1 Introduction
Mars and the Earth differ in several aspects, including size, color, atmosphere, water, topography. However, their internal structures are very alike, as has been inferred from the mean density and the moment of inertia, complemented by meteorite analyses. The Martian crust is on average 50-km thick, as suggested by geoid and topography analyses [51]. Beneath this crust, there is a silicate mantle, between 1300 and 1800 km thick. At the center, there is an iron-rich, metallic core. The exact state of the core remains unknown, even if recent observations concluded that it has to be at least partially liquid [53].
The existence of an active magnetic dynamo on Mars was debated until 1996, when the NASA mission Mars Global Surveyor (MGS) was launched. It was designed to study the past and present geological and geophysical evolution of Mars [3]. Among others, it carried a MAGnetomer/Electron Reflectometry (MAG/ER) experiment. Prior to MGS, very few spacecrafts had carried magnetometers, and only Mariner 4 [43], and Mars 2, 3 and 5 [16] made some measurements, at large distances of the planet. Conclusions were poor about the existence of a dynamic (i.e. reversing) or fossil magnetic field.
MGS was inserted into orbit on 2 September 1997, on a very elliptical orbit. Its low periapsis made it possible to measure the magnetic field at very low altitude (as low as 90 km) for a short period of time. These measurements led to the conclusion that Mars lacks today a global, planetary scale, magnetic field [1]. The magnetic field of Mars is characterized by localized, sometimes very intense, magnetic field anomalies. The term ‘anomalies’ here refers to anomalous with respect to the global (zero) field of Mars, by analogy with the terrestrial lithospheric magnetic field anomalies. This came as a major surprise, as it was not envisaged that a remanent magnetic field could be that intense. MGS measured fields as large as 1500 nT at 90 km altitude. Such remanent magnetic fields are the lithospheric records of an ancient, now extinct, dynamic magnetic field, modified by several alteration processes (volcanoes, impact craters…). Any interpretation relies on a careful analysis of magnetic measurements, but also on the understanding of the different processes that affected the remanent magnetization during Mars’ history.
In the following, we discuss only the internal, lithospheric magnetic field of Mars. We first present global models derived from MGS magnetic measurements. We then focus on local anomalies, using different approaches. These local analyses allow some interesting properties of the ancient magnetic field of Mars to be investigated, which bring new and important constraints on Mars’ formation and evolution.
2 A global map of the Martian magnetic field
MGS remained in orbit around Mars between September 97 and November 2006. Two AeroBraking (AB1 and AB2) periods were separated by a six-month long Science Phase Orbit (SPO). Then MGS entered its Mapping Orbit (MO) cycles in March 1999, and flew during more than 34,000 orbits at an almost constant altitude of 400 km. MGS measured the three components of the magnetic field, Br (radial field, positive upward), Bθ (horizontal North-South, positive southward) Bφ (horizontal East-West, positive eastward), with an accuracy of the order of 1 nT for each component.
Measurements acquired at different altitude can be used to study correlations with geologic structures, or magnetization contrasts of the lithosphere. On the Earth, spherical harmonics models [23] are commonly employed. However, this method is very sensitive to incomplete data coverage [42]. In contrast, Equivalent Source Dipole (ESD) [32] approach is less sensitive to geographical data distribution. In addition, it gives insights on what could be the magnetization contrasts in the source region [33].
Using this method, Langlais et al. [24] produced a global map of the Martian magnetic field at a constant altitude. The model is based on 4840 sources, located below Mars’ surface onto an icosahedral (equi-surface) grid. The mean resolution of the model is 170 km, or 2.92° at the equator. There are no a priori constraints on the magnetization direction, because it is not aligned onto the core field. All three components of the magnetization are thus solved for. The magnetic field computed at a given location is the sum of all dipole contributions, within a distance of 1500 km to limit the size of the inverse problem. Measurements of the three MGS phases are used, but a local time selection is applied on SPO and MO datasets, in order to avoid the expected larger external magnetic field of the daytime. Measurements are averaged onto 1° × 1° × 10 km bins. Outliers are removed, by comparing measurements to an earlier model prediction [32]. Whenever possible, associated variance is computed and used during the iterative inversion. Final root mean square (rms) differences are of the order of 3 and 20 nT for each component for the MO and joint AB/SPO datasets, respectively.
