1 Introduction
Basaltic glass alteration, also called “palagonitization” (Honnorez, 1967, 1972), results from the hydrolysis of the glass silicate network. Until recently palagonitization was considered to be an abiotic reaction, being first attributed to the action of bacteria by Thorseth et al. (1992). Thereafter, it has been hypothesized that bacteria “mediate” or “control” the alteration process, either by increasing or decreasing the alteration rate, and that bacterial activity is manifested by intricate patterns of holes and channels in the glass. The presence of pits in glass, even when bacteria are present, does not confirm that microorganisms have induced or contributed to the formation of the pits and to the glass alteration. Alteration of basalt glass is the natural consequence of the chemical disequilibrium between water and glass (e.g., Techer et al., 2001), so bacterial activity is not specifically needed to mediate or control the alteration process. Here, we demonstrate that microbe-sized holes in natural glass can be produced under strictly abiotic conditions.
A study of the altered glass rims of marine pillow basalts (Morgenstein, 1969) includes, to the best of our knowledge, the first description of remarkable features that are now often ascribed to bacterial alteration. Using optical microscopy of petrographic thin sections the author identified four zones of alteration, between the sideromelane (fresh glass) and fractures (Fig. 1 shows examples of these zones.). Outward from the margins of fractures in sideromelane, he described “palagonite”, then a “solid solution border”, then “microchannels”, and finally fresh glass/sideromelane. The author proposed that quenching of the magma and thermal contraction of the glass at the time of eruption produced stress-induced fractures, which were once open to the flow of seawater. The fractures were often subsequently filled with secondary minerals such as smectites and zeolites. “Palagonite” which consists of secondary products including phyllosilicates, zeolites and iron oxy-hydroxides formed along the edges of some of the fractures. A so called “solid solution border”, also called the “immobile product layer” was described next to the palagonite (Morgenstein, 1969). Remarkable “microchannels” or “hair-channels” averaging 50 μm in length extended into the fresh glass from the “solid solution border” (Morgenstein, 1969). In his samples with microchannels, the “solid solution border” is prominent, and where the microchannels are absent the “solid solution border” is diminished or absent. As already mentioned, until recently the reaction of water with basalt glass (palagonitization) was assumed to be an abiotic process, so Morgenstein logically adopted this interpretation in 1969. He hypothesized that lattice defects in the glass were responsible for the microchannel location and distribution.
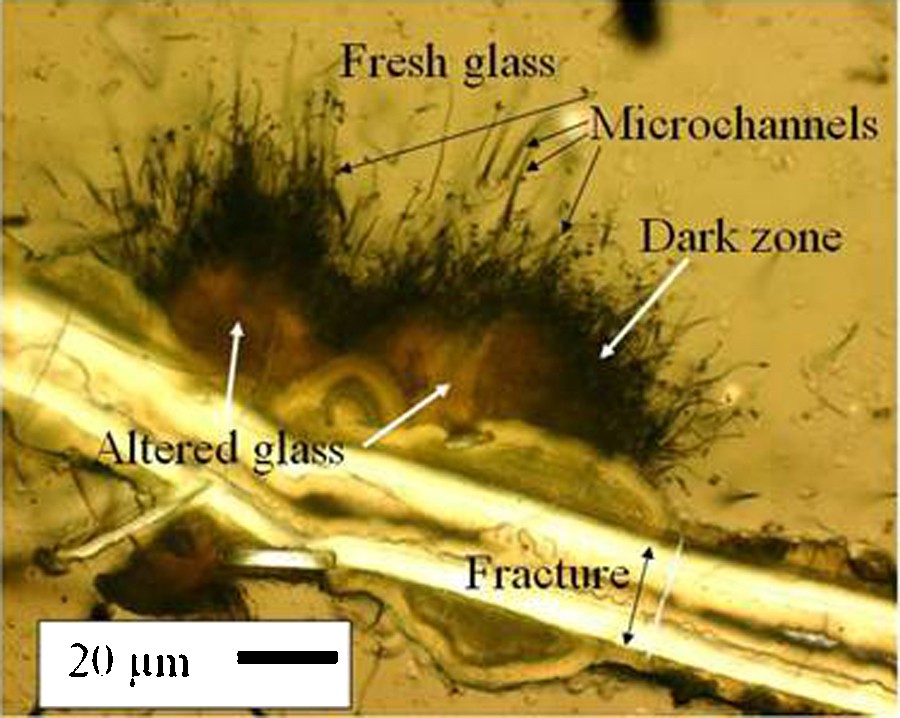
Microscopic image in plane polarized transmitted light of a thin section of sample P6704B-25-C1, a hyaloclastite from the Romanche Fracture Zone. A fracture, areas altered glass, a dark zone, microchannels, and fresh glass are labeled. The double-headed arrow indicates the width of the original fracture. Along sections of the fracture where microchannels are present, the zone of altered glass and the dark zone are wider than where microchannels are absent. In this area microchannels dominate one side of the fracture.
