1 Introduction
The decline of stratospheric ozone in the 1980s (Farman et al., 1985; Hofmann et al., 1994; Solomon et al., 1986) led to increased levels of the UV radiation that reaches the Earth's surface (Fioletov et al., 2001; Kerr and McElroy, 1993; Lakkala et al., 2003; McKenzie et al., 1999) and raised the concerns over the impact that these changes might have (WHO, 1994; WMO, 2007). As shown in the studies of Newman and McKenzie (2011) and Chipperfield et al. (2015), the continuous UV increase might have detrimental effects on both human populations and ecosystems. In the effort to avoid such an outcome, most countries signed the Montreal Protocol in 1987 and agreed to adopt measures to minimize the emissions of ozone-depleting substances (ODS). At the same time, large global networks for the monitoring of solar UV irradiance at the Earth's surface were established in order to improve the understanding of the interactions between solar UV radiation and other atmospheric constituents, and accurately inform the public about changes in solar UV radiation (Fioletov et al., 2004). Measurements of the spectral solar UV-B irradiance are thus available since the end of the 1980s or the beginning of the 1990s at a number of historical stations around the world – e.g., Bais et al. (1993), Kaurola et al. (2000), Zerefos (2002). The importance of UV radiation for humans and for the balance of ecosystems (Asta et al., 2011; Lucas et al., 2015; UNEP, 2010; Williamson et al., 2014) makes continued accurate monitoring imperative. Since satellite instruments perform indirect estimates of UV levels (A Jebar et al., 2017; Cadet et al., 2017; Zempila et al., 2017; Zempila et al., 2016), the proper maintenance and uninterrupted operation of ground-based networks is of exceptional importance.
Many recent studies show that the measures adopted after the implementation of the Montreal protocol were successful and that the weakening of the ozone layer has decelerated since the mid-1990s (Egorova et al., 2013; Mäder et al., 2010) and the first signs of recovery are now evident over both the Northern (Kuttippurath et al., 2013; McLinden and Fioletov, 2011; Newchurch et al., 2003; Smedley et al., 2012) and the Southern Hemispheres (Chipperfield et al., 2017; Kuttippurath and Nair, 2017; Solomon et al., 2016) although it is still debatable whether the recovery will continue in the future (Bernhard et al., 2013a; Manney et al., 2011). As a result of this reported ozone recovery, solar UV-B radiation reaching the surface has decreased during the last two decades over the high latitudes of the Northern Hemisphere (Bernhard, 2011; Eleftheratos et al., 2015). In the future, climate change driven shifts in the atmospheric circulation patterns are projected to induce additional increase of ozone leading to super-recovery, reducing further the levels of UV-B radiation (Hegglin and Shepherd, 2009; Tourpali et al., 2009). Over these high latitudes, other geophysical parameters that also strongly influence the levels of the surface UV irradiance, such as clouds and surface reflectivity, are affected enormously by climate change (IPCC, 2013). Changes of these parameters already affect the variability of the UV irradiance over very high latitudes (Bernhard, 2011; Bernhard et al., 2007; Bernhard et al., 2013b) and are projected to induce additional reduction in its future levels (Fountoulakis and Bais, 2015; Fountoulakis et al., 2014).
Contrary to what happens at higher latitudes, positive trends in UV-B irradiance have been detected over many locations of the Northern Hemisphere mid-latitudes during the last two decades (De Bock et al., 2014; Fitzka et al., 2012; Fountoulakis et al., 2016a; Román et al., 2014; Zerefos et al., 2012). The main reason seems to be the improvement of air quality levels, since aerosols, the emissions of which have been reduced over Europe, North America and many regions of Asia (Mao et al., 2014; Wild, 2011; Zerefos et al., 2009), are among the main factors reducing surface UV levels (Arola et al., 2003; Fragkos et al., 2015; Kazadzis et al., 2009b). Zerefos et al. (2012) suggest that there is a deceleration in the increase of the UV-B irradiance over Canada, Europe, and Japan since the mid-2000s. Fountoulakis et al. (2016a) confirmed these findings for the station of Thessaloniki, Greece, and also suggested that the long-term changes in UV irradiance could be fully explained only by assuming changes in the aerosol absorption efficiency (i.e. in the aerosol physical and chemical properties) in addition to the changes of the aerosol optical depth (AOD) and ozone. Changes in aerosols and clouds are projected to continue playing a major role in future changes of the surface UV irradiance (Watanabe et al., 2011), generating the need for further studies that will shed light on the complex interactions between these parameters (Bais et al., 2015).
