1 Introduction
Ocean–continent transitions (OCTs) at rifted continental margins represent the genetic and geographic link between the margin and the first oceanic crust. Most OCTs are thermally equilibrated, not tectonically active, and covered by thick sediments. Processes which were active at the time of continental breakup can therefore only be studied from indirect proxies such as reconstructed subsidence rates, or the seismic structure of the basement. The aim of this article is to review concepts developed from the study of active processes at mid-ocean ridges, which we think could be used to better explain and quantify aspects of the thermal, magmatic, hydrothermal, and tectonic evolution of OCTs.
We focus on so-called “magma-poor continental margins”, which are characterized by large domains of exhumed mantle and hyperextended crust. Until recently, most data and evidence for mantle exhumation at OCTs came either from the Iberia margin [12,172], or from the Alpine Tethys margins [41,52,89,91]. These places therefore became type localities for what was then viewed as an extreme, “amagmatic” end-member case of rifted margins. However, in the past 10 years, an increased number of surveys have shown that a hyperextended crust, which may be associated with exhumed mantle, is relatively common along the world's deep margins. It is documented at the Iberia–Newfoundland margins, where it is best studied [8,12,109,145,163], in the South Atlantic [35] and South Australian margins [47], in the Red Sea [33], and in the eastern Gulf of Aden [39]. Mantle exhumation also occurs at slow and ultraslow spreading ridges [17,43,73]. The main objective of this paper is to investigate to what extent the concepts which have been developed for these mid-ocean ridge settings can be used to understand mantle exhumation at magma-poor OCTs.
Our starting hypothesis is that processes at OCTs, and particularly mantle exhumation processes, should have a lot of similarities to mid-ocean ridge processes, and that differences most likely result from the “inheritance” of OCTs. By this we mean that, while all the characteristics of a mid-ocean ridge are the results of mid-ocean ridge processes alone, mantle exhumation and the onset of seafloor spreading at OCTs are stages in the evolution of a margin and are therefore also conditioned by the preexisting characteristics (inheritance) of this margin. We will thus review current concepts for slow and ultraslow spreading ridges, and discuss possible thermal, magmatic, hydrothermal, and tectonic similarities, or differences, between OCTs, and these slow and ultraslow spreading ridges, focusing on processes associated with mantle exhumation. This will lead us to address fundamental aspects of the evolution of magma-poor OCTs, such as the timing of the rift to drift transition, the distribution and extent of magmatism, the thermal regime and the evolution of topography, the source of early magnetic anomalies, and the extent of serpentinization.
2 OCT at magma-poor rifted margins
The discovery of exhumed mantle rocks along the Galicia margin, first by dredging, then by drilling during the ODP (Ocean Drilling Programme) Leg 103 [11,12,144] questioned the validity of the classical McKenzie type rift models [94]. In these classical models, the rifted continental crust is juxtaposed along a sharp boundary, against a three-layer oceanic crust. This initial discovery was followed by two decades of active research, including three additional ODP Legs (Legs 149, 173, and 210; [136,165,171]) and numerous geophysical surveys. Based on these results, the Iberia–Newfoundland rifted margin system can be subdivided (Fig. 1; [119]) into conjugate proximal domains, necking zones, and OCT domains, comprising conjugate zones of exhumed continental mantle (ZECM) which form the transition to oceanic crust. The proximal domains are characterized by a stretched crust showing “classical half graben type” sedimentary basins (e.g. Jeanne d’Arc [157]). The more distal domains show complex top-basement and intrabasement reflections, often interpreted as detachment faults, overlain by tilted blocks [90,132]. Crustal thinning from normal continental crustal thickness (± 30 km) to less than 10 km occurs in a “necking zone” which is less than 20 km wide [168]. At the South Atlantic and northwestern Australian margins, the hyperextended stretched continental crust, less than 10 km thick, extends over tens to hundreds of kilometers [35,72,107], with no seismic evidence for normal faulting.
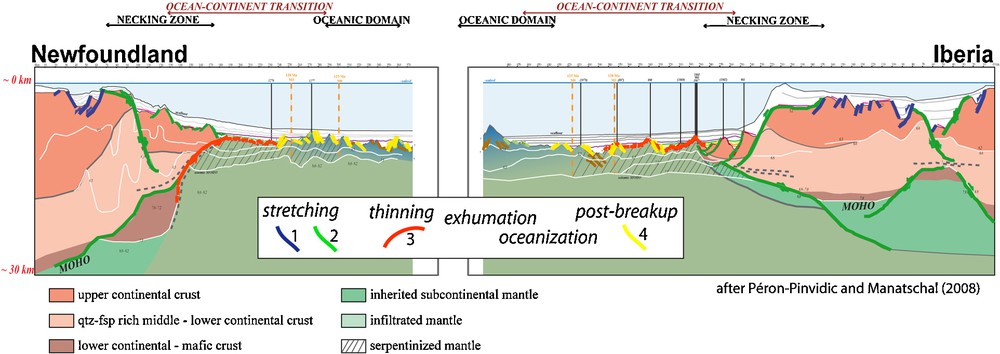
Geological interpretation of the Newfoundland–Iberia magma-poor rifted margins, based on reflection and refraction seismic and ODP drill hole data (modified after Péron-Pinvidic and Manatschal [119] and van Avendonk et al. [168]). This sketch identifies four successive phases of deformation. Mantle exhumation and the transition to true ocean spreading occur as part of the phase referred to as exhumation phase. Black lines: ODP drill sites. Orange dashed lines: magnetic lineaments. It is postulated that the OCT mantle was “infiltrated” by trapped melts on the Iberia side [109].
Fig. 1. Interprétation géologique des marges continentales pauvres en magma de Terre Neuve et de l’Ibérie, établie à partir de profils de sismique réfraction et réflexion et des données de sites de forage ODP (figure modifiée d’après Péron-Pinvidic et Manatschal [119] et van Avendonk et al. [168]). Ce schéma distingue quatre phases de déformation. L’exhumation du manteau et la transition vers une vraie accrétion océanique se produisent lors de la phase dite « exhumation phase ». Traits noirs : sites ODP. Traits pointillés orange : anomalies magnétiques. Le manteau sous la TOC côté Ibérie est figuré comme imprégné par du magma [109].
Further oceanwards, in the OCT, the continental crust tapers to zero and rocks derived from the subcontinental mantle are exposed. In the Iberia–Newfoundland rifted margin system, the Zones of Exhumed Continental Mantle (ZECM) extend over more than 100 km, as indicated by ODP drilling and geophysical surveys [172]. The crustal layer in the ZECM is characterized by velocity gradients different from those of classical continental or oceanic crusts [31,56,81,167]. Seismic velocities in the upper crust (VP: 4.5–7 km/s) are lower than those of adjacent continental crust, whereas seismic velocities in the lower crust (VP > 7.6 km/s) are too high to correspond to either the oceanic layer 3 or the underplated mafic continental crust [40]. The observed gradual transition to mantle velocities (Vp ≈ 8 km/s) could be explained by a progressive decrease in the serpentinization of mantle-derived peridotites [31], or by the occurrence of isolated magmatic intrusions into the mantle [133]. The mantle-derived rocks drilled in the ZECMs along the Iberia–Newfoundland margins range from strongly depleted serpentinized harzburgites (e.g. Newfoundland), to serpentinized lherzolites which have been enriched due to magma infiltration (Iberia [9,37,79,109]).
IODP drilling also revealed that exhumation of mantle-derived rocks in the ZECMs of the Iberia–Newfoundland margins did not occur in a strictly amagmatic environment, as was initially postulated [27,38,70,109]. Although there is no evidence for extrusive magmatic activity before breakup, these studies conclude that magma was present and reacted with the mantle rocks (infiltration) before they where exhumed at the seafloor. Further oceanwards, at ODP Sites 1070 and 1277, gabbros have been drilled and rare clasts of Mid-Ocean Ridge (MOR) type basalts have been found in Aptian debris flows (ODP Leg 149 [136]). Dating of the gabbros [70,137] showed that their ages are similar to those inferred for the magnetic anomalies recorded at the same locations, as would be expected for magmatic rocks emplaced during the accretion of new oceanic crust.