The magnetic field is computed at a constant altitude of 200 km (Fig. 1) using this discrete model. The magnetic field is relatively weak, ±10 nT, almost everywhere North of the topographical dichotomy. The case for the southern hemisphere is very different. There are very intense magnetic fields, up to ±650 nT above Terra Cimmeria and Terra Sirenum. The largest volcanic provinces, Tharsis, Elysium, Olympus, are not associated with magnetic anomalies. The largest impact craters (Hellas, Argyre, Isidis) also lack magnetic signals.
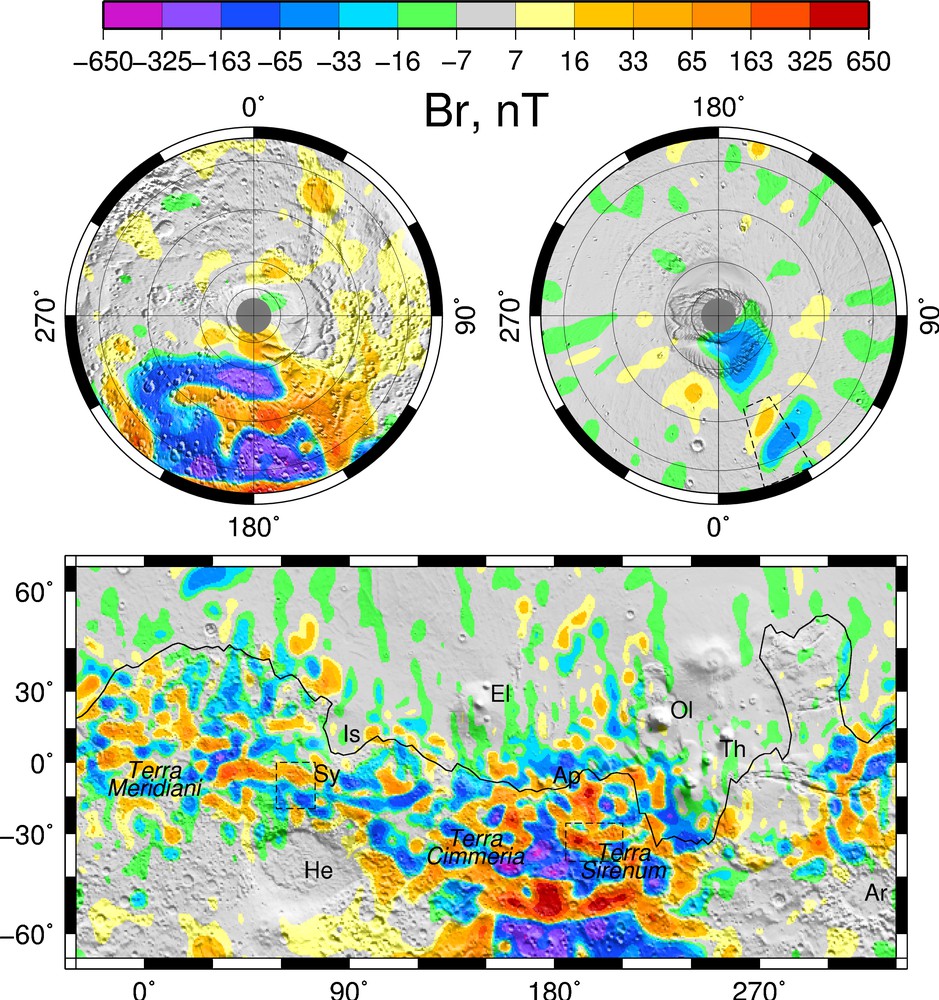
Magnetic field (radial component) predicted at 200-km altitude, from an ESD model of [24], superposed onto a shaded relief of Mars. Top left: South Pole up to 50°S; Top right: North Pole up to 50°N; bottom: between ±65° latitude. The crustal dichotomy is shown by the solid line. Abbreviations denote Hellas, Syrtis Major, Isidis, Elysium, Apollinaris Patera, Olympus Mons, Tharsis, and Argyre. The three surrounded areas correspond to the ones studied by [34,36].
Fig. 1. Composante radiale du champ magnétique prédit à une altitude constante de 200 km, basée sur un modèle de sources dipolaires équivalente [24], superposée sur le relief ombré de Mars. Coin supérieur gauche : pôle sud jusqu’à 50°S ; coin supérieur droit : pôle nord jusqu’à 50°N ; partie inférieure : entre ±65° de latitude. La dichotomie crustale est indiquée par la ligne noire. Les abréviations dénotent Hellas, Syrtis Major, Isidis, Elysium, Apollinaris Patera, Olympus Mons, Tharsis, et Argyre. Les trois zones entourées par les tirets correspondent aux trois zones étudiées par [34,36].