The present study is an extension of the works by Zerefos et al. (2012) and Fountoulakis et al. (2016a), this time using data from 10 historical stations over Canada, Europe, and Japan for a 25-year period (1992–2016). The long-term variability of the UV-B irradiance has been studied with respect to the variability of total ozone, absorption by aerosols, and changes in cloudiness and surface reflectivity.
2 Data and methodology
Data from ground-based stations and satellite sensors have been used for this study. UV-B irradiance and total ozone data were derived from ground-based instruments; aerosols, cloudiness, and surface reflectivity were derived from satellite instruments. As suggested by several studies, the spatial variability of the UV radiation within a satellite pixel may be large, even of the order of 100% (Kazadzis et al., 2009a), mainly due to the corresponding large variability of aerosols and clouds within the pixel (Cadet et al., 2017; Zempila et al., 2018). Over complex terrains with variable surface reflectivity, differences in the UV irradiance of the order of 15% within 20 km have been reported, even under low-aerosol conditions and cloudless skies (Kreuter et al., 2014). Since satellite retrievals provide averages within pixels of ∼ 50 × 50 km2 or wider, quantitative estimates of the variability of these parameters may not be fully representative for all stations. However, reliable ground-based measurements of aerosols, cloudiness, and surface reflectivity are not available for all stations and for the entire period of the study. These uncertainties are more important for the short-term variability of UV-B radiation, and less important for its long-term variability. Nevertheless, the measurements used in this study are averages from 3 or 4 stations for each continent, which is expected to suppress these uncertainties and lead to reliable estimates regarding at least the direction of the changes.
2.1 Spectral UV-B irradiance
For the present study, Northern Hemisphere stations providing continuous spectral UV-B measurements during a 25-year period (1992–2016) were chosen. The list of stations and the corresponding data availability for each station are listed in Table 1. Although available for some sites, measurements before 1992 are not used for the sake of consistency and comparability between the results from different stations. Spectral UV measurements were obtained from the World Ozone and Ultraviolet Radiation Data Centre (WOUDC) (WMO/GAW UV Radiation Monitoring Community), the European UV Database (EUVDB) (Heikkilä et al., 2016) and from the Laboratory of Atmospheric Physics of the Aristotle University of Thessaloniki, Greece (LAP-AUTH).
List of the Northern Hemisphere stations providing spectral UV measurements at least since 1992, used for the present study.
Station (latitude, longitude) | Instrument type | Data source |
Canada | ||
Churchill (58.75 °N, −94.07 °E) | Brewer MKII | WOUDC |
Edmonton (53.55 °N, −114.10 °E) | Brewer MKII | WOUDC |
Saturna Island (48.78 °N, −123.13 °E) | Brewer MKII | WOUDC |
Japan | ||
Naha (26.20 °N, 127.67 °E) | Brewer MKII, Brewer MKIII | WOUDC |
Sapporo (43.05 °N, 141.33 °E) | Brewer MKII, Brewer MKIII | WOUDC |
Tateno (36.05 °N, 140.13 °E) | Brewer MKII, Brewer MKIII | WOUDC |
Europe | ||
Uccle, Belgium (50.80 °N, 4.36 °E) | Brewer MKII | WOUDC |
Reading, UK (51.44 °N, −0.94 °E) | Optronics, Bentham | EUVDB |
Sodankylä, Finland (67.36 °N, 26.63 °E) | Brewer MKII | EUVDB |
Thessaloniki, Greece (40.63 °N, 22.96 °E) | Brewer MKII | LAP-AUTH |
At nine of the overall ten stations used for this study, spectral measurements in the UV-B region are continuously performed since at least 1992 by a single monochromator of type Brewer MkIII (Kerr, 2010). Measurements are from a double monochromator Brewer MkIII spectrophotometer in Sapporo and Tateno after 1 January 2001, and in Naha since 9 September 2006. At the station of Reading, UK, spectral measurements in the UV-B for the period 1992–2003 have been performed by an Optronics 742 spectrophotometer while, since 2003, measurements are performed by a Bentham DM150 spectrophotometer (Bartlett and Webb, 2000; Smedley et al., 2012).