Microphotographie en lumière polarisée d’une lame mince de l’échantillon P6704B-25-C1, une hyaloclastite de la zone de fracture de la Romanche, océan Atlantique. Une fissure, des zones de verre altéré, une « zone sombre », des microchenaux et le verre frais sont indiqués. La double flèche correspond à la largeur initiale de la fissure. Le long de certains segments de la fissure où des microchenaux sont présents, les zones de verre altéré et la « zone sombre » sont plus épaisses que là où les microchenaux sont absents. Dans ce champ de vue, les microchenaux dominent sur un seul côté de la fissure.
Pit-textured glass of an Icelandic hyaloclastite was described and attributed to biological processes (Thorseth et al., 1992). Since then a large variety of alteration features in basalt glass from the ocean basins have been reported based on optical microscopy (OM) and on secondary electron (SE) and backscattered electron (BSE) images (Banerjee and Muehlenbachs, 2003; Cockell et al., 2009; Fisk et al., 1998, 2003; Furnes and Staudigel, 1999; Furnes et al., 1996, 1999, 2001a, 2001b; Giovannoni et al., 1996; Ivarsson et al., 2008; McLoughlin et al., 2009; Staudigel et al., 2004, 2008; Thorseth et al., 1992, 1995, 2003; Torsvik et al., 1998). In addition, similar features have been reported in ophiolites (Furnes et al., 2002, 2004, 2008a, b), Hawaiian hyaloclastites (Fisk et al., 2003; Walton, 2008), Archean pillow lavas (Banerjee et al., 2006; Furnes et al., 2008a), and a Martian meteorite (Fisk et al., 2006). These works rely on the pit and channel morphology and their distribution, or on their association with carbon or complex organic material, or on their relationships with shifts in isotopic ratios as evidence of biological involvement in their production.
Such a variety of microchannel morphologies is not easily explained by either microbial action or abiotic chemical reaction, but microorganisms are able to produce simple pits or grooves (but not microchannels) in volcanic glass (Buss et al., 2007; Thorseth et al., 1995; Staudigel et al., 1995), and the pits can be the same size and shape as bacteria (Thorseth et al., 1995). Although multiple lines of evidence suggest that some microchannels are produced by biological activity, abiotic processes, such as ambient inclusion trails or metamorphism have also been hypothesized to create some tunnel features that are similar in form to microchannels found in basalts and ophiolites (e.g. Lepot et al., 2011; McLoughlin et al., 2010). Some of these features are similar in size and distribution to microchannels in basalt glass. A mechanism of abiotic production of microchannels by chemical dissolution of glass is also possible, as will be described in this paper.
Abiotic corrosion of glass that results in cylindrical features is an example of a chemical process that produces microchannel-like features. Youssefi et al. (1979) and Youssefi, 1980 experimentally etched a lithium-silicate glass with a 1% HF + 5% HCl solution for 15 s (Fig. 2), consequently forming pits and groves. The diameters of the pits are similar to those seen in naturally altered basalt glasses, but the depths were not determined and these chemically generated microchannels are not strictly identical to those observed in naturally altered basalt glass. In addition the Youssefi et al. experiments were carried out with lithium-silicate glass so the results were not directly applicable to basalt. Clearly the biotic alteration of glass exists in some circumstances, and the aim of the present study is to examine the abiotic etching of natural and man-made glasses in order to compare these abiotic alteration features to others already published and attributed to biotic corrosion.
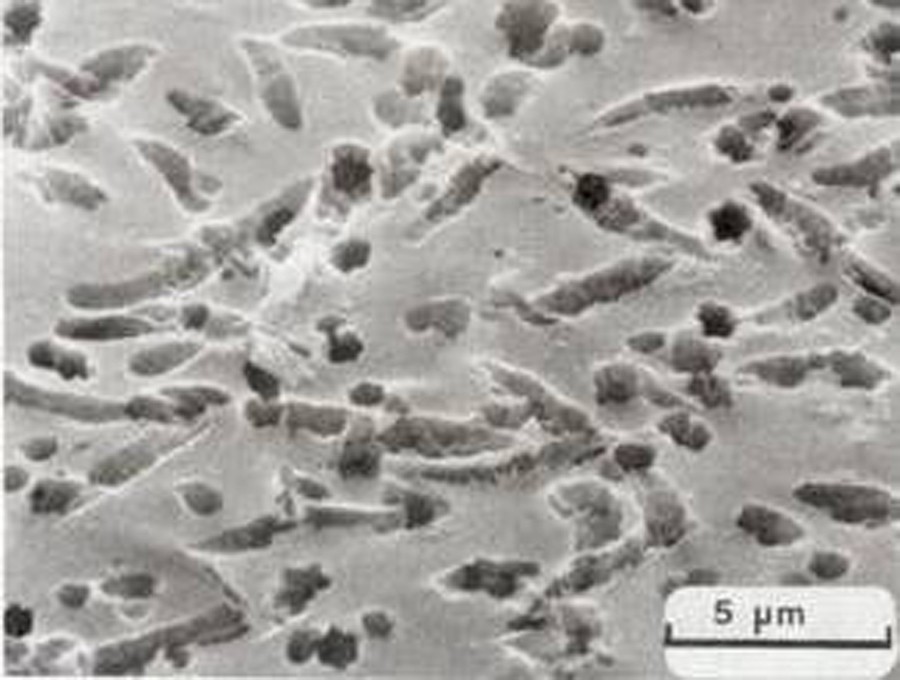
Scanning electron microscope image of the surface of glass etched with acid. Lithium-silicate glass containing 1, 2, 3, 4, and 5 mole percent of P2O5, K2O, Na2O, Al2O3, and MgO, respectively, and free of visible imperfections was etched with a solution of 1% HF and 5% HCl for 15 s at room temperature. The pits and grooves produced by the etching are the same size as those observed in geological glass that has been altered in the marine and subglacial environments.