This implies that these destructive events took place after the Martian magnetic dynamo had shut down. Only a fraction of the lithosphere has kept its remanent magnetization, acquired while the dynamo was still active. The magnetic field in the early history of Mars could arise from two kinds of core dynamo generation: thermal or compositional [46]. The latter is related to the inner core solidification. The liquid becomes enriched in light elements close to the inner core, and consequently moves upward, creating convection. Assuming an initially hot core, with a 14% sulphur composition [10,11], the growth of the inner core would however not have started before 3 Gyr, which is not compatible with the magnetization of the ancient, older than 4 Gyr old units of the southern hemisphere. The early thermal dynamo hypothesis is thus more likely [10,11,19,52]. Furthermore, the thickening of the lithosphere would facilitate the dynamo shutdown, by turning the mantle convecting regime into a stagnant lid regime [10].
Lower altitude magnetic fields remain unknown. Downward continuations, based on spherical harmonics models [12] predict fields as large as 12,000 nT. This value has to be seen as a minimum one, since models do not take into account the short, possibly very energetic wavelengths of the magnetic field. Surface magnetic fields of lithospheric origin on Mars may be of similar amplitude to the Earth’s main magnetic field. Although nonunique, the magnetization model of [24] predicts magnetization as large as 12 A/m for a 40-km thick lithosphere. Such large values imply that the magnetic mineral content is higher on Mars, and/or that the ancient, dynamic magnetic field was more intense than it is on the Earth. In order to improve the resolution of this Martian magnetization global model, regional studies are needed.
3 Local studies
Remanent magnetic anomalies trace both how the ancient magnetic field was recorded in the rocks and the subsequent processes that locally modified the magnetization since the dynamo shutdown. These can be studied using forward or inverse approaches. In the first case, simple geometry magnetized bodies are used (spheres, surface disks, vertical prisms…) [7,48]. However, it is not possible to make an exhaustive exploration of the different parameters (magnetization and location). In addition, some parameters are too correlated with each other to allow a unique solution to be found. This approach is limited, as it implies simple geometry bodies, and isolated magnetic anomalies. In the following, two inverse approaches are described: a local inversion, using equivalent sources and a priori information, and a local version of the ESD approach.
3.1 Semi-inverse approach
The semi-inverse method [34] is a two-step approach. First, a raw, forward approach is used, based on homogeneously magnetized spheres. This first step gives some hints on the source characteristics (number of sources, approximated location and magnetization components). These are then used in the second step, as a priori knowledge of the solution in the inverse problem [47]. On synthetic datasets, the efficiency of the method was shown to be improved when the inversion is based on multialtitude datasets [35], such as the one MGS provided.
This method is applied on three distinct Martian areas [34], representative of the Martian magnetic anomalies (Fig. 1). The first area is located within the heavily cratered Terra Sirenum. Magnetic field anomalies are as intense as 1300 nT at 100 km altitude, and decrease to less than 100 nT at 400 km altitude. While the anomalies appear to be scattered at low altitude, they coalesce at high altitude. The second area is located between Hellas and Syrtis Major. This area is also cratered, but the magnetic fields are weaker, close to 400 nT at 100-km altitude. The last area is chosen in the northern hemisphere, within Vastitas Boralis, where an anomaly was clearly identified [20]. This anomaly is only 125 nT large at low altitude. It becomes very weak at high altitude, close to 10 nT.
The relationship between magnetic field anomalies and magnetized bodies is non unique. This is the reason why a priori information is required in this approach. A priori data errors, as well as parameter errors are also used. Large parameter errors allow a faster convergence through a solution, while small ones prevent the inversion scheme to converge to a solution. Models are a posteriori tested versus observations, by computing the associated chi-square and by looking at the distribution of the differences. If necessary, possible outliers are removed, and a priori parameter errors are modified in order to optimize these two quality criteria.
