1 Introduction
The origin of volatile elements on Earth can be addressed using the noble gas systematics in oceanic basalts by comparing their isotopic compositions to those of the atmosphere, of the meteorites and of the solar wind. Noble gases (He, Ne, Ar, Kr and Xe) are inert gases, have both radiogenic and non-radiogenic isotopes and can fractionate during physical processes (e.g. diffusion, adsorption…). Therefore, they are excellent tracers of atmosphere formation. If it is clear that a degassing of the mantle occurred to form part of the atmosphere [2,7,42], there is some debate about the possibility of the atmospheric noble gases returning back into the mantle through subduction, masking the primordial rare gas composition. This process has to be understood if one wants to constrain the isotopic composition of the primitive Earth, the nature of the parent bodies and the process of volatile acquisition by terrestrial planets.
The issue of the noble gas subduction in the mantle has been addressed in different ways. Staudacher and Allègre have postulated the existence of a ‘noble gas subduction barrier’ in order to preserve the 129Xe anomalies in the Mid Oceanic Ridge source mantle [43]. Sarda et al. [41], based on the Ar–Pb negative correlation on MORB, have suggested that the ‘high μ’ component (high 206Pb/204Pb) has also an atmospheric 40Ar/36Ar ratio consistent with recycling of altered oceanic crust in the mantle. Sarda has also suggested that the air component observed in MORB and OIB reflects a recycled altered material [39]. More recently, based on xenon measurements on CO2 well gases, in particular the light isotopes of the xenon (124(130Xe), Holland and Ballentine [14] have suggested massive subduction of xenon and of other heavy noble gases in the mantle, in the form of seawater. Indeed, they explain the position of the CO2 well gases in 124-126/130Xe–128/130Xe diagrams (Fig. 1) – close to the air ratios, by a mixture between air and solar end-members (similarly to [8]).
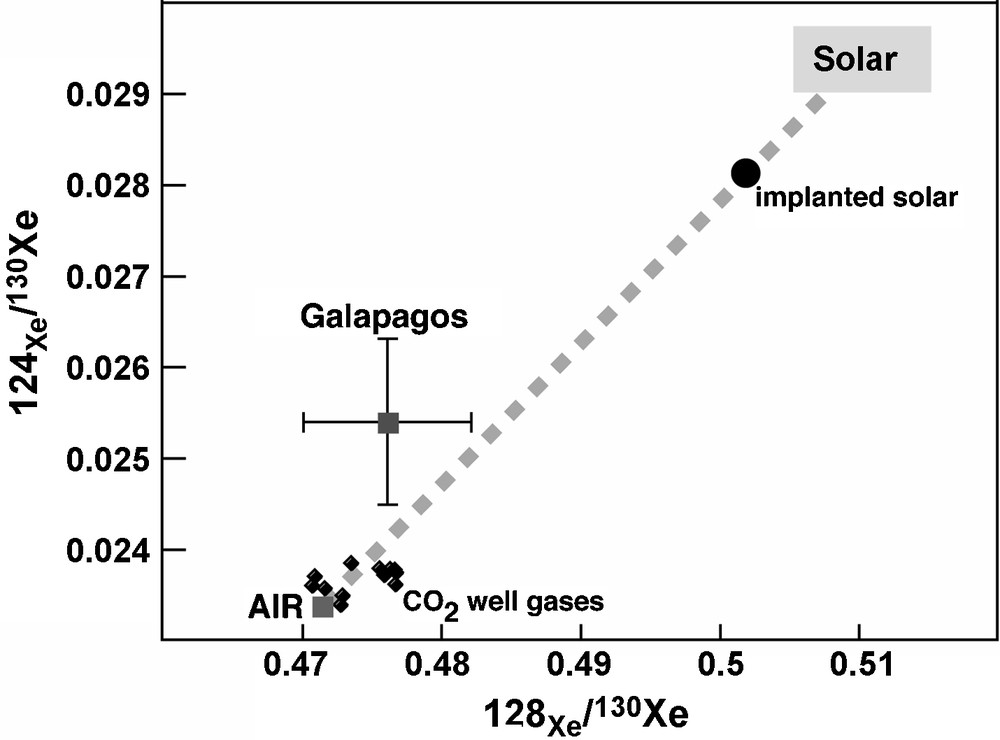
Xenon isotopic diagram showing the CO2 well gases presenting a small proportion of solar xenon [14]. Implanted solar is the composition that will have a material that is exposed to a solar wind. The Galapagos sample has the most primitive neon signature and the xenon corresponds to crushing steps with high 20Ne/22Ne, reflecting little air contamination.