Microphotographie au microscope électronique à balayage de la surface d’un verre attaqué à l’acide d’après Youssefi et al., 1979. Il s’agit d’un verre de silicate de lithium contenant, respectivement, 1, 2, 3, 4 et 5 mole pourcent(s) de P2O5, K2O, Na2O, Al2O3 et MgO. La surface du verre, qui ne présentait aucune imperfection visible, a été attaquée par une solution d’acides à 1 % d’HF et 5 % d’HCl pendant 15 s, à température ambiante. Les trous et sillons résultant de l’attaque à l’acide ont la même taille que ceux observés dans les verres basaltiques qui ont été altérés dans des environnements marins et sous-glaciaires.
From Youssefi et al., 1979, Figure 3b and Youssefi, 1980, Plate V, Photo 4.
We have etched natural and man-made glasses with HF. We emphasize that HF is used only as a proxy for acids that are found in igneous environments such as deep-sea hydrothermal vents (pH ∼ 2.8). HF allows for accelerated reactions in the laboratory. The purpose of this study is to demonstrate that a strictly abiotic alteration of glass could produce features that are similar to those obtained by a putative biotic alteration. Three different types of glass were chosen for the etching experiment: a basalt glass, an obsidian, and an ancient manufactured glass.
2 Sample and procedure description
Four glass samples were chosen for the acid etching study (Table 1). Two were quenched, basalt glasses from the sea floor; sample CYP78-04-07 from 21°N on the East Pacific Rise (Hekinian and Walker, 1987) and sample P6704B-25-C1 from the northern wall of the Romanche Fracture Zone (Bonatti et al., 1970), and two were high silica glasses, a fragment of a Gallo-Roman bottle (sample DC33, Crovisier et al., 2004; Crovisier and Advocat, 2007) and a rhyolitic obsidian from Lipari Island, Sicily, Italy (sample DC38). These samples were chosen for their range in silica content (Table 1) and for the different alteration degrees of the two sea floor samples. Sample CYP78-04-07 is a remarkably fresh glass with no evidence of alteration (Eissen, 1982) coming from a three-centimeter thick sheet-flow, whereas sample P6704B-25-C1 is a hyaloclastite cemented by pelagic sediment and having some alteration along fractures in the glass (Fig. 1). Both sea floor glasses were quenched in seawater and have microphenocrysts of plagioclase and olivine. Sample DC38 is from the well-characterized Lipari rhyolitic obsidian flow (Hunt et al., 1998). Sample DC33 was found below 4 m of sediment at the site of a Roman villa in Lorraine, France. It is a sodium-silicate glass with 69 wt.% SiO2 (Crovisier et al., 2004), and appears to have been buried between 175 and 200 C.E. This Gallo-Roman glass was annealed during manufacturing, and the massive Lipari obsidian can also be considered to have cooled slowly in subaerial conditions.
Échantillons de verre utilisés lors des attaques à l’acide.
Sample number | CYP78-04-07 | P6704B-25-C1 | DC38 | DC33 |
Location | EPR | RFZ | Lipari, Italy | Vic-sur-Seille, France |
Depth, m | 2650 bsl | 5100 to 5300 bsl | Surface outcrop | 4 (below soil surface) |
Material | Basalt | Basalt | Rhyolite obsidian | Gallo-Roman bottle |
SiO2, wt. % | 51a | ∼ basalticb | 73 to 75c | 69d |
Alteration | None | Slight | None | 100 μm alteration layer |
Mineralogy | ol, pl, cpx | ol, pl, sp | None | None |
a Glass, Eissen, 1982.
b Hyaloclastite, Bonatti et al., 1970.
c Glass, Hunt et al., 1998.
d Glass, Crovisier et al., 2004.
