1 Introduction
Pesticides are occasionally used indiscriminately in large amounts, causing environmental pollution; they are therefore a cause of concern. The use of organophosphorus pesticides (OP) has increased considerably due to their low potency and durability as compared to organochlorine pesticides [1]. Residual amounts of (OP) pesticides have been detected in the soil, water bodies, vegetables, grains, and other foods products [2,3]. The mechanism of the toxicity of (OP) compounds consists mainly in blocking acetylcholinesterase, an enzyme that decomposes acetylcholine [4,5]. Immobilisation of this enzyme results in an accumulation of excessive amounts of acetylcholine in nervous tissue and muscular motor plates, as well as in symptoms of endogenic poisoning by this neurohormone. The OP compound malathion [S-1,2(bis ethoxycarbonyl) ethyl dimethyl phosphorodithioate] is used extensively throughout the world to control major arthropods in public health programs, animal ectoparasites, human head and body lice, household insects and to protect grain in storage [6]. Among the targets for malathion toxicity is the liver, which is the most important organ in glucose homeostasis and production of related enzymes [7].
It has been reported in several studies that hyperglycaemia is one of the side effects in poisoning by OP in acute treatment and in subchronic exposure via glycogenolysis and gluconeogenesis [1,8–11]. Nevertheless, Rezg et al. have noted for the first time an activation of glycogen storage and stimulation of enzymes of glycogenesis, accompanied with normoglycaemia in subchronic exposure to malation [12].
The main question of the present study was: what will happen to metabolic parameters in subchronic exposure to malathion to establish these antagonist reactions, glycogenesis and glycogenolysis associated with gluconeogenesis? In addition, the present investigation examines malation effects on some haematological parameters.
2 Materials and methods
2.1 Chemicals
Malathion (fyfanon 50 EC 500 g/l) of commercial grade was used in this study.
Acetylthiocholine iodide, 5,5-dithio bis(2-nitrobenzoic acid) (DTNB), trichloroacetic acid (TCA), KOH, ethanol, ether, Coomassie G250, bovine serum albumin, orthophosphoric acid 85%, chloroform, methanol, and NaCl were obtained from Sigma-Aldrich Co. (Germany).
2.2 Animals and treatments
Adult male Wistar rats (170–180 g), procured from the Tunisian Society of Pharmaceutical Industries, were kept in clean plastic cages and allowed to acclimatize in the laboratory environment for 7 days. Balanced food and drinking water were given to the animals ad libitum.
Animals were randomly divided into two groups of 12 animals and treated for 32 days. The first group received orally 1 ml of corn oil by force-feeding. The second one received 1 ml of corn oil containing 100 mg Mal/kg body weight per day. The experiment was performed in ethical conditions. At the end of the treatment, 24 h after the last dose of malathion, animals were killed by decapitation, without preliminary anaesthesia, and arteriovenous blood was taken quickly. The liver and the skeletal muscle were removed by transverse abdominal incision and kept frozen at −80 °C.
2.3 Acetylcholinesterase (AChE) activity assay
Acetylcholinesterase activity of plasma was estimated by the method of Ellman [13], using acetylthiocholine iodide as a substrate. The rate of hydrolysis of acetylthiocholine iodide is measured at 412 nm through the release of a thiol compound, which, when reacted with DTNB, produces the colour-forming compound TNB.
2.4 Haematological parameters
2.4.1 Haemoglobin concentration
Haemoglobin was measured through its transformation to cyanmethemoglobin, which is measured spectrophotometrically at 540 nm under the action of potassium ferricyanide and potassium cyanide [14].
2.4.2 Haematocrit content
This assay was done directly using a centrifugal machine to haematocrit.
2.5 Metabolic parameters
2.5.1 Plasma glucose assay
Glucose was measured by the glucose oxidase and peroxidase using quinoneimine as a chromogen. The amount of plasma glucose is related to the amount of quinoneimine, which is measured spectrophotometrically at 505 nm [15].
2.5.2 Hepatic and muscular glycogen assay
For the determination of glycogen, we followed the technique of Good [16]: 0.5 g of liver or muscle was extracted with 3 ml of 30% KOH, incubated for 30 min at 100 °C, cooled and brought to acid pH by addition of 20% trichloroacetic acid. Precipitated protein was removed by centrifugation for 10 min at . Glycogen was precipitated by ethanol and weighed. The results are expressed in g of glycogen per 100 g of liver.