The solar irradiance at 307.5 nm is highly affected by changes in total ozone and at the same time is absorbed less effectively by sulfur dioxide compared to wavelengths in the range 306–309 nm. Thus, the particular wavelength was chosen to study the changes of the solar UV-B radiation relative to the corresponding changes of the total ozone column. Although wavelengths shorter than 306 nm are affected more effectively by changes in ozone, the measurements at these wavelengths are more uncertain (e.g., Bais et al. (2001)) due to the stray light effect (Karppinen et al., 2014) in single monochromator instruments and the low signal-to-noise ratio (Fountoulakis et al., 2016b), especially at large solar zenith angles and/or under cloudy conditions. In the following, the irradiance at 307.5 nm is referred to as UV-B irradiance.
Well maintained and calibrated scanning spectrophotometers provide reliable measurements, with low uncertainty of the order of 5% (Bernhard and Seckmeyer, 1999; Garane et al., 2006; Gröbner et al., 2006), though usually with low temporal resolution – e.g., Zempila et al. (2016). For that reason, calculating the daily integrals may be misleading. Hence, taking into account that most instruments are regulated to perform at least one measurement near local noon, we analyzed the irradiance reported for that time of day. Furthermore, changes of irradiance at local noon are highly representative of changes in the corresponding daily doses (integrals of the UV irradiance over the day) since the greatest fraction of the solar irradiance at short UV wavelengths reaches the surface in a time period of 1–2 h around local noon (i.e. the time of the day when the solar elevation is maximum).
The irradiance at local noon is calculated as the average of the irradiance measured within 30 min around local noon. For clear-sky conditions, the averaging is extended to 60 min around local noon to increase the amount of data entering the mean. Thereby the value assigned to noon irradiance is slightly underestimated, but the uncertainty of the trend is not strongly affected because a large number of measurements are considered in calculating UV-B trends and climatology. Climatological values for each day of the year and each station are calculated for the entire period of study by averaging the noon irradiance for each day of the year across all 25 years. These values are subtracted from the entire dataset and the daily anomalies (% change relative to the climatological values) are calculated. The daily anomalies for each month are averaged to derive the monthly anomalies. Monthly anomalies are used in the analysis only if data are available for at least 15 days of each month. The 11-year solar cycle and the Quasi-Biennial Oscillation (QBO) of the winds in the equatorial stratosphere affect stratospheric ozone and clouds, and subsequently UV-B radiation. Thus, their effects have been removed using the methodology described in Zerefos et al. (2012). Monthly means for the solar flux at 10.7 cm were downloaded from the NOAA National Geophysical Data Centre (http://www.ngdc.noaa.gov/stp/space-weather/solar-data/solar-features/solar-radio/noontime-flux/penticton/), while for the QBO wind data were downloaded from the Freie Universität Berlin (http://www.geo.fu-berlin.de/en/met/ag/strat/produkte/qbo/index.html). Finally, the monthly anomalies were averaged to derive annual mean anomalies. For Sodankylä, the annual mean anomalies were calculated using months from April to September to avoid high uncertainties due to the very large noon solar zenith angles (SZAs) in winter. For the nine remaining stations, annual anomalies are not used in the analysis for each individual station, if data are available for less than seven months. The annual mean anomalies were averaged for the stations in Canada, Europe, and Japan using equal weights for each year and each station, and the trends for each of these three regions were calculated.
For the station of Thessaloniki, analysis of the spectra measured under clear skies was performed in addition to the analysis for the all-sky cases. For this station, detection of the cloudy cases is achieved using data from a collocated pyranometer as described in Vasaras et al. (2001). Although detection of the clear sky cases is also possible for Sodankylä and Reading using the algorithm implemented in EUVDB (Heikkilä et al., 2016), the small number of clear-sky cases makes the analysis highly uncertain for these stations.