Samples used in the experiments were broken from larger pieces. They were not sawn so as to limit the production of fractures. Pieces were selected based on a minimum cross section of 1 cm2 and the appearance that they were homogenous and free of cracks. The selected pieces were mounted in separate 2.5 cm diameter epoxy blocks, ground and polished first with SiC paper of grit size 220, 600, 800, and 1200 and then polished with 10 and 1 μm diamond paste. The polished samples were ultrasonically cleaned in alcohol and etched by gentle agitation in a bath of 1% HF for 60 s at room temperature. The acid concentration and the duration of the etching were established from dissolution rates of silicate glass from the literature (e.g. Spierings, 1993). The concentration of HF is similar to and the reaction times slightly longer than those used by Youssefi et al. (1979) and Youssefi (1980). After etching, the samples were immediately (in less than 1 s) transferred to a bath of distilled water (also at room temperature) and agitated.
Samples were coated with gold, and observed and photographed with a scanning electron microscope (SEM) before and after etching. Before etching the whole polished surface was examined systematically, using microphenocrysts as landmarks, and recognizable regions of the surface were photographed at low magnification. The SEM is a JEOL JSM 840 operated at 15 kV with a beam current of 1 to 3 nA. After the SEM examination of the unetched samples was completed, the gold coating was removed from the samples with brief (about 1 min) polishing with SiC 600, 800, 1200 grits and 10 and 1 μm diamond paste. After HF etching, a new gold coating was applied and the sample was again examined with the SEM by returning to the same characteristic areas photographed before etching. The areas were photographed again at the same magnification as the SEM images taken before etching, and at higher magnification where differences were observed.
3 Results
3.1 Untreated polished samples
In preparation for the etching study a number of areas of each of the two samples P6704B-25-C1 and CYP78-04-07 were mapped and imaged with an SEM. Images of two untreated, polished areas of sample P6704B-25-C1 (Fig. 3a and d) and one area of sample CYP78-04-07 (Fig. 4a) were found to be free of alteration. Euhedral plagioclase microphenocrysts are slightly darker than the surrounding matrix. In sample P6704B-25-C1, fractures in the glass are barely evident in the untreated polished surface but are indicated by the alignment of polishing pits. These polishing pits are typically 5 μm long