Drilling during ODP Leg 210 provided evidence for a widespread event of alkaline magmatism, which postdated continental breakup and probably lasted a few tens of millions of years [163]. Drilling at ODP Sites 1277 and 1276, respectively, sampled alkaline magmatic veins in basement [70], and sills in Aptian sediments [61], that can be mapped throughout the Newfoundland margin [121]. Thus, the magmatic history of the margin is complex and little is known about how magma was formed and distributed during final rifting, and about why magmatic activity continued in the OCT after the onset of seafloor spreading at the newly formed ridge.
The discovery of weak magnetic anomalies during deep-tow magnetic investigations of exhumed mantle-derived peridotite outcrops [154] indicates that magnetic anomalies are not restricted to volcanic terranes, but can also be recorded in areas where gabbros and serpentinized peridotites are found in the shallow basement [145]. Discussions still go on, however, about whether these anomalies may mark true, albeit magma-poor, seafloor spreading [154], or could have been created by magmatic intrusions within exhumed peridotites representing former subcontinental mantle lithosphere [133]. Another point of discussion is about the source of these anomalies, which could be magmatic, as proposed by Russell and Whitmarsh [133], or associated to serpentinization of the exhumed peridotites [145,154]. The timing of serpentinization and its interaction with magmatic activity during continental breakup is yet another pending issue [145].
Little is known about the thermal structure of OCTs during continental breakup. The subsidence history is commonly used as a proxy to determine this thermal structure. Drilling in the Iberia margin suggests that extreme thinning of the continental crust in the necking domain (Fig. 1) occurred under shallow marine conditions [119]. Paleowater depths in the OCT itself are poorly constrained. A common observation is, however, that the OCT appears deeper than the first unambiguously oceanic crust [120]. Whether this reflects colder thermal conditions at the time of breakup remains to be ascertained. The difficulty is that this thermal history is long past and poorly recorded at most margins, and that the thermal structure related to breakup has been equilibrated.
3 Slow and ultraslow spreading mid-ocean ridges
The fundamental difference between ridge spreading and rifting at divergent margins is that each increment of plate separation at a ridge creates new lithosphere, with no inheritance and with a thermal regime that is determined solely by the balance between axial heat supply and cooling. Current conceptual models of mid-ocean ridges emphasize the interactions between three fundamental processes, which are probably also key processes at OCTs: tectonics, magmatism, and hydrothermal circulation. The ridge axis lithosphere (thermal boundary layer) is where these processes take place, and its thickness and thermal structure are critical parameters. They control faulting, and the width of the tectonically active domain, through the thickness of the brittle layer [123,143], and they affect the ability for magma to pool at shallow depths [18], feeding eruptions and providing heat to hydrothermal convection cells [173].
Slow and ultraslow spreading ridges have a thick axial lithosphere (Fig. 2) which allows for the development of large offset normal faults, and of a deep (typically 500 to 2000 m deep) and wide (typically 5 to 25 km wide) axial valley [149]. Plate cooling and thickening with age away from the axial region (Fig. 2) also probably plays a significant role, keeping deformation and magmatism localized in the thinner lithosphere of the axial region. True ridge jumps (i.e. ridge relocation out of the former axial deformation zone) are excessively uncommon at slow spreading ridges, except in the vicinity of hotspots [104].

Flow line parallel section across the ultraslow spreading eastern Southwest Indian Ridge (section located on Fig. 6; all data from Cannat et al. [25]). Shown are, from top to bottom: principal magnetic anomalies, seafloor topography, predicted subsidence curve (dashed), base of crustal layer modelled from gravity data (dotted), and two isotherms (700 and 1200 °C) calculated with a 2D half-space cooling-with-age model. The 700 °C isotherm is inferred to correspond to the base of the brittle lithosphere [65], and the 1200 °C isotherm, to the top of the convecting asthenosphere. Note that the crust does not thicken beneath topographic highs indicating that these are dynamically supported tectonic features. Also shown: the inferred axial detachment fault (thick grey line), and inferred flow lines in asthenospheric mantle (dashed with arrows). The 1200 °C isotherm defines a narrow subaxial channel. This configuration could favor focused asthenospheric mantle upflow beneath the ridge, as proposed by White et al. [169].
Fig. 2. Coupe parallèle à la direction d’écartement des plaques à travers l’axe de la dorsale ultralente Sud-Ouest indienne (coupe localisée sur la Fig. 6; données de Cannat et al. [25]). Ce schéma montre, du haut vers le bas de la coupe: les principales anomalies magnétiques, la topographie du plancher océanique, la courbe de subsidence prédite (pointillés), la base de la croûte modélisée à partir des anomalies gravimétriques (pointillés courts), et deux isothermes (700 et 1200 °C), calculés avec un modèle de refroidissement avec l’âge en demi-espace. L’isotherme 700 °C est supposé correspondre à la base de la lithosphère fragile [65] et l’isotherme 1200 °C, au sommet du manteau convectif (asthénosphère). La croûte n’est pas épaissie sous les reliefs élevés. Ces reliefs sont donc des structures tectoniques non compensées. La ligne grise épaisse figure une faille de détachement axial et les flèches figurent les déplacements de matière supposés dans le manteau asthénosphérique. L’isotherme 1200 °C définit un canal asthénosphérique axial assez étroit. Cette configuration pourrait favoriser une focalisation du flux asthénosphérique sous les dorsales ultralentes, comme proposé par White et al. [169].
In this section, we first outline nine key concepts, or questions, regarding slow and ultraslow spreading ridges, which we view as most relevant to discuss OCT processes. We then focus our review on the eastern part of the Southwest Indian Ridge (SWIR). This region of the SWIR has a spreading rate of 14 mm/yr, comparable to that inferred from the earliest magnetic lineaments at the Iberia–Newfoundland margin [103,170]. It exposes large expanses of ultramafic seafloor (called “a-volcanic seafloor” on Fig. 6), over scales that are directly comparable to those of the well-documented Iberia–Grand Banks corridor (Fig. 6b).

(a): map of residual mantle Bouguer gravity anomalies in the easternmost SWIR (modified after Cannat et al. [25]), showing in black the contours of seafloor domains which have a volcanic upper carapace and more negative gravity anomalies (thicker oceanic crust), and in yellow the contours of corrugated seafloor domains. The remaining seafloor, covering about 50% of the mapped area, shows no evidence for volcanism and forms “smooth” ridges and troughs, corresponding with more positive gravity anomalies (thinner crust); (b): a similar-sized box (600 km across-axis and 500 km along-axis) in the Newfoundland–Iberia conjugate margin system, reconstructed at the time of breakup, and showing the Newfoundland Seamounts and Tore Madeire volcanic provinces in blue [145]; (c): shaded seafloor topography over a-volcanic, “smooth” Southwest Indian seafloor —insert on (a). Dredge (green diamond) recovered serpentinized peridotites with minor gabbros and basalts [141].
Fig. 6. (a) : carte des anomalies gravimétriques de Bouguer résiduelles réduites au manteau pour la région orientale de la dorsale Sud-Ouest indienne (d’après Cannat et al. [25]), montrant en noir les limites de domaines du fond qui ont une carapace volcanique et des anomalies gravimétriques plus négatives (croûte plus épaisse) et en jaune les limites de domaines du fond qui portent des cannelures et sont interprétés comme des surfaces exhumées d’anciens détachements axiaux. Le reste des fonds cartographiés, soit environ 50 % de la surface de la carte, ne montre ni morphologies volcaniques, ni cannelures. Ces fonds à morphologie lisse ont des anomalies gravimétriques positives (croûte mince) ; (b) : une boîte de taille comparable (600 km selon la direction de la divergence des plaques et 500 km perpendiculairement) dans le système de marges conjuguées Terre Neuve–Ibérie, reconstruit pour l’époque du début de l’océanisation [145]. Les Newfoundland Seamounts et la province volcanique de Tore Madeire sont en bleu ; (c) : bathymétrie ombrée d’un domaine à morphologie lisse et non volcanique près de la dorsale Sud-Ouest indienne – encart en (a). Une drague (losange vert) a permis d’échantillonner des péridotites serpentinisées, avec une petite quantité de basaltes et de gabbros [141].