Data are first selected with respect to their altitude, which leads to two distinct datasets. Source parameters are then investigated using either one or the other or both datasets. Three magnetized bodies are needed for the first area. Models based on a single low- or high-altitude dataset fail to correctly predict high- or low- altitude measurements, respectively, but the use of both datasets strengthens the results. Despite the relative proximity of the three magnetic sources, they are associated with different magnetization parameters. Only one common feature is their intense magnetic moment, of the order of 2 × 1016A m2. Their depths range between 30 and 60 km. Assuming the magnetic sources to be tangent-to-the-surface spheres, their magnetization would be 54 and 32 A/m for the deepest ones, and up to 143 A/m for the shallowest one. Such large values are not met on the Earth on such large scales (> 105 km3). The magnetization directions also vary, from a steep (−50°) inclination, to a subhorizontal (∼20°) inclination for the last one. The three-body model predicts more than 57% of the raw signal. Correlation coefficients exceed 0.95 for Br and Bθ. Rms differences are of the order of 100 and 20 nT for the low- and the high-altitude datasets, respectively. Similar fits and correlation coefficients are obtained for the two other areas.
3.2 Equivalent sources
These relatively large residuals are due to the implicit simplicity of the model. Only three sources are used to predict magnetic field measurements over an area that is 1300 × 1000 km2 wide. In order to decrease the residuals, one can use more sources (thus increasing the complexity of the inversion scheme), or a localized version of the ESD approach. The latter was applied by [22], above Apollinaris Patera, a Martian shield volcano associated with a magnetic anomaly. This 200-km wide volcanic edifice is located at (174.4°E, 9.3°S). Crater counts showed that this edifice was completed 3.74 Gyr ago [50]. Its oldest visible surface is 3.98 Gyr old, but its initial activity may be older.
The volcano is associated with a strong 200 nT magnetic anomaly at 100-km altitude. The comparison with the few low-altitude and the numerous high-altitude data is essential, as it confirms the correlation between the magnetic anomaly and the volcanic edifice. This magnetic anomaly is studied using a localized ESD method. Since the dipoles have to be as equidistant as possible, it is not possible to choose the location of a particular dipole, but in counterpart, it is relatively easy to increase the number of sources, keeping in mind that the most remote ones will be affected by edge effects. Dipoles are placed on a hexagonal mesh, using up to seven concentric hexagons. The mean distance between adjacent sources is 1.9°, or 100 km, similar to the minimum altitude of the measurements. The optimal number of sources is a posteriori evaluated, by comparing rms differences between different configurations in a small area around the volcano.
A nonuniform magnetization case is studied, in which no constraints are imposed on the magnetization. Second, the directions of the magnetization are imposed, leading to the uniform magnetization case: a priori directions are those of a single dipole, located below the volcano. Rms residuals are as low as 3 nT for the MO data. The uniform and the nonuniform magnetization cases allow the coherency of the magnetization directions to be evaluated. The magnetization remains positive around the volcano for the uniform magnetization case (a negative magnetization would correspond to a magnetization acquired in a reversed magnetic field). The semi-inverse method was also used over this area to check the consistency of these results: a single source, located below the volcano, has similar magnetization directions, but the fit to the data is somehow degraded, due to the simplicity of the model.
Interestingly, the directions computed in the nonuniform magnetization model are very consistent: the seven closest sources are associated with paleopoles that cluster around (99.2°E, 87.8°S), with a corresponding α95 equal to 18°. On the Earth, typical dispersion associated with equatorial sources is of the order of 13° [29], which is comparable with this study.
4 Implications for early Mars
In this section, we discuss the previous modeling results in terms of constraints for early Mars’ evolution.
4.1 Polar wander
On the Earth, paleomagnetic studies rely on the basic assumption that the magnetic field was dipolar, axial and centered. The same assumption can be made for Mars. In addition, differences between rotation and paleomagnetic poles are to be interpreted as true polar wander, because no clues about plate tectonics (or plate movement) have been found on Mars.
Many studies with regards to magnetic paleopoles have been conducted on Mars ([4,9,17,20,21,45]; see also Fig. 2). Most of these studies were done using relatively simple forward approaches (surface disks, vertical prisms), on isolated or dipolar magnetic anomalies. While some did not find any paleopole clustering [17], some others observed that about half of the paleopoles cluster around the Tharsis bulge. Because some magnetic paleopoles were South and North, Boutin and Arkani-Hamed [9] concluded that the Martian paleodynamo also experienced field reversals. Sprenke [45] used a statistical approach to infer the most likely location of the Martian paleopole, assuming that no polar wander occurred before the dynamo cessation. Resulting paleopole is more likely located around (230°E, 50°N), very close to the Tharsis bulge.

Location of magnetic paleopoles as computed in several studies: () [4];(■) [9]; (♦) [17]; (Δ) [20]; (★) [22]; (▾) [34]; (▴) [36]; red contour denotes the most probable location area for the magnetic pole for [45]. North and South magnetic paleopoles are plotted indifferently so that they appear on the same Martian hemisphere. The map is centered over Alba Patera (250°E, 40°N).