2.5.3 Hepatic protein assay
The protein content in liver was quantified by the method of Bradford [17], using bovine serum albumin as a standard.
2.5.4 Hepatic lipid assay
The lipid content in liver was quantified by the method of Folch [18], using a mixture of chloroform and methanol for the extraction. Lipid was precipitated by ethanol and weighed. The results were expressed in g of glycogen per 100 g of liver.
2.6 Statistical analysis
All data are expressed as mean ± SEM by factorial ANOVA, followed by Student test. Differences between groups were considered significant when .
3 Results
3.1 Physical observation
No signs of toxicity were observed in malathion-treated animals until the end of experiment.
3.2 Acetylcholinesterase (AChE) activity and haematological parameters
Malation reduced significantly AChE activity. No significant change was observed in haematocrit values, in spite of the significant increase in haemoglobin concentration (Table 1).
Effect of subchronic administration of malathion on AChE activity, haematocrit value and haemoglobin concentration
Treatment | AChE (nmol/min/mg protein) | Haematocrit value (%) | Haemoglobin concentration (mmol/l) |
Control (n=12) | 12.20±0.79 | 42.28±0.76 | 1.64±0.13 |
Malathion (n=12) | 6.62±0.07⁎⁎⁎ | 42.03±0.63 | 2.00±0.09⁎ |
⁎ as compared with control.
⁎⁎⁎ as compared with control.
3.3 Effect of malathion on metabolic parameters
Malathion at dose of 100 mg/kg/day decreased significantly hepatic proteins and lipids contents and muscular glycogen rate, in spite of the significant increase in hepatic glycogen rate. Blood glucose concentration was not significantly different between groups (Table 2).
Effect of subchronic administration of malathion on plasma glucose concentration, hepatic and muscular glycogen rates and hepatic proteins and lipids' contents
Treatment | Blood glucose concentration (g/l) | Muscular glycogen rate ( of liver) | Hepatic glycogen rate ( of liver) | Hepatic proteins rate ( of liver) | Hepatic lipids rate ( of liver) |
Control (n=12) | 1.16±0.24 | 2.7 ± 0.10 | 3.91±0.34 | 4.20±0.21⁎ | 5.92±0.16 |
Malathion (n=12) | 1.20±015 | 1.88 ± 0.06⁎⁎⁎ | 5.88±0.43⁎⁎ | 3.60±0.09 | 4.65±0.18⁎⁎⁎ |
⁎ as compared with control.
⁎⁎ as compared with control.
⁎⁎⁎ as compared with control.
4 Discussion
4.1 Effect of malathion on haematological parameters
The results of this study indicate that malathion, in subchronic exposure at a dose of 100 mg/kg per day, decreases significantly acetylcholinesterase (AChE) activity on plasma, which is often taken as an indicator of (OPs) pesticide intoxication. In addition, our results showed a marked increase in haemoglobin concentration, which can be considered as an adaptive situation in order to guarantee a good oxygenation in response to pulmonary damage induced by subchronic exposure to malathion. Indeed, a rise in the haemoglobin content is generally among the signs of the pulmonary fibrosis [19,20], which several studies suggested to be among the injurious consequences of (OP) compound administration [21–23]. This explanatory assumption needs to be examined in more detail with appropriate biochemical and hormonal determinations. On the other hand, the haematocrits' count has not been changed significantly.
4.2 Effect of malathion on metabolic parameters
The results of this study indicate that malathion in subchronic exposure decreased significantly hepatic proteins and lipid contents. This reduction could be associated to liver gluconeogenesis. Indeed, Abdollahi et al. [11] have showed an increase in the hepatic gluconeogenic enzyme activity, phosphoenolpyruvate carboxykinase (PEPCK), in response to malathion exposure, which indicates a stimulated gluconeogenesis.
In addition, several studies indicate that malathion exposure causes hyperglycaemia, as an immediate consequence of malathion administration [8–10], which was explained by stimulation of glycogenolysis in several organs [1,10,11] and of gluconeogenesis in liver [11], provoking glucose release into the blood. This explained the significant decrease in hepatic proteins and lipid contents and the observed muscular glycogen rate.