3.1 The role of the axial lithosphere thickness for the exhumation of mantle-derived rocks
Most ridges receive enough magma to build a magmatic crust that is 6 km thick on average [29]. The depth to the base of the brittle lithosphere at slow spreading ridges is constrained by the maximum depth of earthquake's hypocenters [6,161,162,174]. It is commonly greater than 8 km at ridges slower than 3.5 cm/yr (Fig. 3). These slow spreading ridges therefore generally have an axial root of brittle mantle (Figs. 2 and 3). This configuration allows for the axial valley bounding faults to root into the subaxial mantle. It can therefore be viewed as a prerequisite for mantle exhumation. This is consistent with the distribution of most documented outcrops of mantle-derived peridotites along ridges with spreading rates lower than 3 to 4 cm/yr [17,43].

Lithosphere thickness at mid-ocean ridges as a function of the half-spreading rate (redrawn from Chen and Morgan [28]). At fast spreading ridges, lithosphere thickess is constrained by the depth of seismic reflectors and of seismic velocity anomalies which are interpreted as due to the presence of a melt lens in the crust (open boxes [42,106]). At slow spreading ridges, lithosphere thickness is constrained by the maximum depth of axial seismicity. Black dots are centroid depths from teleseismic studies [67]; stars are depths of microearthquakes at 23°N on the MAR [162]. Thermal models of the ridge axis (750 and 1100 °C isotherms) involve a component of hydrothermal cooling, which is tuned to fit these observational constraints [28]. The thickness of the magmatic crust calculated for a “normal” temperature mantle [13] is shown as a dashed grey line. At fast spreading rates, it corresponds to the average oceanic crustal thickness of 6 to 7 km [29]. It decreases at the slowest spreading rates. Mantle exhumation is common at ridges slower than about 3.5 cm/yr, where the magmatic crustal thickness is on average less than the axial brittle lithospheric thickness.
Fig. 3. Épaisseur de la lithosphère à l’axe des dorsales océaniques, en fonction du taux d’expansion (modifiée d’après Chen et Morgan [28]). L’épaisseur de la lithosphère aux dorsales rapides est déterminée à partir de la profondeur de réflecteurs sismiques et d’anomalies de vitesse sismique attribuées à la présence d’une lentille de magma [42,106]. L’épaisseur de la lithosphère aux dorsales lentes est déterminée à partir de la profondeur maximale des séismes. Points noirs : hypocentres déterminés à partir d’études télésismiques [67]; étoiles : hypocentres de microséismes enregistrés à 23°N sur la dorsale Médio-Atlantique [161]. Les modèles thermiques axiaux [28] sont ajustés pour reproduire la profondeur des isothermes correspondants (750 °C pour la transition fragile–ductile et la profondeur maximale des séismes et 1100 °C pour le solidus des magmas de type MORB). Cet ajustement joue sur l’intensité du refroidissement hydrothermal. L’épaisseur de la croûte magmatique produite par fusion du manteau sous la dorsale (trait pointillé gris épais) est calculée pour un manteau de température normale [13]. Cette épaisseur correspond, sauf pour les dorsales ultralentes, à l’épaisseur moyenne de la croûte océanique, soit 6 à 7 km [29]. L’exhumation du manteau est fréquente aux dorsales plus lentes que 3,5 cm/an environ, à l’axe desquelles la lithosphère fragile est en général plus épaisse que la croûte magmatique.
3.2 The thermal balance of mid-ocean ridges: heat supplied by magma and heat removed by hydrothermal circulation
Conductive thermal models have been developed that fit the seismic constraints on the lithospheric thickness of mid-ocean ridges (Fig. 3). These conductive models introduce magma as dikes or sills injected at crustal depths [28,62,123,147]. Hydrothermal heat advection is most commonly approximated by using enhanced thermal conductivity in a shallow domain near the axis [123]. These enhanced thermal conductivities are determined empirically so as to fit seismic constraints on axial lithospheric thickness (Fig. 3). They typically correspond to eight to 10 times the true thermal conductivity value for basaltic or ultramafic rocks [123]. This enhancement factor is comparable to estimates of the ratio of advected over conducted heat (or Nusselt number) at mid-ocean ridges, which have been made independently from off-axis heat flow measurements [155]. More realistic convective models of axial hydrothermal circulation yield higher Nusselt values [53], but hydrothermal cells at slow spreading ridges are probably transient in both time and in space [68,173]. The enhanced conductivity factor of conductive ridge thermal models can therefore be viewed as an equivalent to the Nusselt number of a time- and space-averaged axial hydrothermal system.
Magma is the principal carrier of heat to the axial lithosphere [147]. However, current ridge thermal models are significantly more sensitive to variations in spreading rate, than to variations in magma supply [30]. This can be understood because dikes or melt sills in these models are mostly emplaced and crystallized within the shallow domain of enhanced hydrothermal cooling, so that the heat they release is rapidly extracted. As will be discussed below, this needs not always to be the case, particularly at ultraslow spreading ridges and OCTs.
3.3 At what depth does magma crystallize in the axial lithosphere?
The thick axial lithosphere imposes constraints on the magma plumbing system of slow spreading ridges. One constraint is simply that temperatures in the lower crust on axis are too low for melts to form steady-state crustal magma chambers, as they do at fast ridges [42,113]. Instead, crustal magmatic bodies at slow spreading ridges should mostly form as intrusions within cooler host rocks [17]. Geological mapping in domains of exhumed lower crust and mantle-derived rocks at slow spreading ridges indicates that the resulting (intruding) gabbro bodies are up to a few kilometers in size [44,46]. This is consistent with observations made in Alpine and Apennine ophiolites [1,80].
The exhumed mantle at slow spreading ridges also contains a variety of millimeter- to decimeter-sized magmatic veins [19,21,75], as well as domains of melt-impregnated peridotite [142,156]. Melt-impregnation in the form of interstitial plagioclase, as observed in the mantle-crust transition zone of ophiolites [143], in Alpine ultramafic bodies such as Lanzo [110,124], and possibly also in samples drilled in the Iberian OCT [9,27,37,109], is rare in abyssal peridotites from slow spreading ridges. It occurs within centimeters, or decimeters, of gabbroic veins in otherwise plagioclase-free peridotites [21]. Outcrop-scale occurrences of plagioclase-bearing peridotites have been reported in transform faults, but are uncommon at ridges themselves [43]. Melt impregnation in the form of interstitial CPX, representing less than 2 % of the rock volume, is, however, common in peridotites from slow spreading ridges [21,140,142], and olivine-rich troctolites found at ODP Site 1309, Mid-Atlantic Ridge, have been interpreted as deriving from mantle peridotite after extensive melt–rock interactions [48].
While large gabbro bodies at slow spreading ridges are commonly assumed to have formed at crustal depths [36], the melt impregnated peridotites, as well as some of the smaller gabbroic intrusions, have crystallized in hot mantle rocks, probably within the lowermost, ductile part of the axial lithosphere [19,21]. Magma crystallization at subcrustal depths at slow spreading ridges is also postulated based on studies of mineral–melt and mineral–mineral partition coefficients in basalts [24,59,63,99], and in melt-impregnated peridotites [74–76]. These inferred higher pressure crystallization signals could, however, also be explained by melt–rock reactions within crustal gabbro bodies [85]. More work is therefore needed to determine the proportion of deeply-crystallized magmatic rocks at slow spreading ridges.
Another constraint imposed on the magma plumbing system of slow spreading ridges, with direct impact on the efficiency of magma transport and on the frequency of eruptions, is linked with the extensional deformation of the thick axial lithosphere. Behn et al. [7] have shown that dike injection plays a role in the formation of the axial valley topography, while flexure associated with faulting could inhibit further diking because it generates compression at the base of the brittle plate. This work provides a conceptual framework in which to envision a time evolution of the magma plumbing system at slow spreading ridges, depending on the tectonic state of the axis, and on the availability of fresh magma. This time evolution could comprise periods of rapid magma extraction through dikes, leading to eruptions on the seafloor, and periods of dike suppression, favoring magma crystallization at depth within the axial lithosphere. Understanding these tectonic and magmatic interplays and their timescales is one of the current frontiers for slow spreading ridge studies.