Fig. 2. Position des paléopôles magnétiques calculés dans plusieurs études : () [4] ; (■) [9] ; (♦)[17] ; (Δ) [20] ; (★)[22] ; (▾) [34] ; (▴) [36] ; le contour rouge représente l’emplacement le plus probable pour [45]. Les paléopôles magnétiques Nord et Sud sont tracés indifféremment, de telle sorte qu’ils soient tous sur le même hémisphère martien. La carte est centrée sur Alba Patera, en (250°E, 40°N).
The three paleopoles associated with the three magnetized bodies in Terra Sirenum [36] are located in (310°E, 26°N), (299°E, 46°N) and (221°E, 44°N), respectively. The mean location would be close to (270°E, 40°N), slightly northeast of the Tharsis bulge. This may indicate that relatively close magnetized bodies may have different magnetization components, however consistent with a stable magnetic pole, close to the previously inferred locations. The two other studied areas lead to similar observations (see Fig. 2). These results confirm previous hypotheses: the magnetic paleopole was not located at the nowadays rotation pole at the time when the lithosphere became magnetic.
On the contrary, the paleopole deduced from the magnetic anomaly associated with Apollinaris Patera is very close to the rotation pole. Although this is a unique result, it may be seen as a robust one, as it is (so far) the only example of a magnetic anomaly associated with a geological structure. This new result brings a new constraint, as it means that polar wander took place before the dynamo shutdown. Moreover, this can also imply that the axis of rotation of Mars has been stable for about 4 Gyr, since Apollinaris Patera acquired its magnetization.
This new constraint has to be introduced in models of Mars’ evolution. Based on these results, we can propose a possible scenario for Mars’ evolution:
- • Mars forms and differentiates. A magnetic dynamo starts in the core, and the cooling lithosphere acquires a remanent magnetization;
- • the Tharsis volcanic complex starts to grow, at a medium- to high-latitude location; the dynamo is still active;
- • the Tharsis complex becomes larger, altering the mass distribution of Mars. This induces a polar reorientation. Tharsis apparently moves equatorward;
- • Tharsis is in its current location. Apollinaris Patera is emplaced;
- • the dynamo shuts down; Tharsis bulge finishes its construction; large impacts take place, modifying the magnetic properties of the lithosphere.
Of course, this appealing scenario would have to be confirmed, by looking for other correlations between geological structures and magnetic signatures. A possible alternative but possible scenario is one in which the rotation equilibrium of Mars is not altered by the growth of the Tharsis bulge solely, but also by the hemispheric dichotomy, which is older than Tharsis [38]. Definitive answer might come from absolute timing of the surface, or from a better knowledge of the lithospheric structure, possibly using seismometers [27].
4.2 Magnetic mineralogy and geological processes
Another interesting result comes from the very intense magnetization that is required by different models. Assuming a 50-km thick layer, the magnetization capable of producing the observed magnetic anomalies has to be larger than at least 4.76 A/m [31]. ESD models predict magnetization components ranging between ±12 A/m, for a 40-km thick layer [24]. Others suggested even larger values, up to 60 A/m [15].
Very intense magnetizations were computed by [36] that are not commonly met on the Earth. Such intensely magnetized bodies may however be found near the mid-ocean ridge. Fresh basalts indeed carry remanent magnetization up to 20 A/m [8]. But these basalts are rapidly altered, reducing the magnetization to ∼1 to 5 A/m. In addition, this magnetization is confined to a thin, ∼ half-a-kilometer layer.
Single-domain magnetite and pyrrhotite, as well as multidomain (> 15 μm) hematite and metallic iron [49] are the proposed candidates for the Martian magnetic minerals. Pyrrhotite is not a common mineral on the Earth, but it has been identified as the main magnetic carrier in numerous Martian meteorites [40]. However, pyrrhotite has a relatively low Curie temperature (320 °C), and as a consequence, the depth at which pyrrhotite would be magnetized is relatively limited. On the contrary, MD hematite has a high, 675 °C Curie temperature, which would be compatible with a thick magnetized layer. The relatively weak saturation magnetization (∼2 kA/m), and the usual (but see [28]) low coercivity, are drawbacks to MD hematite as a good candidate for the main magnetic carrier. In addition, while coarse grain hematite deposits were detected above Terra Meridiani [6,14], their origin is very likely of sedimentary origin [5], which again disfavors hematite. The other alternative is SD magnetite, which has a high Curie temperature (580 °C) and a large saturation magnetization (480 kA/m).