However, it is interesting to note that hyperglycaemia is temporary [8,9]. Indeed, a time-dependent variation in blood glucose concentration was probably repeated daily, independently of the duration of the treatment (acute or chronic). Thus, malathion induced a gradual increase followed by a decrease in blood glucose, which can even reach hypoglycaemia [12]. On the other hand, it has been shown that acute exposure to malathion leads to the increase of glycogen deposition in liver during 6 to 24 h after malathion treatment [8]. It is well established that the increase in hepatic glycogen rate is due to the stimulation of key enzyme of glycogenesis (glycogen synthetase) [12].
One possible explanation for these results could be the turnover of glucose by its release through a succession of glycogenolysis and gluconeogenesis [1,11], which involves abnormal hyperglycaemia and its storage via glycogenesis [12] in subchronic exposure to malathion (Fig. 1).
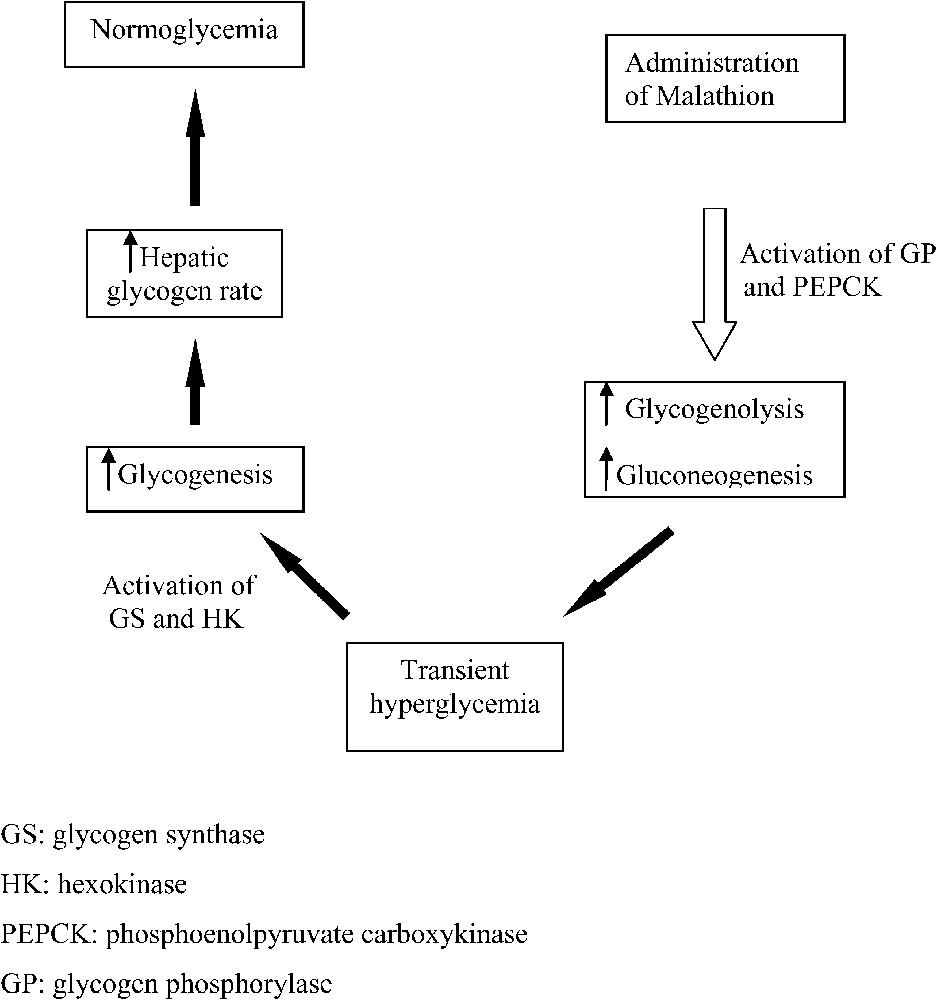
The mechanism of turnover of glucose in subchronic exposure to malathion: A time-dependent variation of different metabolic parameters.
Consequently, the divergence observed between studies may be due to the difference between the experimental protocols, especially the duration separating sampling and last administration of malathion. However, finding out the exact mechanism of glucose turnover in subchronic exposure needs appropriate sampling and molecular analysis. This explanatory assumption is currently under study by our team.