3.4 What is the proportion of magmatic rocks in the oceanic crust?
It is important to note that, because magma crystallization at slow spreading ridges occurs in a context of ongoing tectonic exhumation, even the end-member case of 100% subcrustal magma crystallization, would not correspond to a magma-free oceanic crust. Instead, the oceanic crust may comprise mantle-derived rocks with multiple generations of magmatic intrusions, formed at progressively shallower depths during tectonic exhumation [19,21]. It can be predicted that if the deep magma bodies form over a region that is wider than the region over which exhumation operates, a significant proportion of the deeply-crystallized magmatic rocks will remain in the mantle (Fig. 4). If, by contrast, the ridge's magma plumbing system is narrow relative to the width of the tectonized domain, most deeply-crystallized magmatic rocks will be tectonically emplaced in the oceanic crust [24].
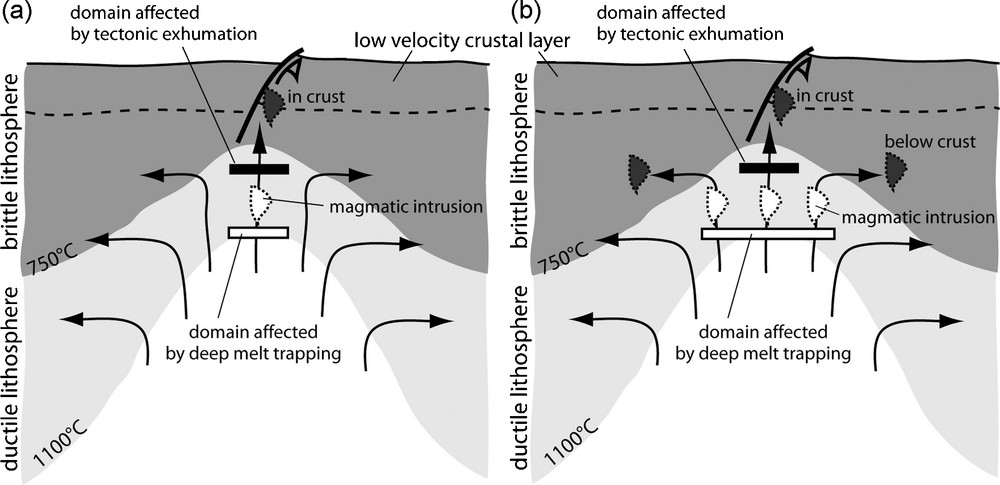
Schematic sections across a slow spreading ridge axis (redrawn from Figure 10 in Cannat et al. [24]). Brittle and ductile lithosphere are inferred to be limited by isotherms, as on Figs. 2 and 3. Arrows show hypothetical flow lines for solid material. Some of the melt delivered from the mantle is trapped and crystallizes into the axial lithospheric mantle (white for crystallizing mush, dark grey for gabbros or melt-impregnated peridotites). On (a), deep melt trapping operates over the same width as tectonic exhumation. All of the magmatic rocks crystallized from deeply trapped melts are therefore tectonically incorporated into the oceanic crust, together with their ultramafic host rock. On (b), deep melt trapping operates over a domain that is wider than the domain affected by tectonic exhumation. Gabbros (and melt-impregnated peridotites) which crystallized in the more external deep melt bodies are therefore incorporated into the upper lithospheric mantle and not into the crust.
Fig. 4. Coupes schématiques d’une dorsale lente (d’après la figure 10 de Cannat et al. [24]). Les niveaux fragiles et ductiles de la lithosphère sont limités par des isothermes, comme sur les Fig. 2 et 3. Des flèches montrent le déplacement très simplifié du matériel solide, à cause de l’écartement des plaques. Une partie du magma issu du manteau est piégée et cristallise dans le manteau lithosphérique (en blanc le magma, en gris foncé les gabbros ou les péridotites imprégnées par des gabbros). En (a), le magma est piégé dans un domaine aussi large que celui sur lequel opère l’exhumation tectonique. Toutes les roches magmatiques qui ont cristallisé en profondeur sont alors exhumées et incorporées tectoniquement à la croûte océanique, avec leur encaissant ultrabasique. En (b), le magma est piégé dans un domaine plus large que celui sur lequel opère l’exhumation tectonique. Les gabbros (et les imprégnations magmatiques) qui ont cristallisé en profondeur dans les domaines les plus externes sont donc incorporés au manteau lithosphérique, et pas à la croûte.
While seismic refraction studies could detect large (> 1 km) gabbroic bodies in the uppermost oceanic mantle, they will most likely miss smaller intrusions, unless they represent more than 10% of the overall volume of rock. This can be verified using an average Vp velocity of 7.1 km/s for gabbros [101], 8 km/s for peridotite [32], and a 7.9 km/s overall velocity for the uppermost mantle. The thick mantle lithosphere of slow and ultraslow spreading ridges (Figs. 2 and 3) may thus store significant volumes of magmatic rocks [18,148]. A few seismic studies of slow-spread lithosphere have also indicated anomalously low upper mantle velocities (7.5 to 7.8 km/s), which have been interpreted as due to partial serpentinization [71,102], but may also be at least partially due to trapped magmatic rocks [86].
3.5 What controls the ridge's magma supply?
The uprising asthenospheric mantle below the ridge (Fig. 2) is a key, yet invisible actor: its dynamics, temperature and composition determine the quantity of magma that is supplied to the ridge. When expressed per increment of plate separation and per increment of ridge length, this quantity has the dimensions of a melt “thickness” and is commonly used to describe the ridge's magma supply (Fig. 5). Magma supply is linked to mantle temperature because of the pressure dependence of peridotite solidus temperatures [77,95,96]. Magma supply is also linked to mantle composition: the more primitive and volatile-rich the mantle, the more melt it produces [4]. Alternatively, low melt supply in ridge regions such as the 13°N region of the Mid-Atlantic Ridge, and the easternmost portion of the SWIR, has been interpreted as due to remelting of a previously depleted asthenospheric mantle [98,141,142].

Melt thickness (i.e. magma supply; see text for further explanations) calculated for mid-ocean ridges as a function of (a) the full spreading rate for a “normal” mantle potential temperature (i.e. corresponding to the production of enough magma to build a 6 km thick oceanic crust), and (b) the regional axial depth (i.e. the axial depth averaged over a ridge length above 100 km and inferred to represent isostatic depth [77]). (a) is modified from Minshull et al. [103]. Melt thickness is calculated for focused (black curve [13]), and for unfocused (grey and dashed curves [118,130]) asthenospheric corner flow beneath the ridge. Unfocused flow may prevail at and near OCTs [103,118]. Focused asthenospheric corner flow provides a better fit to crustal thicknesses measured at slow and ultraslow spreading ridges [169]. At a spreading rate of 1.4 cm/yr, the rift to drift transition may thus result in a more than two-fold increase in melt production. (b) is modified from Cannat et al. [26]. Melt thickness is calculated for a dry peridotite solidus [64] and for the focused asthenospheric flow configuration of Bown and White [13]. Black dots: calculated melt thickness for a range of mantle potential temperatures at a spreading rate of 1.4 cm/yr. At a given mantle potential temperature, slower spreading rates result in a decrease in melt thickness. Crosses show this predicted decrease calculated for “normal” mantle potential temperature (1330 °C in this particular melting model). Unfocused asthenospheric corner flow corresponds to a more pronounced calculated decrease, but the relationship between regional axial depth and melt thickness remains the same.
Fig. 5. Épaisseur de magma (i.e. alimentation magmatique ; voir texte pour explication), calculée pour les dorsales océaniques en fonction (a) du taux d’expansion pour un manteau de température potentielle « normale » (i.e. correspondant à la production d’assez de magma pour construire une croûte de 6 km d’épaisseur) et (b) de la profondeur régionale de l’axe de la dorsale (i.e. la profondeur moyenne de l’axe sur plus de 100 km, supposée représenter une profondeur isostatique [77]). (a) est modifiée d’après Minshull et al. [103]. L’épaisseur de magma est calculée pour un flux asthénosphérique de type « corner flow », focalisé (trait noir [13]) ou non focalisé (trait gris pointillé [118,130]). Un flux non focalisé pourrait prévaloir dans les TOC [103,118]. Un flux focalisé permet de mieux reproduire les épaisseurs de croûte des dorsales lentes et ultralentes [169]. Pour un taux d’expansion de 1,4 cm/an, le passage du rifting à l’océanisation pourrait donc s’accompagner d’un doublement de la production de magma. (b) est modifiée d’après Cannat et al. [26]. L’épaisseur de magma est calculée pour un solidus de péridotite sèche [64] et pour un flux asthénosphérique focalisé (même configuration que pour Brown et White [13]). Points noirs: épaisseur de magma calculée pour une gamme de températures du manteau et pour un taux d’expansion de 1,4 cm/an. Pour chaque température, une baisse du taux d’expansion se traduit par une diminution de l’épaisseur de magma produite. Les croix montrent cette diminution, calculée pour un manteau de température potentielle normale (1330 °C dans le modèle de fusion utilisé). Un flux asthénosphérique non focalisé entraînerait une diminution plus prononcée, mais la relation calculée entre épaisseur de magma et profondeur régionale de l’axe serait la même.