Magnetite is the most common magnetic mineral on the Earth, and is also present in Martian meteorites. It can form by oxyexsolution of titanomagnetite, which may occur in the parent magma if the oxygen fugacity is high enough (high temperature oxidation). It can also exsolve from iron-bearing silicates, such as plagioclase or pyroxene in plutonic rocks. Secondary magnetite can also form at relatively low temperature, resulting from serpentinization processes [30] (Fig. 3). For instance, an 8% hydration of a basaltic crust (enstatite) would lead to a 10% volume fraction of magnetite, which is large enough to explain the required magnetization values [36]. A relative abundance of water in a basaltic lithosphere, coupled with a vigorous mantle convection to produce small-scale hot plumes, are then key elements required by this scenario during the earliest stages of Mars’ history [44]. Other scenarios, based on more large-scale patterns, including a degree-one convection, can lead to the same results [37].

Schematic view of the deep serpentinization processes. A local warm plume heats the base of, and the lithosphere. The ultrabasic and/or basic rocks react with the crustal water: this may produce large amounts of magnetite, which is magnetized in the presence of global magnetic field due to a core dynamo (chemical remanent magnetization).
Fig. 3. Vue schématique des processus de serpentinisation. Un panache thermique réchauffe la lithosphère et sa base. Les roches mafiques et ultramafiques réagissent avec l’eau : cela produit de la magnétite en quantité, qui s’aimante en présence d’un champ magnétique global (aimantation rémanente chimique).
5 Conclusions – the case for lower altitude measurements
In this paper, we review some recent improvements of our understanding of Mars in terms of its magnetic field history. Despite the relatively high-altitude of the unique areographical coverage of Mars, it is possible to establish some properties of the magnetic field. It has a remanent origin. It is not homogeneously distributed over the whole surface of Mars. Lithospheric magnetization is one to two orders of magnitude larger than what is commonly observed on the Earth. This implies larger amounts of coherently magnetized magnetic minerals, as well as deep Curie isotherms. Such amounts may imply both primary and secondary magnetic minerals. The latter may relate to hydrothermal alteration, producing large amounts of magnetite.
Magnetization directions can also be investigated. A study over Apollinaris Patera showed a paleopole located near the rotation pole. Some of the previous studies on isolated magnetic anomalies indicated a possible location close to Tharsis bulge. This new result is very important, as it confirms the major polar wander that occurred on Mars. Moreover, it gives timing constraints on this polar wander: it occurred prior to the disappearance of the magnetic field.
Mars has currently no global, planetary scale, magnetic field, but it possessed one during its history. The large, intense magnetic anomalies discovered by MGS are of remanent origin. What is still measured today is a combination of very ancient magnetization processes, and younger demagnetization processes. The fact that Hellas and Argyre are not associated with magnetic fields argues for a dynamo shutdown prior to their emplacement, around 4.0 Gyr ago [18]. A more accurate timing for the dynamo will rely on (1) absolute age determination, and (2) more detailed characterization of the magnetization contrasts between impacted and nonimpacted areas. Such mapping relies strongly on the altitude at which the magnetic field is measured. Unfortunately, current measurements are too high to allow a proper characterization. New, lower altitude measurements, are eagerly awaited.
During the past years, magnetic measurements on board MGS have revolutionized the way Mars and other planets were understood. It was not thought that lithospheric magnetic field could be that intense. Interestingly, ancient measurements of the Hermean magnetic field by Mariner 10 have been reanalyzed. New models, based on large lithospheric fields were proposed [2]. There are many other puzzling questions on Mars’ magnetic field to which we cannot yet answer. These are the period of activity of the Martian dynamo; the depth of Curie isotherm at the time when magnetization was acquired; the nature and the abundance of the magnetic minerals; the lateral and vertical contrasts of the magnetization [39]. These questions would be addressed by a new mission, such as the proposed Mars Environment and Magnetic Orbiter (MEMO) [25,26]. Not only these new measurements will help to answer these questions, but they will also bring new constraints on the internal structure of Mars, its thermal evolution, as well as its atmospheric escape history and the past existence of liquid water at the surface of Mars [13], possibly leading to the appearance of life [41].
Acknowledgements
This work has benefited from the support of the INSU/CNES Programme National de Planétologie and from the European Community’s Improving Human Potential Program under contract RTN2-2001-00414/MAGE. We wish to thank two anonymous reviewers. Additional support was provided by Evan and Youven.