2.2 Total ozone from ground-based measurements
Archived total ozone column measurements performed by Brewer and Dobson spectrophotometers under the auspices of the World Meteorological Organization, Global Atmosphere Watch network, WMO/GAW, are routinely deposited at WOUDC and were used in this study. WOUDC total ozone column data from a large number of stations have already been used extensively both for ozone trend and ozone layer monitoring studies (WMO, 2014) as well as for satellite total ozone data validation purposes (e.g., Bak et al., 2015; Balis et al., 2007; Garane et al., 2017; Koukouli et al., 2015; Labow et al., 2013; Loyola et al., 2011). According to Kerr et al. (1985), the estimated uncertainty in the retrieval of total ozone from the Brewer spectrophotometers is about 1%. The corresponding uncertainty for the Dobson spectrophotometer is about 1% for cloud-free direct-sun observations and 2–3% for zenith-sky or cloudy observations (Van Rosendael et al., 1998). In this work, daily mean total ozone columns reported between 1992 and 2016 by Brewer spectrophotometers (for stations over Europe and Canada) and Dobson spectrophotometers (for the three Japanese stations) have been used. For Reading, ozone data from three satellites that were used as ground-based measurements are not available before 2003. A more detailed description of the three different satellite instruments and the corresponding datasets is provided in the following section, since datasets from the same instruments have been used to study the aerosol index. The methodology for the calculation of the annual mean anomalies from the daily means and the corresponding trends is the same as that for the spectral UV irradiance.
2.3 Aerosol index and total ozone from satellite records
The aerosol index (AI) is a measure of the radiative effect of aerosols and has been derived using the TOMS aerosol algorithm that has been described in detail by Herman et al. (1997) and Torres et al. (1998). Non-absorbing aerosols, such as sulfates and sea-salt particles, result in negative AI values, whereas UV-absorbing aerosols, such as dust and smoke, result in positive AI values. The aerosol index has been validated and analyzed in a number of studies such as those of Gkikas et al. (2016), Koukouli et al. (2006), de Graaf et al. (2005). AI was chosen for the present study instead of the Aerosol Optical Depth (AOD), mainly because AI is a measure of the absorption of UV radiation by aerosols while AOD is a measure of the attenuation of the direct solar beam. Furthermore, AOD in the UV is available since 1992 from only a very limited number of ground stations (e.g., Cheymol and De Backer (2003)). However, changes of AI are strongly correlated with changes in AOD, i.e. higher (more positive) values of AI denote higher AOD while lower (more negative) AI denotes lower AOD, as shown in Koukouli et al. (2006). The AI values used in the present study have been derived from measurements performed by three different satellite instruments, namely the TOMS/Nimbus7 (October 1978 to May 1993), TOMS/EarthProbe (July 1996 and December 2006) and OMI/Aura [October 2004–to date]. The Total Ozone Mapping Spectrometer (TOMS) instrument, designed and developed by NASA, is a downward nadir viewing spectrometer that measures backscattered Earth radiances at six discrete ultraviolet wavelengths (312–380 nm for TOMS/Nimbus-7 and 308–360 nm for TOMS/EP (Krueger et al., 1998)). The total ozone data are derived from the backscattered UV reflectance by a dedicated TOMS retrieval algorithm (e.g., Ziemke et al. (2005)). AI estimates from these sensors have been downloaded from the publicly available NASA GSFC ftp location, ftp://toms.gsfc.nasa.gov/pub/. The Ozone Monitoring Instrument, OMI, has been flying on board the Aura spacecraft since 2004 (Levelt et al., 2006). OMI derives its heritage from NASA's Total Ozone Mapping Spectrometer (TOMS) instrument and the European Space Agency (ESA) Global Ozone Monitoring Experiment (GOME) instrument on the ERS-2 satellite. The wealth of atmospheric composition information provided by OMI is unparalleled (Levelt et al., 2018), with the instrument prominently featured in all ozone trend and ozone recovery studies (e.g., Weber et al. (2017) and references therein). In this study, AI has been extracted for selected locations from the Aura Data Validation Center, https://avdc.gsfc.nasa.gov/.
In the case of the aerosol index, the absolute (not the relative ones as for spectral UV radiation and ozone) annual mean anomalies are used in the study. The mean values of the overpasses for each day were averaged for each month, if at least 15 days were available. The monthly climatological values were subtracted from the monthly averages to derive the monthly absolute anomalies, which in turn were used to derive the annual anomalies. As discussed in Li et al. (2009), the datasets from the three different satellite instruments are reliable and consistent with each other for the periods 1980–1993 (N7-TOMS), 1996–1999 (EP-TOMS), and 2004–present day (OMI).