Fig. 1. Diagramme isotopique du xénon représentant des échantillons de puits de CO2 [14]. Une faible proportion de xénon solaire ou de xénon solaire implanté et fractionné isotopiquement pourrait expliquer la différence avec les valeurs de l’air. L’échantillon provenant des îles Galapagos est un verre basaltique sous-marin montrant un rapport 20Ne/22Ne de ∼12,5, et qui a la signature la plus primitive en néon observée dans les basaltes océaniques.
The 38Ar/36Ar ratio can also bring clues on the possible subduction of noble gases in the mantle. The 38Ar/36Ar ratio appears to be atmospheric in both MORB and OIB sources (Fig. 2), even for samples that are uncontaminated by air or seawater (i.e. high neon isotopic ratio) [20,45,46]. The atmospheric value is different from the solar value and may suggest recycling of atmospheric argon in order to give air-like argon in the mantle.
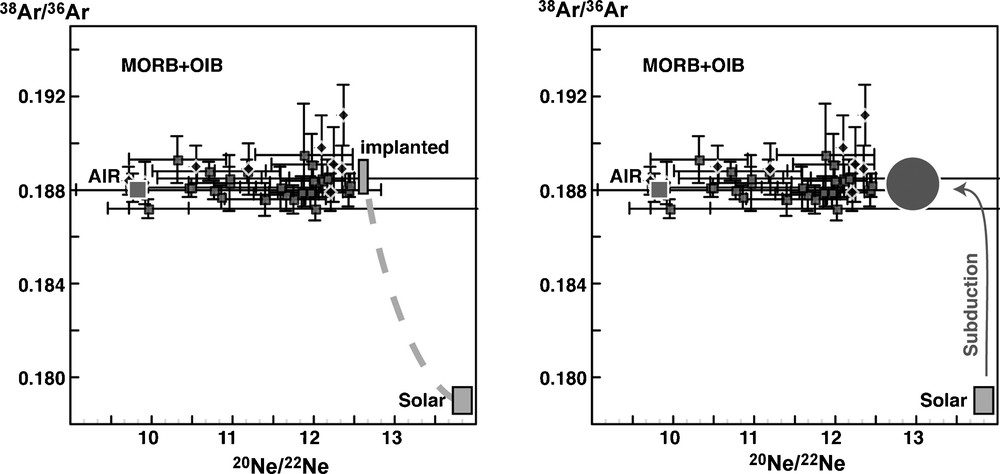
Ne–Ar systematics in oceanic basalts. Two extreme scenarios can be proposed for the origin of the air-like 38Ar/36Ar ratio in MORB and OIB. One is that the parent bodies of the Earth were exposed to a solar wind. Solar wind implantation is fractionating the isotopic ratios [12]. Another hypothesis is the subduction of air argon, after the isotopic fractionation of the atmospheric argon following the major degassing in the Hadean [35]. Data are unpublished data from Raquin and Moreira.
Fig. 2. Systématique Ne–Ar dans les basaltes océaniques. Deux scénarios extrêmes peuvent être proposés pour expliquer le fait que le rapport 38Ar/36Ar est atmosphérique dans le manteau (et non solaire). Un des scénarios est que le rapport atmosphérique reflète la composition de l’argon solaire implanté dans les corps parents de la Terre, avec fractionnement de masse dépendant de la masse [12]. Une autre hypothèse est la subduction massive d’argon atmosphérique, celui-ci ayant été fractionné isotopiquement peu de temps après un évènement majeur de dégazage à l’Hadéen [35]. Les données proviennent de Raquin et Moreira (non publiées).
The use of both radiogenic and stable isotopic ratios of the argon and xenon (40Ar/36Ar and 38Ar/36Ar and 124–128Xe/130Xe and 129Xe/130Xe) should give constraints on the possible noble gas subduction in the mantle. We propose a model of degassing–subduction for these isotopic ratios and demonstrate that argon and xenon subduction appears to be difficult to consider, and that the peculiar noble gas signatures of the Earth probably reflect processes that occurred during the formation of parent bodies.
2 Noble gas isotopic ratios in the mantle and of early Earth (‘stable’ isotopes)
We present here only the case of the non-radiogenic isotopic ratios of the noble gases. The cases of the radiogenic ratios are relatively well known and the discussions about their origin can be found in [3,28,38,44,48–50]. Values of the isotopic ratios are given in Table 1.