and 1 μm across and their long dimension aligns with the fractures. Where sample P6704B-25-C1 is free of fractures it is also free of polishing pits (Figs. 3a and d). The surface of sample CYP78-04-07 has more polishing pits, mostly visible along the fractures, than does sample P6704B-25-C1 (Fig. 4a). Some of these fractures radiate from plagioclase grain (Fig. 4a). The Lipari obsidian and the Gallo-Roman glass had no fractures or crystals, but some pits were created by polishing (not shown).
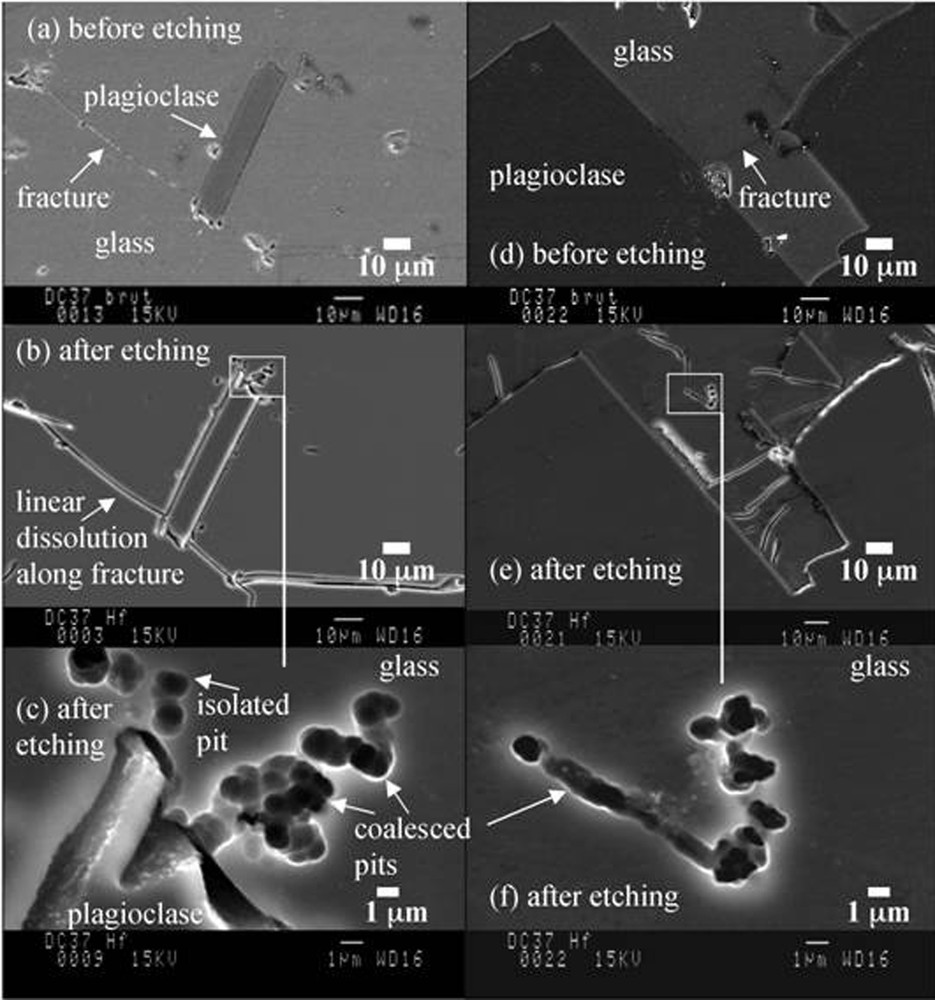
SEM images of two regions of sample P6704B-25-C1. (a) Before etching. A 60 μm long plagioclase microphenocryst in basalt glass. A fracture is indicated. Pits are only found along fractures. (b) After etching. The same plagioclase microphenocryst as seen in (a). Dissolution pits at the top end of the microphenocryst are enlarged in (c). (c) Isolated and coalesced dissolution pits in glass. The plagioclase grain is labeled. (d) Before etching. A > 100-μm plagioclase microphenocryst in basalt glass. No pits are present. (e) After etching. The same view as (d). Etch pits are highlighted by the frame and also shown in (f).
Microphotographies au microscope électronique à balayage de deux zones de l’échantillon P6704B-25-C1. (a) Avant attaque à l’acide : un microphénocristal de plagioclase de 60 μm de long est présent dans le verre basaltique. Des trous sont uniquement présents le long des fissures, dont l’une est indiquée. (b) Après attaque : le même microphénocristal de plagioclase est visible, mais les trous de dissolution à l’extrémité supérieure du microphénocristal sont agrandis en (c). (c) Trous de dissolution isolés et groupés dans le verre. Un microphénocristal de plagioclase de plus de 100 μm de long est visible dans le verre basaltique ; avant attaque, aucun trou de dissolution n’est visible. (e) Après attaque : même vue que (d) : les trous de dissolution sont mis en relief par le cadre et également montrés dans (f), où des trous groupés sont alignés pour former un microchenal de 13 μm de long et 1 μm de diamètre.
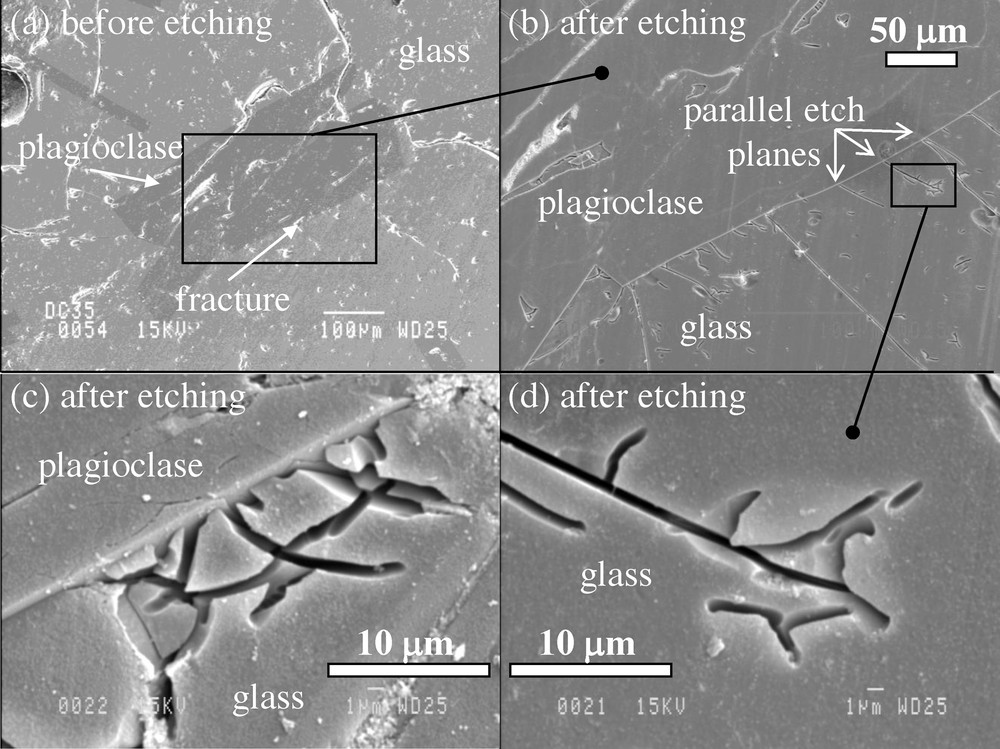
SEM images of sample CYP78-04-07 before etching and after etching. (a) Before etching. A plagioclase grain enclosed in glass. Pits created by polishing follow fractures that radiate from the plagioclase but no pits are found away from fractures. (b) This is the frame in (a) it shows parallel etch planes after etching. (c) After etching. Curved dissolution planes at the edge of a plagioclase crystal. (d) After etching. Detail from (b) showing flat dissolution plane with branches.