2.4 Clouds
Monthly total cloud cover data is retrieved from the MERRA-2 reanalysis (Gelaro et al., 2017) for the time period 1991–2016. The Modern-Era Retrospective analysis for Research and Applications version 2 (MERRA-2) is a NASA atmospheric reanalysis for the satellite era using the Goddard Earth Observing System Model, Version 5 (GEOS-5) with its Atmospheric Data Assimilation System (ADAS), version 5.12.4. The MERRA project focuses on historical climate analyses for a broad range of weather and climate time scales and places the NASA EOS suite of observations in a climate context. MERRA-2 was initiated as an intermediate project between the aging MERRA data and the next generation of Earth system analysis envisioned for the future coupled reanalysis. The spatial resolution of MERRA-2 reanalysis is 0.5° × 0.625° (∼50 × 50 km2 at mid-latitudes). In this study, the monthly cloud cover data has been extracted for selected locations from the NASA Giovanni data visualization base (https://giovanni.gsfc.nasa.gov/Giovanni). The monthly climatological values were subtracted from the monthly averages to derive the monthly anomalies, which in turn were used to derive the annual anomalies and finally the annual percentage anomalies by dividing with the annual mean.
2.5 Surface reflectivity
In the present study data from the MERRA-2 reanalysis are used to calculate changes in the surface reflectivity. Monthly surface reflectivity data has been extracted for selected locations from the Giovanni data visualization base https://giovanni.gsfc.nasa.gov/Giovanni, for the time period 1991–2016. As for the case of cloud cover, first the monthly climatological values were subtracted from the monthly averages to derive the monthly anomalies, which in turn were used to derive the annual anomalies and, finally, the annual percentage anomalies were calculated by dividing by the annual mean.
3 Results and discussion
The annual mean anomalies of the 307.5 nm daily noon irradiance, the daily mean total ozone, the AI, and the total cloud cover are presented in Fig. 1 for Canada, Europe, and Japan. Although analysis of the surface reflectivity was performed, these results are only discussed and not shown in Fig. 1. The detected trends are listed in Table 2. The trends for each individual station are listed in Table 3. Although the detected trends for total ozone, AI and clouds are very similar for the three regions, this is not the case for the UV-B irradiance.
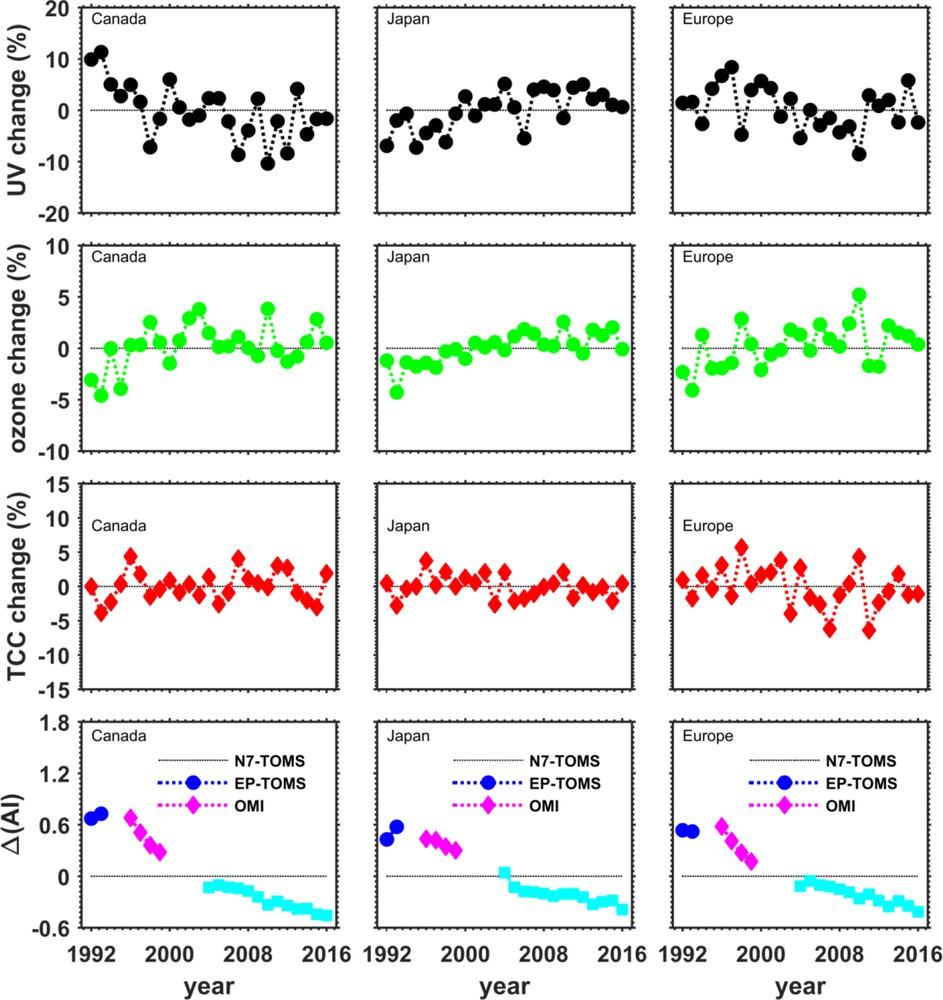
Annual mean anomalies of the all-skies irradiance at 307.5 nm (first), the total ozone (second), the Total Cloud Cover (TCC) (third), and the AI (fourth row) over Canada (left), Japan (middle), and Europe (right column).