Melt extraction patterns, and the dynamics of flow in the uprising mantle below the ridge are also important factors in determining the ridge's melt supply. Faster, active upwelling produces more melt, at all spreading rates, than passive corner flow-type upwelling. At slow spreading rates, mantle upwelling velocities may be so slow that the mantle cools from above and stops melting at great depths [130]. Because of this nonadiabatic cooling effect, models which assume a simple corner flow upwelling geometry predict a pronounced decrease of magma supply at spreading rates lower than 4 cm/year (Fig. 5a [130]). However, as pointed out by White et al. [169], this strong sensitivity to spreading rate does not fit seismic determinations of oceanic crustal thickness, which remain close to the 6 km worldwide average [30] down to spreading rates of 2 cm/yr. These observed crustal thicknesses fit better with the focused corner flow model of Bown and White ([13]; Fig. 5a), in which mantle upflow at slow spreading ridges is focused in a narrow axial channel, due to rapid cooling of the new lithosphere off-axis (Fig. 2). Going from unfocused to focused upwelling at slow spreading ridges, or at slowly rifted OCTs [103] could therefore result in a more than two-fold increase in magma supply (Fig. 5a).
3.6 Regional variations of ridge depth (bathymetry) as a proxy for magma supply
Mantle temperature, mantle composition, and magma supply can be predicted to have an effect on ridge depth, averaged over long portions of the axis (> 100 km), and inferred to represent isostatic equilibrium [77]. Colder mantle is more dense, and produces less magma as it rises beneath the ridge, resulting in a thinner, low density crustal layer, and in greater isostatic seafloor depths. The order of magnitude predicted for this effect is shown on Fig. 5b: to a decrease of ≈ 20 °C in the temperature of the asthenospheric subaxial mantle, should correspond ≈ 1 km less melt, and a deepening of the axis by ≈ 0.5 km. The role of mantle compositional variations is more complex, because depleted mantle produces less melt, but is more buoyant than fertile mantle [152]. Fast spreading ridges typically lie at depths ca 2700 m, corresponding to the predicted isostatic values for a 6 km thick crust and a “normal” temperature mantle [77]. The effect of axial valley tectonics adds 200 to 400 m to this isostatic reference value at slow spreading ridges [149].
3.7 Local variations of ridge depth, axial segmentation and fault topography
Fast spreading ridges have an essentially 2D structure, with little along-axis variations in axial depth, crustal thickness, or crustal seismic structure. Slow spreading ridges are, by contrast, markedly 3D. Axial depth typically varies by 2000 m, around the regional isostatic value, over distances of 30 to 100 km [138]. Larger axial depth variations, over longer distances, have been reported at some ultraslow spreading ridges [34,97,115]. These deviations from the regional average are associated with the segmentation of the ridge by axial discontinuities. Large transform faults, with a typical spacing of 300–400 km, are present at both fast and slow spreading ridges. Smaller transform discontinuities, with offsets of 50 km or less, are found only at slow spreading ridges, and can migrate along-axis [57]. Slow spreading segment ends correspond to a crust that is thinner than the regional average, while segment centers generally have a thicker crust, indicative of a higher magma supply [84,160]. Although this has not been systematically confirmed by seismicity studies [6,174], thermal models suggest that the lithosphere at the magma-poor segment ends is thicker than at the more magmatically robust segment centers [122]. The volcanic system at slow spreading ridges is also segmented, with volcanic morphologies indicative of significant along-axis magma redistribution in the crust, via blade-like dikes fed from the segment center [150].
Slow spreading segment ends, independent of the offset of the discontinuity [10], correspond to deep and wide axial valleys, while segment centers generally have a shallower and narrower axial valley [138]. These segment-scale variations have been shown to result primarily from along-axis variations in the amplitude of the tectonically-induced topography, possibly due to along-axis variations in the strength of the axial lithosphere [112,143]. The largest topographic effect occurs near segment ends, where the axial valley wall that abuts the discontinuity (also called the inside corner massif [139]), typically reaches depths less than 2000 m below sealevel, while the axial valley bottoms out at depths more than 4000 m. A significant part of this fault topography is preserved in off-axis regions, in the form of abyssal hills [58]. It is important to note that the amplitude of the fault topography developed at slow spreading ridges, over distances of only a few tens of kilometers, commonly exceeds the full global range of regional isostatic seafloor depth variations.
3.8 What is the cause of axial segmentation?
There are currently two interpretations for the origin of the segmentation at slow spreading ridges. In one interpretation [84,126], it is inferred to be due to segmented upwelling (diapirs) in the asthenospheric mantle below the ridge, feeding more magma to segment centers. In this interpretation, ridge segments are therefore anchored to individual asthenospheric mantle diapirs, and the size and spacing of these diapirs require very low upper mantle viscosities, which may be due to the presence of interstitial melt in the uprising asthenospheric mantle [127,128]. In the other interpretation [88], segmentation is inferred to result from the along-axis migration of magma in a permeable horizon which is proposed to exist at the base of the lithosphere [153]. This inferred permeable horizon is deeper in colder regions of the axis (near ridge discontinuities on-axis, and beneath older lithosphere off-axis). The resulting slope is proposed to trigger 3D gravity-driven magma migration, toward the ridge [153], and toward the segment centers [88]. Contrary to the mantle diapirs hypothesis, this magma migration hypothesis does not provide an explanation for the initiation of ridge segmentation. However, magma migration would help stabilize the ridge segmentation pattern, acting as a feedback to whatever process (including mantle diapirs) caused an initial thermal perturbation, by redistributing magma and heat from colder to hotter regions of the axis.
3.9 The context and extent of serpentinization
Serpentinization reduces the density and the seismic velocity of ultramafic rocks [32,101], and helps localize strain, due to the low frictional coefficient of serpentine [50,129,131]. It also produces magnetite that can record a remanent magnetization [116,159]. Studies of ultramafic samples from slow spreading ridges show that their serpentinization occurred mostly at relatively high temperatures: 200 to 350 °C [54], corresponding to the optimum temperature range determined experimentally for serpentinization reactions [92].
Domains of exhumed mantle at slow spreading ridges have crustal thicknesses less than 3 to 4 km [20,102], and these crustal thicknesses are not seen to increase off-axis. This indicates that very little if any serpentinization occurs at greater depth, and virtually none off-axis, except next to transform faults [102]. Numerical models for simplified ridge settings have recently shown that serpentinization rapidly shuts down, unless high permeabilities are maintained or frequently reactivated by tectonic extension [49]. This is due to the fact that serpentinization consumes large volumes of water and results in a substantial volume increase [78,114]. It has been proposed, in the context of OCTs [145], that such large and reactivated permeabilities could be due to bending and extension at the rolling-hinge of the detachment faults that accommodate mantle exhumation. Recently published models of serpentinization at mid-ocean ridges also link it with axial detachment faults [3,93].
Recent studies at the Mid-Atlantic Ridge have also demonstrated that a substantial part of the serpentinization there is linked with high temperature, black smoker-type hydrothermal cells, which are primarily fuelled by magmatic heat [2,87]. This has two important implications: one is that at least part of the serpentinization at mid-ocean ridges is associated to magmatic injections; the other is that this magma-associated serpentinization occurs in a context of vigourous hydrothermal convection and is therefore controlled by thermal gradients that cannot be approximated using conductive thermal models.