Microphotographies au microscope électronique à balayage de l’échantillon CYP78-04-07, le bord vitreux d’une coulée basaltique de 3 cm d’épaisseur provenant de la dorsale Est Pacifique, à 21°N de latitude. Avant l’attaque à l’acide : (a) : un microphénocristal de plagioclase dans le verre basaltique. Les trous/sillons provoqués par le polissage suivent les fissures qui rayonnent à partir du plagioclase mais aucun trou de corrosion n’est observé au-delà des fissures. (b) Après attaque : le cadre dans (a). (c) Après attaque : plans de dissolution courbes le long de l’arête du microphénocristal de plagioclase. (d) Après attaque : détail de (b) montrant des plans de dissolution présentant des ramifications.
3.2 Hydrofluoric acid-etched samples
Images at two magnifications are shown for the HF-treated sample P6704B-25-C1 (Figs. 3b, c, e, and 4f), and at three magnifications for sample CYP78-04-07 (Fig. 4b, c, and d). The features that are relevant to this investigation are the roughly equidimensional holes (pits) (Fig. 3b, c, e, and f), and the linear holes (channels) (Figs. 3f, 4c and d). Other effects of the HF treatment are:
3.2.1 Sample P6704B-25-C1
Resulting from etching with HF, the isolated pits visible in the glass of sample P6704B-25-C1 (Figs. 3b, c, e and f), are 1 to 2 μm in diameter, appear to be less than 1 μm deep, and have smooth, spheroidal interiors. The 1-μm pits can coalesce into globular clusters to form larger pits that are 5 μm or more in diameter (Fig. 3c) or to form linear arrays of pits that are 10 μm long and 1 to 2 μm wide that do not follow the visible fractures (Fig. 3f). The coalesced pits also have smooth, spheroidal surfaces. Where the termini of coalesced pits were imaged they appear to be deeper than they are wide. Discrete and coalesced pits can occur within a few micrometers to more than 20 μm away from feldspar grains, so that their location does not appear to be related to the presence of microphenocrysts. Pits are relatively rare: together the isolated and coalesced pits are estimated to cover less than 1% of the glass surface. The new pits in sample P6704B-25-C1 do not appear to follow fractures in the glass, and it may also be noted that the enhanced dissolution of preexisting fractures did not produce pits in this sample (Fig. 3b). The pits created by etching are here different from features produced by etching of the East Pacific Rise basalt glass (sample CYP78-04-07) and the two high silica glasses (samples DC33 and DC38).
3.2.2 Sample CYP78-04-07
Etching of sample CYP78-04-07 glass produced planar features (Fig. 4c and d) and not the isolated and coalesced pits that were observed in sample P6704B-25-C1. The acid corrosion viewed with the SEM appears here as straight or curved planar grooves that intersect the polished surface of the glass to make 1 μm wide and 2 to 30 μm long linear openings (Fig. 4c and d). The planes dip steeply into the glass and their termini are not visible. The etched surfaces of the planes appear smooth and not spheroidal as was the case in sample P6704B-25-C1. The planes can connect to the surfaces of plagioclase phenocrysts, or they can be isolated from them. Planes depicted on Fig. 4c are curved, some of which originate at the surface of a plagioclase grain. Fig. 4d shows a flat plane that originates at the surface of a plagioclase grain and has several branches.
No mineral precipitates were observed after the one-minute etching experiments (Figs. 3 and 4). Etching for 2 min, however, produced abundant secondary mineral precipitates that made observation of the etched features impossible with the SEM. This prevented us from determining how the features grew with increased duration of etching, such as by broadening or deepening of pits or planar features.
3.2.3 Samples DC33 and DC38
Etch pits or planes were not produced in the two high silica glasses (the ancient Roman glass and the Lipari obsidian, Table 1) when treated with 1% HF for 60 s. Etching only removed surface irregularities.