Long-term trends of the irradiance at 307.5 nm, the total ozone and the TCC in % per year, and the AI in absolute units, for Canada, Japan, and Europe.
307.5 nm irradiance (Change %) |
Total ozone (Change %) |
TCC (Change %) |
AI (Absolute Change) | |||
IP | NP | IP | NP | IP | IP | |
Canada | −0.43 ± 0.13 | −0.29 ± 0.13 | 0.11 ± 0.06* | 0.04 ± 0.06* | 0.03 ± 0.06* | −0.05 ± 0.00 |
Japan | 0.33 ± 0.08 | 0.32 ± 0.10 | 0.15 ± 0.03 | 0.13 ± 0.03 | −0.04 ± 0.05* | −0.04 ± 0.00 |
Europe | −0.17 ± 0.12* | −0.19 ± 0.14* | 0.14 ± 0.05 | 0.08 ± 0.06* | −0.12 ± 0.08* | −0.04 ± 0.00 |
* The asterisk denotes a non-statistically significant trend at the 95% confidence level. For the 307.5 nm irradiance and total ozone, the trends have been derived including (IP) and not including (NP) the years 1992 and 1993 in the analysis, while for TCC and AI the differences between the two cases are very small, thus only the IP trends are presented.
Long-term trends of the irradiance at 307.5 nm, the total ozone, the surface reflectivity, and the TCC in % per year, and the AI in absolute units, for individual stations in Canada, Japan, and Europe.
Region | Station | 307.5 nm irradiance (Change %) |
Total ozone (Change %) |
TCC (Change %) |
Surface Reflectivity (Change %)) | AI (Absolute Change) |
Canada | Churchill | −0.34 ± 0.18* | 0.06 ± 0.08* | 0.00 ± 0.10* | −0.46 ± 0.15 | −0.07 ± 0.01 |
Edmonton | −0.29 ± 0.15 | 0.09 ± 0.07* | 0.00 ± 0.12* | −0.01 ± 0.25* | −0.06 ± 0.00 | |
Saturna Island | −0.65 ± 0.17 | 0.11 ± 0.07* | 0.09 ± 0.13* | 0.05 ± 0.06* | −0.04 ± 0.00 | |
Japan | Naha | 0.30 ± 0.13 | 0.17 ± 0.03 | −0.04 ± 0.20* | 0.03 ± 0.02* | −0.05 ± 0.00 |
Sapporo | 0.11 ± 0.12* | 0.17 ± 0.03 | −0.15 ± 0.12* | −0.24 ± 0.12 | −0.03 ± 0.00 | |
Tateno | 0.58 ± 0.12 | 0.10 ± 0.04 | −0.05 ± 0.18* | 0.00 ± 0.02* | −0.05 ± 0.00 | |
Europe | Uccle, Belgium | −0.06 ± 0.17* | 0.26 ± 0.06 | −0.22 ± 0.10 | 0.03 ± 0.02* | −0.04 ± 0.00 |
Reading, UK | −1.20 ± 0.27 | 0.07 ± 0.08* | −0.15 ± 0.13* | 0.10 ± 0.05* | −0.04 ± 0.00 | |
Sodankylä, Finland | −0.32 ± 0.20* | 0.08 ± 0.07* | −0.21 ± 0.11 | −0.46 ± 0.11 | −0.04 ± 0.00 | |
Thessaloniki, Greece | 0.34 ± 0.12 | 0.13 ± 0.06 | 0.11 ± 0.25* | −0.08 ± 0.06* | −0.05 ± 0.00 |
* The asterisk denotes a non-statistically significant trend at the 95% confidence level.
