfactory bulb and a decrease in the balance existing between excitatory and inhibitory transmitters. In contrast, no change was reported when animals were exposed to a non-conditioning odour, indicating that the increase in inhibition only occurs for relevant odours (for a review, see [26]). These findings emphasize the significance of changes in the excitatory–inhibitory ratio that occur in the olfactory bulb following odour learning. It is noteworthy that the neurochemistry of inhibition enhancement is reminiscent of the up-regulation of the permanent supply of bulbar interneurons that correlates with a stronger memory in odour-enriched mice. Together, these findings indicate that adult bulbar neurogenesis might be involved in many natural behaviours that yet remain to be explored.
9 Concluding remarks
We still do not know why there are new neurons in the adult brain, but researches on regulation of adult neurogenesis, as well as an increasing number of studies directly aimed at elucidating the function of the new cells, have led us to two conclusions. First, bulbar neurogenesis is affected by relevant behaviour, and in particular, behaviours specifically related to bulbar function. Second, changes in rate or extent of neurogenesis have an effect on subsequent behaviour. Thus, although behaviour can change the structure of the olfactory bulb, changes in structure can subsequently change, or at least affect, subsequent behaviours. The correlation between activity and adult neurogenesis suggests that new neurons are involved in a general aspect of bulbar function, most likely in sustaining the ability of the adult network to adapt information processing to relevant ethological needs.
The sense of smell has played essential roles during mammalian evolution. Odour representation is dynamic and highly complex, perhaps requiring a unique mechanism of plasticity. Neurogenesis, migration, and replacement of new neurons in the bulb are likely to play a part in this adaptive mechanism. Since it takes time for new neurons to mature and become synaptically integrated, adult neurogenesis may contribute to slow, long-term adjustments of the bulbar circuitry, rather than to fast and acute plastic changes. However, faster mechanisms may also take advantage of new neurons. Several new bulbar interneurons die within a month after having reached their mature state. Our results show that newborn granule cells are synaptically connected during this period of fast cell death. Selective elimination of neurons may allow for a rapid modification of the circuitry. The ability to mould the integration of new neurons and to eliminate continually older cells without depleting the neuronal population may bring into neuronal networks a degree of circuit adaptation unmatched by synaptic plasticity alone.
In this brief review, we have tackled two of the main problems regarding the function of new neurons in the adult olfactory bulb. Both are fundamental issues. First, we have discussed the role of adult neurogenesis in the context of the olfactory bulb's function. Under the assumption that the olfactory bulb processes odour information before relaying it to the olfactory cortex, we hypothesize that adult neurogenesis allows the olfactory bulb to adjust appropriately the degree of processing. Second, the existence of a pool of juvenile neurons, that enables the system to adapt to future similar situations, raises the possibility that adult neurogenesis acts post hoc to provide a structural basis for brain plasticity. Overall, we believe that research on adult neurogenesis in the olfactory bulb is not only interesting in itself, but provides a new avenue to the understanding of adult brain plasticity.
Acknowledgements
This work was supported by the Annette Gruner-Schlumberger Foundation, the ‘Fédération pour la recherche sur le cerveau’, the ‘Fondation pour la recherche médicale’ and the ‘Agence nationale de la recherche’ (ANR-05-Neur-028-01). We thank Cécile Moreau for help with illustrations.
Appendix A A glossary for stem-cell biology
Stem-cell biology is in a phase of a fast expansion and dynamic conceptual changes and is currently forming connections with a broad range of basic and applied researches. In addition, the field is simultaneously exposed to public and political scrutiny. As a result, a common language in the stem-cell community is an important tool for coherent exposition to these diverse audiences, not least because certain terms in the stem-cell vocabulary are used differently in other fields.
Asymmetric division: Generation of distinct fates in progeny from a single mitosis. Oriented division may position daughter cells in different microenvironments or intrinsic determinants may be segregated into only one daughter. Observed in some but not all stem cells and can occur in other types of progenitor cell.
Cell replacement therapy: Reconstitution of tissue by functional incorporation of transplanted stem-cell progeny. Distinct from ‘bystander’ trophic, anti-inflammatory or immunomodulatory effects of introduced cells.
Commitment: Engaging in a programme leading to differentiation. For a stem cell, this means exit from self-renewal.
Embryonic stem cell: Pluripotent stem-cell lines derived from early embryos before formation of the tissue germ layers.
Founder/ancestor/precursor cell: General terms for cell without self-renewal ability that contributes to tissue formation. In some cases, they generate tissue stem cells.
In vitro stem cell: Self-renewal ex vivo in cells that do not overtly behave as stem cells in vivo. Occurs due to liberation from inductive commitment signals or by creation of a synthetic stem-cell state.
Niche: Cellular microenvironment providing support and stimuli necessary to sustain self-renewal.
Plasticity: Unproven notion that tissue stem cells may broaden potency in response to physiological demands or insults.
Potency: The range of commitment options available to a cell.
Totipotent. Sufficient to form entire organism. Totipotency is seen in zygote and plant meristem cells; not demonstrated for any vertebrate stem cell.
Pluripotent. Able to form all the body's cell lineages, including germ cells, and some or even all extraembryonic cell types. Example: embryonic stem cells.
Multipotent. Can form multiple lineages that constitute an entire tissue or tissues. Example: haematopoietic stem cells.
Oligopotent. Able to form two or more lineages within a tissue. Example: a neural stem cell that can create a subset of neurons in the brain.
Unipotent. Forms a single lineage. Example: spermatogonial stem cells. Progenitor cell Generic term for any dividing cell with the capacity to differentiate. Includes putative stem cells in which self-renewal has not yet been demonstrated.
Regenerative medicine: Reconstruction of diseased or injured tissue by activation of endogenous cells or by cell transplantation.
Self-renewal: Cycles of division that repeatedly generate at least one daughter equivalent to the mother cell with latent capacity for differentiation. This is the defining property of stem cells.
Stem cell: A cell that can continuously produce unaltered daughters and also has the ability to produce daughter cells that have different, more restricted properties.
Stem-cell homeostasis: Persistence of tissue stem-cell pool throughout life. Requires balancing symmetric self-renewal with differentiative divisions at the population level, or sustained asymmetric self-renewal.
Stemness: Unproven notion that different stem cells are regulated by common genes and mechanisms.
Transit-amplifying cell: Proliferative stem-cellprogeny fated for differentiation. Initially may not be committed and may retain self-renewal.