4 Discussion
4.1 Etch pits – “biotic” and “abiotic”
Pits formed in glass under non-sterile conditions, for instance exposed to seawater or incubated in bacterial cultures, have the approximate dimensions of microorganisms are referred to here as “biotic”, although they were not necessarily produced by microorganisms. SE images of such “biotic” pits in marine basalt glass that was exposed to seawater on or below the sea floor, show altered glass, biofilms, and attached microorganisms. These samples exhibit shallow circular, oval, or rounded rectangular pits about 1 μm in diameter (Thorseth et al., 2001, 2003), which can be isolated or form clusters or chains. Basalt glass, which was placed in bacterial cultures, was also found to have pits that have rounded rectangular or oval forms about 1 μm in diameter occurring either isolated or grouped in clusters or chains (Thorseth et al., 1995). In both of these “biotic” examples, microbial cells are present and are about 1 μm long. The depth of the pits appears to be less than 1 μm. Some pits in the glass from the sea floor (Thorseth et al., 2001, 2003) and from cultures (Thorseth et al., 1995) are virtually indistinguishable.
The abiotic pits obtained in sample P6704B-25-C1 (Fig. 3) by 1% HF etching are similar in size and distribution to those attributed to biotic alteration of natural marine basalts (Thorseth et al., 2001, 2003) and of glasses exposed to biotic experiments (Thorseth et al., 1995). The abiotic pits produced here are typically about 1 μm in diameter and can be found isolated or arranged in clusters and chains. The acid-etched abiotic pits, however, have sharp edges rather than the subdued rounded edges of the pits in glass from cultures. Also rectangular pits with rounded corners, which are present in samples where bacteria can be found (natural and experimental), are not present in the acid samples treated here. The acid-etched coalesced pits from this study form deeper (∼ 1 μm) holes in the glass surface (Fig. 3c and e), whereas clusters of pits in the “biotic” glass appear to be less than 1 μm deep. Where acid-etched pits coalesced into a linear feature or a groove (Fig. 3e and f), the groove is about 1 μm wide. In a biotic experiment, 0.5-μm wide grooves were produced in basalt glass that was exposed to microorganisms for 410 days (Staudigel et al., 1995). Thus, acid-etched pits are similar in size and distribution to pits in glass exposed to microorganisms, but their shapes are not identical to biotic pits. Considering the extremely different conditions of the previous biotic experiments and the abiotic experiments reported here, it is remarkable that the pits are similar in size.
4.2 Etch planes
The etch planes are two-dimensional features that are morphologically distinct from one-dimensional microchannels seen in basalt glass (e.g., Fig. 1). In the acid-etched sample CYP78-04-07, no pits were observed but etch planes appear parallel to each other in the vicinity of feldspar crystals and are separated from each other by about 10 to 50 μm (Fig. 4b). On Fig. 4c the planes form arcs that intersect a plagioclase microphenocryst. On Fig. 4b and 4d a flat plane intersects a plagioclase microphenocryst and extends about 50 μm from the plagioclase surface (Fig. 4d). Etch planes were not observed in the etched samples P6704B-25-C1, DC33 and DC38, nor in basalt glass altered in natural settings. However, similar planar features were described in the altered surface of Medieval cathedral glass (Garcia-Valles et al., 2003). The cause of the shape and distribution of etch planes is not known, but it could be related to stress in the glass caused by the different thermal expansion coefficients between glass and plagioclase grains. Differential contraction of the glass and the mineral during cooling may result in the development of stresses in the glass adjacent to the phenocryst and the etched planes could reflect these stresses. Unaltered basalt glasses often contain curved fractures around microphenocrysts further suggesting that curved etch planes in CYP78-04-07 (Fig. 4c) are related to stress in the glass. Stained glass can also have similar curved fractures (Libourel et al., 2011).
4.3 Microchannels
As mentioned, microchannels are best observed in OM petrographic thin sections. SEM images of polished or fractured surfaces provide only limited information about the third dimension of microchannels. However, producing thin sections that intersect acid-etch pits shown on Figs. 3 and 4 is challenging. Even so, comparisons between three-dimensional optical images and two dimensional electron images can be made.
The abiotic coalesced pits of the etched glasses (Fig. 3) share several of the features of microchannels found in basalt glass in nature:
- • the microchannels are up to 5 μm in diameter, and thus similar in diameter to coalesced pits. Some coalesced acid-etched pits appear to penetrate at least 5 μm into the glass surface nearly perpendicular to the glass surface (Figs. 3c and e), but the maximum depth of the pits could not be determined by SEM. Whether the pits extend into the glass for tens of micrometers as do the microchannels in basalt is not known;
- • many microchannels in natural samples are perpendicular to the glass surface where they originate and most deep etch pits in our experiments also appear to penetrate perpendicular to the glass surface;
- • microchannels in natural samples are not evenly distributed on glass surfaces. For example, some areas of fractures have microchannels while others do not (Fig. 1), and microchannels can occur on one face of a fracture while the opposing face is free of microchannels. The HF induced pits are also not uniformly distributed over the glass surface, but appear in isolated areas (Fig. 3), but what controls the distribution of deep coalesced pits is not known.