Concentration and isotopic ratios of the rare gases in the atmosphere, in the present-day MORB reservoir and in the primitive Earth, assuming that the isotopic compositions were solar after its formation
Tableau 1 Concentrations et rapports isotopiques des gaz rares dans l’atmosphère, dans le manteau source des basaltes de dorsales et dans la Terre primitive, si on suppose que la Terre avait une composition isotopique solaire lors de sa formation
Gas | Atmosphere | MORB source | Solar |
4He | 2.08 × 1019 ccSTP | 10−5 ccSTP/g | |
4He/3He | 722 500 | 90 000 | 2800 |
22Ne | 6.64 × 1018 ccSTP | 2.3 × 10−11 ccSTP/g | |
20Ne/22Ne | 9.8 | 12.6 | 13.80 |
21Ne/22Ne | 0.029 | 0.06 | 0.0328 |
36Ar | 1.24 × 1020 ccSTP | 2.7 × 10−10 ccSTP/g | |
38Ar/36Ar | 0.1880 | 0.1880 | 0.1790 |
40Ar/36Ar | 295.5 | 25,000 | |
84Kr | 2.57 × 1018 ccSTP | 7.9 × 10−12 ccSTP/g | |
130Xe | 1.4 × 1016 ccSTP | 1.5 × 10−13 ccSTP/g | |
124Xe/130Xe | 0.02337 | ∼0.023 | 0.02939 |
128Xe/130Xe | 0.4715 | ∼0.47 | 0.51 |
129Xe/130Xe | 6.5 | 7.5 | 6.27 |
It is relatively clear now that the 20Ne/22Ne ratio is solar-like in the mantle, different from the atmospheric value, although its precise value is not known [15,23,27,29,30,40]. This difference between the mantle and the atmosphere is certainly due to an escape of the neon from the atmosphere with mass fractionation [17]. To enter in more details, there is a debate on the exact 20Ne/22Ne ratio of the mantle. It has been suggested that the neon ratio is similar to the solar wind value (=13.84 [12]) [13,48]. On the other hand, some authors suggest that the 20Ne/22Ne ratio is similar in the mantle to a component called ‘neon B’ (∼12.5) [6] that is sampled in gas-rich meteorites and in lunar soils [4,44–46]. The origin of this component is now clear since the samples returned from the Genesis mission have shown that mass fractionation occurs during solar wind implantation, with a deeper implantation of the heavier isotope 22Ne (or 38Ar) [12]. Assuming this fractionation during implantation and sputtering of the surface, one can easily show that after a short time of irradiation, a steady state is obtained with a 20Ne/22Ne ratio of ∼12.6, similar to the ‘neon B’ component. Therefore, it appears that the process of incorporation of neon in parent body precursors was solar irradiation, probably during the T-Tauri phase of the Sun, and that the solubilisation of solar neon in the mantle during a magma ocean episode was not necessary.
Atmospheric 38Ar/36Ar (∼0.188) is very different from the solar ratio (0.179 [5,36]) either resulting from fractionated implantation in parent bodies [25,44] or from hydrodynamic escape with mass fractionation of the atmosphere with initially a solar composition [17,33]. However, in this last case, the mantle had to be solar also, whereas it appears that it has presently an air-like signature [20,45,46]. This could either suggest a subduction of atmospheric argon in the mantle or reflect the initial argon composition of the parent bodies (‘argon B’).
There are only few precise 124–128Xe/130Xe measurements in mantle-derived rocks due to very low abundance of these isotopes (∼10−17 g/g). Only CO2 well gases allow relatively precise measurements. [8] and [14] have produced data of quality showing small excesses relative to air of the 124–128Xe/130Xe ratios (Fig. 1). These data suggest a solar xenon contribution in the mantle. It has been suggested that the Earth was initially solar for the isotopic compositions and that the atmosphere was isotopically fractionated by a hydrodynamic escape [34]. Subduction of this atmospheric xenon would then have modified the primitive mantle composition.
We have seen that both argon and xenon isotopic ratios in the mantle can be interpreted as the result of subduction of atmospheric argon and xenon, previously isotopically fractionated by a hydrodynamic escape or by a late veneer. We, therefore, propose to evaluate this hypothesis by developing the following model of degassing/subduction of Ar and Xe.
3 Degassing–subduction model for the noble gases
We will assume that subduction started 4.4 Ga ago, after the major geological event that modified the atmosphere (hydrodynamic escape, large impacts, late veneer…) Fig. 3. We therefore assume a simple first-order degassing process, associated with a subduction of atmospheric rare gases. The hypotheses for the developed model are the following:
- • Fractions gAr and gXe of the present argon and xenon budgets of the atmosphere are in the mantle after the first stage of degassing. This first stage of degassing is certainly due to impacts (Moon formation?) or to the presence of a magma ocean and is required by the 129I–129Xe or 244Pu–136Xe systematics [3]. We now consider the second stage of degassing, which can be called the ‘plate tectonic degassing’, reflecting the oceanic crust formation, and then magma degassing to the atmosphere.
- • The 38Ar/36Ar and the 124–128Xe/130Xe ratios of the atmosphere are fractionated compared to solar value – and the mantle value – due to a hydrodynamic escape or a late veneer with material having an atmospheric composition (comets?) (e.g. [9,17,24]).