Over all three regions, very low ozone was recorded in 1992 and 1993, possibly as a result of the eruption of Mount Pinatubo (Aquila et al., 2013; Randel et al., 1995). In the case of Canada, the very low ozone in conjunction with the low TCC in these years led to very high UV-B irradiance (∼10% above the climatological mean). Thus, different conclusions may be extracted depending on whether these years are included or not in the analysis of the UV-B irradiance and ozone (i.e. the mean UV-B decrease is −0.29%/year vs. −0.43%/year, while the ozone increase is 0.04% vs. 0.11%). The corresponding differences in the trends of UV-B irradiance are less significant for Europe and Japan, as shown in Table 2.
Although ozone increases significantly over Europe and Japan, the reduction of the UV-B irradiance over Europe is not statistically significant (unless 1992 and 1993 are excluded from the analysis), while over Japan a statistically significant positive trend in UV-B has been detected. Over these regions, both AI and TCC decrease, though only the changes of the former were found to be statistically significant. Thus, the long-term changes in UV-B irradiance can be attributed mainly to the changes of AI. As discussed in the following, the role of changes in surface reflectivity is also not negligible over higher latitudes.
Over Japan, positive trends in UV-B irradiance were observed for all the three stations, indicating that the effect of the AI reduction fully overcomes the effect of ozone recovery. The trends of TCC were found to be very small and statistically insignificant for all stations, while a negative significant trend of surface reflectivity was estimated for Sapporo. It is also evident from Fig. 1 that over Japan the UV irradiance increases faster before the mid-2000s as compared to the period after this point, confirming the findings of Zerefos et al. (2012).
Results of the analyses are more complex over Europe. Over Uccle the mean UV-B change is nearly zero, while over Thessaloniki the UV-B levels increase and over Reading and Sodankylä the UV-B levels decrease. Analysis of clear-sky noon data for Thessaloniki confirms that the long-term changes of UV-B irradiance are mainly driven by changes in aerosol load. As shown in Fig. 2, clear-sky UV-B irradiance increases significantly during the period of study (by ∼ 0.35 ± 0.13%/year), following the decrease of AI. Analysis of satellite data confirms that surface reflectivity is nearly invariable throughout the years over this particular station. Over the higher-latitude stations, changes in ozone are estimated to be the main driver of changes in UV-B, though the role of changes in surface reflectivity and cloudiness cannot be considered negligible, especially for the station of Sodankylä. For this station, a statistically significant reduction of the surface reflectivity by 0.46 ± 0.13%/year has been detected, probably due to a corresponding reduction in snow cover (Derksen et al., 2016). Despite the larger increase in ozone and the negative trend in surface reflectivity over Sodankylä, the negative trends in UV-B irradiance over this particular station are weaker than the corresponding negative trends at the lower-latitude station of Reading. This is possibly due to the significant negative trend in TCC over Sodankylä.

Annual mean anomalies of the clear-sky irradiance at 307.5 nm (upper panel), the total ozone (middle panel), and the AI (lower panel) over Thessaloniki, Greece.
Over Canada, no significant trend in the changes of ozone or cloudiness has been detected, while significant reduction of the surface reflectivity has been found only for the station of Churchill. However, UV-B irradiance decreases significantly over Edmonton and Saturna Island, despite the significant decrease of the AI, which is stronger than the corresponding decrease over Europe and Japan. The stronger reduction of the UV-B irradiance over Saturna Island, compared to the other two Canadian stations, may be again related to the statistically insignificant positive trend in cloudiness over this station. It should be noted at this point that the mean latitude of the Canadian stations is much higher than that of the stations of Japan used in the present study, leading to a relatively stronger effect of ozone compared to the effect of aerosols (e.g., Meleti et al. (2009)). However, it is uncertain whether this is enough to explain the differences in the behavior of UV-B irradiance between these two regions. Additional explanations might be that the high variability of cloudiness, surface reflectivity, and ozone over Canada makes trend detection difficult and the fact that the interaction between UV-B irradiance and all the factors affecting it is very complex. Thus, further investigation is necessary to clarify this result.