3.10 Mantle exhumation, suppression of volcanism, and magnetic anomalies at the magma-poor, ultraslow eastern SWIR
Some sections of the SWIR, and of the Gakkel Ridge in the Arctic are among the deepest mid-ocean ridge regions on Earth, with average axial depths above 4500 m, and an average crustal thickness of only 3 to 4 km [22,71,100,108]. These ridge regions are inferred to represent a magma-poor end-member for the ridge system worldwide. They expose ultramafic rocks for tens of kilometers along-axis and on both ridge flanks [25,45,100]. These characteristics differ from those of more magmatic slow and ultraslow spreading ridges, where ultramafics are generally exposed only within 20 km of axial discontinuities [20], and are intruded by kilometre-sized gabbro bodies [46,69]. Instead, magma-poor ultraslow spreading ridge regions recall the setting inferred for the Newfoundland-Iberian conjugate margins during the mantle exhumation phase [82,119,145], and are therefore the best candidates for our purpose of using present-day ridges to better understand past mantle exhumation at OCTs. Now we shall focus on three issues: the tectonic context of mantle exhumation, magma distribution and the local suppression of volcanism, and the origin of magnetic anomalies in domains of exhumed mantle.
3.10.1 Tectonic context of mantle exhumation
The SWIR east of the Melville Fracture Zone (Fig. 6) has been mapped up to seafloor older than magnetic anomaly 8 (26.5 Ma [15]), revealing large expanses of seafloor exposing serpentinized mantle-derived peridotites, with minor gabbros and basalts [141], and no evidence for a volcanic upper crustal layer [25]. This a-volcanic seafloor has been called a “smooth seafloor” because it occurs in the form of broad ridges, with a smooth and rounded topography. Smooth seafloor domains near the axis (Fig. 6c) typically lie at depths of 3500 m (crest of broad ridges) to 4500 m (troughs between broad ridges). They deepen off-axis following a standard cooling with age subsidence curve (Fig. 2). Smooth seafloor represents 50% of the seafloor on Fig. 6a. It formed simultaneously in both diverging plates, by repeated shifts in the polarity of axial detachment faults, leading to fault capture, over time scales of 1 to 2 million years [25]. Successive master faults extended 20 to 50 km along-axis, creating the characteristic ridge and trough topography of smooth seafloor domains, with an amplitude of 500 to 1000 m, and a wavelength of 10–15 km in the direction perpendicular to the ridge (Fig. 6c). Mantle exhumation in the eastern SWIR is, therefore, not found to be primarily associated with very long-lasting detachments, as inferred in most current OCT models [82]. The ridge and trough morphology in smooth seafloor domains compares well with the topography produced in thermomechanical models of axial faulting, for high proportions of tectonic versus magmatic extension [83,166].
Mantle exhumation in the eastern SWIR is also not found to be primarily associated with “corrugated” surfaces. These characteristic features, which have first been identified at the faster spreading Mid-Atlantic Ridge [16,166], are interpreted as the exhumed footwalls of large offset axial detachment faults. In the eastern SWIR, they represent only 4% of the total mapped area and appear to have formed in conditions of slightly enhanced magma supply, compared to smooth seafloor [25]. In the Atlantic, they also form a few percent of the seafloor [151,152,164], and are commonly associated with extensive outcrops of gabbro [46,69].
3.10.2 Melt distribution and the local suppression of volcanism
A first order characteristic of accretion in the magma-poor eastern SWIR is that magma supply to the ridge axis is highly segmented, over scales of 100 to 300 km [23,108,134]. The gravity map shows irregularly spaced gravity low bull's eyes (Fig. 6a), which correspond to thicker crust, and to seafloor with clear volcanic morphologies. These gravity low bull's eyes correspond to active (on-axis), and fossil (off-axis) volcanic centers [23,26]. Volcanic terrains extend out of these gravity low bull's eyes, and are postulated to form by along-axis dike propagation [22]. Magma distribution at the eastern SWIR therefore appears to involve both focusing of the magma supply to volcanic centers, and dike-controlled along-axis magma redistribution at crustal level. This is comparable to the magma distribution pattern inferred for less magma-poor slow spreading ridges (cf. Section 3.6), but with nearly an order of magnitude difference in the scale of the magmatic segmentation (100–300 km against 30–100 km), and with effective suppression of volcanism in the ends of the long magmatic segments. The origin of this magmatic segmentation remains an open question (mantle diapirs, along-axis migration of melts, or other processes to be determined; cf. Section 3.6).
3.10.3 The origin of magnetic anomalies in domains of exhumed mantle
The conventional understanding of seafloor magnetic anomalies is that their source mainly resides in an upper crustal layer of effusive volcanics [60]. Smooth seafloor domains of the eastern SWIR, which lie out of the reach of most dikes, lack such an effusive layer. Yet, these smooth domains do record magnetic anomalies, which can be followed into nearby volcanic domains and are demonstrably related to magnetic inversions [135]. Magnetic anomalies in smooth seafloor domains have, however, variable amplitudes, and are generally less defined than in adjacent volcanic domains, indicating lesser magnetization contrasts between adjacent blocks of opposite polarity [135].
Both serpentinized peridotites and gabbros are capable of carrying a remanent magnetization, and have been inferred to contribute to magnetic anomalies observed over domains of exhumed mantle at other slow and ultraslow spreading ridges [66,117,125,135,158], and at OCTs [133]. Indeed, combining two sources in variable proportions is a way to account for the variability of the magnetic signature of these exhumed mantle domains. Significant variability could, however, also be produced by serpentinized peridotites alone: their magnetic properties vary depending on their degree of serpentinization, but also, for a given degree of serpentinization, as a function of the chemistry of serpentine phases [5,116,159]. In contrast to basalts and gabbros, serpentinized peridotites can also carry a significant component of induced magnetization [111,116]. Shifts to more positive crustal magnetization values observed in the magnetic record of mid-ocean ridge exhumed mantle domains have been interpreted as due to this induced component [66,117,125,135,158].
4 Discussion: constraining the conditions of continental breakup using the concepts developed at slow spreading ridges
4.1 The rift to drift transition
In classical models of rifted margins, the onset of ridge spreading was defined as the time of formation of the first basaltic oceanic crust. Evidence for widespread mantle exhumation at present-day mid-ocean ridges has, however, questioned this definition. Alternative models have proposed the formation of early magnetic anomalies, which may be due to serpentinization of the exhumed mantle-derived component of the crust, as the criteria for initial ridge spreading at melt-poor OCTs [145,154]. Magnetic anomalies recorded in OCTs could, however, also be produced by discrete magmatic intrusions in exhumed subcontinental lithospheric mantle [133].
Our review of mid-ocean ridge characteristics leads us to take a different perspective, focusing on what we see as the fundamental definition of oceanic accretion, which is that each increment of plate separation creates new lithosphere, with no inheritance and with a thermal regime that is determined solely by the active balance of axial heat supply and cooling. By contrast, the thermal regime and the lithosphere at OCTs are, at least in their early stages of development, partly inherited from earlier stages in the evolution of the margin (Fig. 7). We propose to define the onset of spreading as the time when the OCT reaches the state of active thermal equilibrium, which characterizes oceanic ridges (stage 2 on Fig. 7).