There are also physical differences between microchannels found in basalt glass in biotic environments and coalesced pits produced by HF etching experiments. The coalesced pits (Fig. 3c) appear to be much broader at the surface than at a depth of a few micrometers. This suggests that the diameter of the coalesced pits decreases with depth in the sample. In contrast, microchannels in basaltic glass often maintain a near constant diameter over their length, although sometimes expanding at their termini. However, the coalesced pits in this study are similar to granular textures that have been reported in volcanic glasses and have been attributed to microorganisms (Furnes et al., 2001a, 2008b; Thorseth et al., 2003).
4.4 Characteristics of etching
Etching of glass depends on multiple factors, some of which being external to the glass, such as fluid pH, etching agent, temperature, and pressure, and some of which being specific to the glass such as its composition and homogeneity, its cooling and annealing history, and the presence of crystals (Crovisier et al., 1987; Youssefi, 1980). In our experiments, the pH, etching agent, temperature, and pressure were all constant, so that differences in the style of etching should only depend on the characteristics of the glass. In sample P6704B-25-C1, etching occurred as isolated pits and not as curved planes around crystals. Sample CYP78-04-07 displayed no etch pits. Both samples are chemically and mineralogically similar, and both were quenched from their eruption temperature of about 1200 °C to ambient seawater temperature (about 4 °C). The features that distinguish the two samples are their emplacement mechanism and their ages. Age is not considered to be a factor in stress release at ambient seawater temperatures (∼ 4 °C); however, the mechanism of emplacement may be important. Sample P6704B-25-C1 is a hyaloclastite made up of small glass shards cemented by sediment, whereas sample CYP78-04-07 is a solid “inch-thick” lava flow. The cooling and therefore the annealing histories of these two samples are quite different. In the case of sample P6704B-25-C, glass shattering could dissipate the thermal stress-induced by quenching, whereas the unfractured sheet-flow sample (sample CYP78-04-07) most probably contains “locked in” thermal stress.
We interpret the difference in the style of etching by HF between the basalt glasses and the high silica glasses (samples DC33 and DC38) to be due to either the glass chemistry or their annealing history. Neither etch pits nor etch planes were observed in high silica glasses, but etching did remove a uniform layer of glass and consequently removed irregularities in the glass surface. The higher silica content may diminish the acid etching impact on the glass lattice. But we rather suggest that the natural annealing of the Lipari obsidian and the “factory” annealing of the Gallo-Roman glass, as well as the absence of heterogeneity, disorder, and crystals in these glasses may be responsible for the absence of etch pits and etch planes.
Conditions of the etching experienced by natural basalt glass in the oceans are significantly different from the conditions in our HF etching experiments. Basalts typically were exposed to seawater or slightly modified water at temperatures of 2 to 30 °C for thousands to millions of years. In contrast, the experimental abiotic pits made here result from contact with a 1% HF solution for 60 s at room temperature. The similarity in the size, shape, and distribution of HF pits produced here with pits found in biotic samples again suggests that it is the glass and not the conditions of etching that is controlling the style of the produced features. However, the different etching agents and duration of etching also influence the details of the etch pits, such as their depth (> 1 μm in abiotic experiments and < 1 μm in biotic samples), or morphology (sharp edges in abiotic experiments and rounded edges in biotic samples).
5 Conclusions
Abiotic glass-etching experiments using 1% HF (as a proxy for more slowly acting acids) addresses the question of the origin and morphology of pits and microchannels in natural and synthetic glass exposed to alteration agents in nature. Secondary electron images of the surface of polished basalt glasses taken before and after acid etching with 1% HF for 60 s at room temperature demonstrate the effect of the acid on the glass. In one sample, the pits produced on the surface of basalt glass are similar in size and distribution to pits attributed to microbial action on the surface of basalt glass recovered from the sea floor and basalt glass exposed to microorganisms in laboratory cultures. Acid-generated coalesced pits are similar to microchannels found in putatively biologically altered basalt glass. Although there is evidence that microbes create microchannels in glass, there are no paired before and after pictures that demonstrate the effect. The similarity of the pits produced by 1% HF acid etching and pits found in glass subjected to microbial cultures is intriguing, given the extremely different conditions of etching. This suggests that the location and style of pitting is probably dictated by the structure, stress, and heterogeneities in the glass rather than by the environment in which the pitting occurs. High silica glasses are not pitted by HF, suggesting that glass composition also affects etching. As the mechanism(s) for producing microchannels in basalt glass biotically or abiotically has not yet been demonstrated, abiotic production of microchannels is a viable alternative interpretation to the hypothesis that they are created or mediated by microorganisms. If microchannels were produced abiotically, then the organic and inorganic chemical indicators of life that have been found in them would have been introduced after the microchannels were created. Until biotic formation of microchannels can be demonstrated, their use as biomarkers should be approached with caution.
Acknowledgements
We acknowledge Martin Fisk's funding by the Université de Strasbourg, France, while working on this project.