4 Summary and conclusions
Data from stations over Europe, Canada, and Japan, where spectral UV-B irradiance is measured since the beginning of the 1990s, were used to study the long-term trends of the irradiance at 307.5 nm for a 25-year period, from 1992 to 2016. Ground-based measurements of total ozone, as well as satellite measurements of the Aerosol Index, the Total Cloud Cover and the surface reflectivity were also used in order to attribute the estimated changes in UV-B irradiance to the corresponding changes in these factors, which are generally considered as the main regulatory factors for the levels of the solar UV-B irradiance that reaches the Earth's surface (Bais et al., 1993; Bernhard et al., 2007).
Over Japan, UV-B irradiance has increased significantly during the past 25 years, possibly due to the corresponding significant decrease in the absorption by aerosols, i.e. changes in the AOD – e.g., Wild (2011) – and the absorption efficiency of aerosols (Kudo et al., 2012). It was found that the greatest part of this increase took place before the mid-2000s, confirming the findings of Zerefos et al. (2012). All three Japanese stations show consistent results.
In Europe, although changes in aerosols are similar over all stations, their effects on UV-B irradiance are more pronounced over central and southern Europe, while at the stations of northern Europe, long-term changes in UV-B irradiance were found to be driven mainly by ozone recovery. This is probably due to the more significant positive trends of ozone, which in conjunction with the lower sun elevation over higher latitudes have a stronger impact on the long-term changes in solar UV-B radiation.
Analysis of the clear-sky irradiance for the station of Thessaloniki shows a continuous increase in UV-B irradiance during the entire period of study, which was stronger during the last decade. These findings are not in agreement with Fountoulakis et al. (2016a), who suggest that the rate of the UV increase weakens after 2006. However, in that study, the trends in UV-B irradiance were restricted to a specific SZA of 64° where attenuation by ozone possibly plays a more significant role. For most of the year, this SZA is larger than the SZA at local noon. A more in-depth study of the long-term changes in aerosols over the station of Thessaloniki should be performed to reveal whether aerosols change differently at different times of the day. This might also explain part of the difference between the two studies.
The UV-B irradiance has been found to increase significantly over Canada, without being able to directly attribute it to the changes of any of the major factors affecting it. This result points to the complexity of the interaction of UV-B radiation with its main regulatory factors. Changes in surface reflectivity were found to be non-negligible regarding their impact on the levels of UV-B irradiance over the high-latitude stations of Churchill, Sapporo, and Sodankylä, confirming the findings of Bernhard (2011) and providing a sign that they might play an important role for the future changes of UV-B irradiance over the Arctic.
Concluding, the present study shows that over the Northern Hemisphere, the long-term changes in the UV-B radiation that reaches the Earth's surface vary greatly over different locations, and that the main drivers of these changes are changes in aerosols and total ozone. Over higher latitudes, part of the observed changes may be attributed to changes of the surface reflectivity and clouds. Since the connection between the changes in UV-B irradiance and changes in the different factors is not clear, efforts to reduce the uncertainties in the measurements and to improve the understanding of the interactions between solar UV radiation and the related geophysical variables are necessary.
Acknowledgments
The ground-based data used in this publication were obtained as part of the WMO Global Atmosphere Watch publicly available via the World Ozone and UV Data Centre (http://woudc.org), and from the EUVDB (http://uv.fmi.fi/uvdb/) database. We would like to acknowledge and warmly thank all the investigators that provide data to these repositories on a timely basis, as well as the handlers of these databases for their upkeep and quality guaranteed efforts. The QBO indexes are from the CDAS Reanalysis data and are the zonally averaged winds at 30 and 50 hPa and taken from over the equator (http://www.cpc.ncep.noaa.gov/data/indices/). The F10.7 cm solar radio flux density, used as proxy of the 11-year solar cycle, was acquired from the Solar and Terrestrial Physics Division (STP) of NOAA's National Geophysical Data Center (NGDC) Website (http://www.ngdc.noaa.gov/stp/stp.html). We further acknowledge the TOMS NASA GSFC team for maintaining the ftp://toms.gsfc.nasa.gov/site as well as the Aura Data Validation Center team for maintaining the http://avdc.gsfc.nasa.gov/site. The monitoring site at Reading, UK, is funded by the UK Department of Environment, Food and Rural Affairs (DEFRA). The Academy of Finland has funded the UV measurements at Sodankylä by the FARPOCC and SAARA projects.