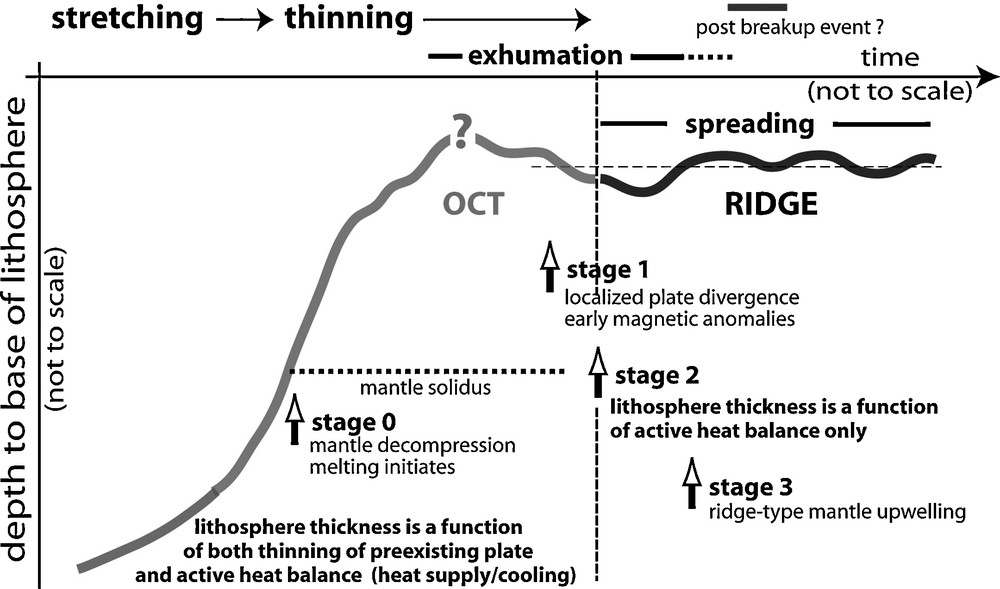
Interpretation of the thermal structure of the rift to drift evolution at a magma-poor rifted margin system. The base of the ductile lithosphere is inferred to be limited by an isotherm, as on Figs. 2–4. In this sketch we postulate that true ocean spreading begins (stage 2) when the thermal regime in the zone of plate divergence results only from the active balance of heat supply (primarily from melts), and cooling (hydrothermal and conductive). This requires that plate divergence has already become durably localized. Early magnetic anomalies may record this initial stage of localization (stage 1). In a third stage (stage 3), we postulate that the upwelling pattern in the asthenosphere settles to its ridge-type, focused configuration, resulting in a substantial increase in melt supply (Fig. 5a), which could locally and/or episodically favor the cessation of mantle exhumation and the formation of a thicker, fully magmatic oceanic crust. Stage 0 in this proposed evolution corresponds to the initiation of rift-related mantle melting, when plate divergence has thinned the lithosphere so that its base is shallower than the solidus of the underlying asthenospheric mantle.
Fig. 7. Schéma interprétatif de la structure thermique pendant l’évolution du rifting à l’océanisation, dans une marge continentale peu magmatique. La base de la lithosphère ductile est supposée correspondre à un isotherme, comme sur les Fig. 2–4. Nous proposons que l’accrétion océanique démarre (étape 2) quand le régime thermique dans la zone de divergence des plaques ne dépend plus que de l’équilibre actif entre l’apport de chaleur (principalement magmatique), et le refroidissement (hydrothermal et conductif). Cela suppose que la divergence s’est localisée de façon durable. Une telle localisation pourrait produire les premières anomalies magnétiques (étape 1). Dans une troisième étape (étape 3), il est possible que le flux asthénosphérique, initialement peu focalisé, évolue vers une configuration plus focalisée, associée à une production magmatique accrue (Fig. 5a). Cette plus grande production magmatique pourrait entraîner, au moins localement et/ou de façon épisodique, l’interruption de l’exhumation du manteau et la formation d’une croûte océanique plus épaisse et de nature magmatique. L’étape 0 dans ce schéma correspond à l’initiation de la fusion du manteau sous le rift, quand la divergence des plaques a permis d’amincir la lithosphère continentale, de telle sorte que sa base soit moins profonde que le solidus du manteau asthénosphérique sous-jacent.
This active thermal equilibrium requires that plate divergence has already become durably localized (stage 1 on Fig. 7). Analogies with ultraslow spreading ridges indicate that this initial stage of strain localization should be expected to produce conjugate magnetic anomalies if it causes mantle exhumation, even without volcanism. Plate cooling and thickening with age away from this region of localized deformation should help to keep deformation and magmatism localized in the future, or “incipient” ridge region (Fig. 2). It is conceivable, however, that plate divergence and magmatism may relocate away from this incipient ridge region, causing the ridge to “stutter” [70]. Analogy with present-day ultraslow spreading ridges suggests that this is unlikely to happen once the incipient ridge has functioned for more than 10 Ma, because the off-axis oceanic lithosphere by that time should be more than 40 km thick (Fig. 2), preventing further relocalization of the main deformation zone.
This thick near-axis lithosphere would also bound a relatively narrow asthenospheric channel (Fig. 2), favoring the transition from a broad to a focused asthenosphere upwelling pattern, as postulated by Minshull et al. [103]. This evolution of the asthenospheric flow (stage 3 on Fig. 7) may produce a substantial increase in melt supply (Fig. 5a), from mostly a-volcanic OCT terrains, to the mostly volcanic terrains more typical of slow spread oceanic lithosphere. Compositional heterogeneities in the subcontinental mantle [109] could also affect melt supply at the new ridge.
The early life of the new ridge may also be complicated by broadly distributed post-breakup tectonic and magmatic events in the nearby OCT. These are now well documented in the Newfoundland-Iberia transect: morphotectonic event of Péron-Pinvidic et al. [120]; Aptian to Albian breakup unconformity of Tucholke and Sibuet [163]; polymagmatic evolution shown by Jagoutz et al. [70]. These events may result from local mantle convection patterns which have been proposed to form along the edge of the continent [14,105]. Such local convection cells may be expected to impact the dynamics of asthenospere upwelling at the new ridge.
4.2 Distribution of magmatism
Mantle exhumation, at both mid-ocean ridges and OCTs, clearly characterizes magma-poor plate divergence. Our review of slow and ultraslow spreading ridges shows, however, that the formation of an ultramafic seafloor does not necessarily mean that accretion is amagmatic. For one thing, melt distribution is segmented along-axis, with higher melt supply segment centres spaced by a few tens of kilometers (in the Atlantic), to a few hundred kilometers (in magma-poor sections of ultraslow spreading ridges). Widespread mantle exhumation occurs at segment ends [20,43], and even these domains are not amagmatic since ultramafic rocks are intruded and impregnated by a variety of magmatic rocks. A still poorly constrained proportion of these magmatic rocks results from melt crystallization below the crust (cf. Section 3.3). These deeply crystallized magmatic rocks and their ultramafic host rock would be tectonically exhumed by axial detachment faults, or accreted to deeper, upper mantle level in the diverging plates, where they would go undetected by seismic methods up to a proportion of ≈ 10%. Fig. 4 shows that the proportion of such subcrustal magmatic bodies is expected to be larger in ridge settings where magma is extracted from the asthenospheric mantle over a wide region, while faulting of the axial lithosphere is localized to a more narrow domain.
These mid-ocean ridge findings lead us to stress the potential for deep melt trapping to be an important process at melt-poor OCTs. The asthenospheric upflow zone at OCTs, i.e. the domain over which mantle melting may occur, is generally modelled as wider than the tectonic exhumation system [82,172]. Magma-poor OCTs, in addition, would, at least in the early stages of their evolution, have a thicker lithosphere than mid-ocean ridges (Fig. 7). This thick lithospheric lid would both stop mantle melting at greater depths (Fig. 8), and therefore reduce melt production, and offer more opportunities for melts to become trapped and to crystallize at depth. Anomalously low upper mantle velocities (7.5 to 7.8 km/s) in the ZECM of Iberia–Newfoundland [31,56,81,167], which have been interpreted as due to partial serpentinization, may thus also be at least partially due to magmatic rocks. This would be consistent with sampling of melt-impregnated peridotites, containing up to 20% former melt, as described from ODP drilling off Iberia [9,38,79,109], and in OCT remnants of the Alps [110].

Interpretation of the predrift evolution of a melt-poor continental margin system (between stages 0 and 1 on Fig. 7). The base of the ductile lithosphere is inferred to be limited by an isotherm, as on Figs. 2–4. Black spots are melt impregnations and deeply crystallized gabbros. Configurations (a) and (b) may develop successively, or may coexist along the margin, defining a melt-controlled segmentation. On (a) plate divergence is distributed over a wide domain, and melt intrudes the lower continental lithosphere, where it crystallizes slowly, releasing heat and bringing temperatures up to just below the solidus temperature of basaltic melts [148]. Heat conducted upward thins the brittle lithosphere. This phase of deep melt crystallization thus leads to a thermal erosion of the margin's lithosphere, maintaining shallow topography. Configuration (b) develops when localized extension leads to mantle exhumation. Subsidence may then occur both because the low-density crustal layer (comprised of tectonized and serpentinized peridotites) is thinner, and because localized deformation maintains high permeabilities in the upper lithosphere and allows for more vigourous hydrothermal cooling. This cooling effect is expected to be maximum if melts are not trapped into the deep lithosphere, but intrude into the hydrothermally cooled permeable upper lithosphere.
Fig. 8. Schéma interprétatif de l’évolution tardive d’une marge continentale peu magmatique (entre les étapes 0 et 1 de la Fig. 7). La base de la lithosphère ductile est supposée correspondre à un isotherme, comme sur les Fig. 2–4. Points noirs : imprégnations magmatiques et gabbros dans le manteau lithosphérique. Les configurations (a) et (b) pourraient se succéder, ou coexister le long d’une même marge, formant une segmentation associée à l’apport magmatique. En (a) la divergence des plaques est distribuée sur un large domaine. Du magma est piégé en profondeur et cristallise en dégageant de la chaleur qui maintient la température du manteau infiltré juste sous la température du solidus des magmas [148]. La conduction de cette chaleur amincit la lithosphère fragile sus-jacente. Cette étape de piégeage profond de magma conduit donc à une érosion thermique de la lithosphère et pourrait expliquer le maintien de topographies peu profondes, malgré un fort amincissement crustal. En (b), la divergence est localisée sur une faille qui conduit à l’exhumation du manteau. Cette étape devrait correspondre à une subsidence, car la croûte composée de manteau serpentinisé et de roches magmatiques est plus mince que la croûte continentale amincie de (a) et parce que la déformation localisée peut entretenir une perméabilité dans la lithosphère supérieure et donc favoriser le refroidissement hydrothermal. Ce refroidissement devrait être maximal si les magmas ne sont plus piégés en profondeur, mais mis en place dans le domaine à forte perméabilité qui est refroidi par l’hydrothermalisme.
Finally, the stuttering localization of the plate divergence at OCTs [70] would be expected to produce complex patterns of lithospheric thinning over the OCT region. By analogy with ridge melt migration models [88], we postulate that slopes in the basal surface of this irregular lithospheric lid could favor 3D melt migration and produce equally complex magmatic segmentation patterns (Fig. 8).
4.3 Hydrothermal circulation and the extent of serpentinization in the OCT's upper mantle
Upper mantle serpentinization down to depths of 4 to 10 km has been inferred from seismic data at magma-poor OCTs [31,40,55,146]. Numerical models of continental margin evolution postulate that serpentinization occurs down to the 400, or 500 °C isotherm, which, given the cold thermal structure inferred for OCTs, may indeed be 10 km deep [118]. However, our review of mid-ocean ridge data shows that significant serpentinization there does not extend to depths greater than 3 to 4 km. It could be argued that this 3 to 4 km depth limit corresponds to the depth at which axial temperatures are hotter than 400 °C, and thus too hot for efficient serpentinization [51]. If OCTs have a lower temperature gradient, serpentinization there could then be occurring at greater depths. This interpretation, however, also implicitly requires that permeabilities at depths greater than 3 to 4 km along slow spreading ridges are too low for an active hydrothermal convection to develop, because such a convection would then cool these permeable regions of the axis, allowing for deeper serpentinization.
We thus conclude that permeability is the primary factor, controlling both the thermal gradient, and the accessibility of water needed for serpentinization reactions. If we are right, inferred serpentinization at depths greater than 3 to 4 km at OCTs would require that the high permeability domain next to the detachment faults there was thicker than that inferred at slow spreading ridges. This may be the case if detachment faulting at OCTs involves bending of a thicker footwall plate. The alternative is that reduced seismic velocities at depths greater than 3 to 4 km at magma-poor OCTs are instead caused by deeply crystallized magmatic rocks (see previous section).
Serpentinization at mid-ocean ridges is at least partially associated to high temperature, black smoker-type hydrothermal cells, primarily fuelled by magmatic heat [2,86]. Although we still do not know how much serpentinization occurs in lower temperature and less vigourous axial hydrothermal cells which may not be fuelled by magmatic heat, there is therefore a distinct possibility that serpentinization is enhanced when magma is injected within the high permeability upper lithosphere. If this is the case, we would expect serpentinization to be less active in environments (magma-poor ridges and OCTs) where mantle exhumation is not accompanied by significant magmatism, or where magma is primarily crystallized in deeper lithospheric levels, well below the permeable upper domain of hydrothermal circulations.
4.4 Thermal regime, topography and subsidence
Thermal conditions in OCTs have direct implications for the survivability of syn- to postrift petroleum systems in deep margins, which represent one of the last frontiers for the exploration industry. The subsidence record provided by sedimentary sections is used as a proxy to reconstruct these thermal conditions. One of the most unexpected results of recent continental margin studies is that extreme crustal thinning at magma-poor OCTs appears to have occurred under shallow marine conditions. In the Newfoundland-Iberia system, it has been shown that both margins then rapidly subsided during the phase of mantle exhumation [119]. This indicates that the initial lack of subsidence cannot be explained by the addition of light material and must therefore be mainly due to a hotter thermal regime (otherwise the margin would not have subsided afterwards to the normal expected depth).
One of the hypotheses proposed to explain this delayed subsidence is that rapid crustal thinning was accompanied by large-scale melt infiltration in the continental mantle lithosphere [119]. Our review of mid-ocean ridge processes leads us to support this possibility: it stresses the potential for deep melt crystallization in the mantle lithosphere of plate divergence environments, and it suggests that melt produced in the upwelling asthenosphere may also migrate along the base of the lithosphere toward regions where a thermal anomaly has been initiated (Fig. 8). These regions may thus concentrate the melt produced over a broader domain of mantle upwelling. The thermal effect of deep melt trapping has been modelled by Sleep and Barth [148] for a simplified slow spreading ridge configuration. Melt heats the deep mantle lithosphere, and the maximum amount of melt which can crystallize corresponds to the release of latent heat which would heat the host rock to the melt solidus temperatures. Developments of this thermal model to adapt it to the case of OCTs would be needed, but the net effect can be predicted to be thermal erosion of the preexisting mantle continental lithosphere, with the formation of a thick domain of hot lithospheric mantle, which would be maintained at near basalt solidus temperatures (Fig. 8a). This hot thermal regime could allow basement topography to remain relatively shallow, even if the continental crust above is very thin.
Further lithospheric stretching, which may be expected to result in remelting of any melt crystallized at earlier stages, could then favor more durable strain localization, leading to mantle exhumation (Fig. 8b). Rapid subsidence would be expected at this stage due to further thinning of the low-density crustal layer (from thinned continental crust to thinner partially serpentinized ultramafic layer). The emplacement of melt at shallower depths in the lithosphere could also result in a more efficient loss of magmatic heat to hydrothermal fluids, and therefore in a colder overall thermal structure (Fig. 8b). We also expect enhanced hydrothermal heat loss at this stage, both because fracturation next to the exhumation fault should maintain high permeabilities [145], and because shallow melt bodies emplaced next to this highly permeable domain should provide the heat to run more vigorous hydrothermal convection [173].
Finally, seismic data in the Newfoundland–Iberia margin system suggest that the OCT at the mantle exhumation phase was deeper than the first basaltic oceanic crust [120]. This shallowing may be due to a larger influx of magma, resulting in the formation of a thicker crust, following the transition from unfocused to focused asthenospheric corner flow [103].
5 Conclusions
In classical plate tectonic models, the transition from rifting to seafloor spreading was assumed to be abrupt in time and space. This enabled one to define a precise age for continental breakup and to map an ocean–continent boundary that not only separated continental from oceanic crusts, but also two communities of researchers working with different concepts and models. The discovery of mantle exhumation at slow and ultraslow spreading ridges, and at OCTs has made this simple picture obsolete. Today it looks as if the transition from rifting to seafloor spreading is, at least in magma-poor systems, more transitional and complex, than previously suggested. This discovery not only questions paradigms, concepts and terms previously used to describe the limits between oceanic and continental crusts, but also encourages scientists to cross the borders between the ridge and margin communities. This is the approach we adopted in this paper, based on a review of key concepts, or questions, concerning slow and ultraslow spreading ridges. Rather than new results, the main outcome of our review is a proposition for a working model that includes four principal stages in the rift to drift transition at magma-poor OCTs (Fig. 7). In this model, we emphasize the role of the thermal regime, as a key parameter for tectonic, magmatic and hydrothermal processes associated with continental breakup.
Acknowledgments
We thank our two anonymous reviewers for their most helpful comments, and the convenors and participants of the “Ocean Continent Transition” workshop in 2007 in Paris (Académie des sciences). This work was partially supported by “Action Marges” and CNRS-INSU. This is IPGP publication number 2433.