- • The mantle was initially solar for the 38Ar/36Ar and the 124–128Xe/130Xe ratios. Noble gas compositions vary with time due to subduction of atmospheric argon and xenon.
- • The continental crust was extracted continuously over time. This concerns the 40K extraction of the mantle. The crust extraction time is given by the present concentration in the MORB source compared to the bulk Earth concentration, which reflects the depletion of the mantle. We neglect the iodine extraction.
- • The present degassing rate is given by the helium isotopes. The 3He flux at ridges is 1000 mol/year [10].
- • The subduction term is proportional to the total quantity in the atmosphere. It has an isotopic composition similar to the atmospheric one (e.g. not fractionated isotopically).
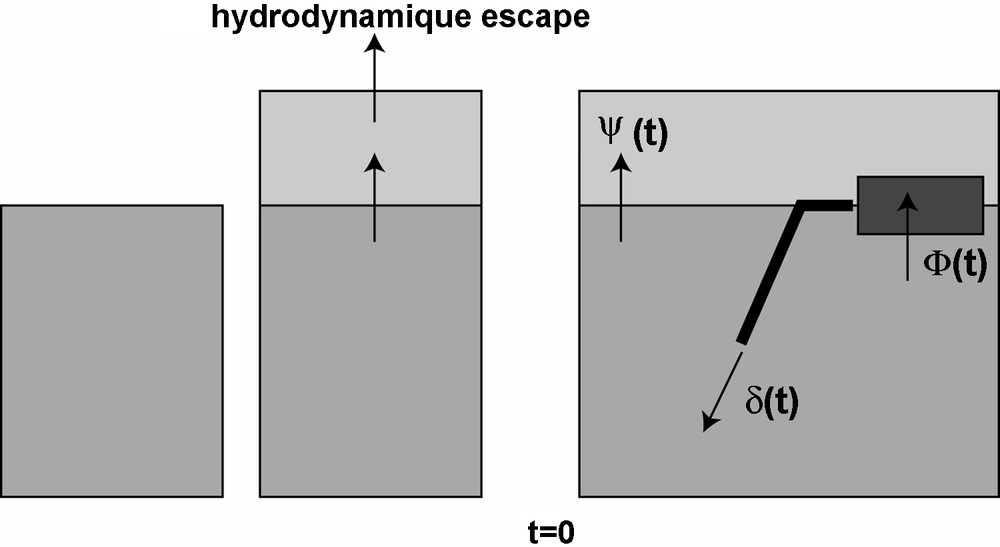
Sketch showing the proposed model. The basic ideas are that a mass fractionation occurs just after the first stage of mantle degassing (for example by a hydrodynamic escape, increasing the 38Ar/36Ar, and decreasing the 124-128Xe/130Xe ratios, of the atmosphere from the solar values to the present-day ratio. Following this fractionation, plate tectonics begin and then atmospheric rare gases can be subducted. In parallel, continental crust pumps the K.
Fig. 3. Schéma illustrant le modèle proposé dans cet article. L’idée est qu’un fractionnement de masse s’est produit juste après le dégazage massif du manteau (par exemple grâce à un processus d’échappement hydrodynamique), augmentant ainsi le rapport 38Ar/36Ar et diminuant les rapports 124-128Xe/130Xe de l’atmosphère à partir d’une valeur solaire. Après ce fractionnement, la tectonique des plaques commence et permet un recyclage de gaz rares atmosphériques dans le manteau. En parallèle, la croûte continentale pompe le K.
The equations describing the evolution of the different isotopes are the following:for a stable isotope in the mantle
The initial quantity of rare gas isotopes in the mantle left after the first degassing event is supposed to be a fraction g of the total atmospheric quantity. The total primitive budget of the atmosphere is assumed to be the same as today. The differential equations were solved using Matlab©.
For xenon, since 129I has a short half-life (17 Ma), it is important to know the time of the first degassing event. The 129I in the mantle at the moment T1 of the first degassing event is where . T1 is taken as 100 My for the calculations (∼degassing at 4.47 Ga). The iodine content of the primitive mantle is 10 ppb [22]. The 129Xe/130Xe ratio of the mantle when the model start, is
In the present model, since we start from after the first degassing step, which is a ‘tectonic’ stage, Ψ(t) is supposed to follow the potential mantle temperature, given in Labrosse and Jaupart [21], interpreted in terms of mean melting rate using the following equation: [19]. To determine the present-day Ψ, one can use helium. For a 3He concentration in the mantle of 10−10 ccSTP/g [28] and for a degassing mantle size of 1027 g (e.g. upper mantle), the degassing constant Ψactual is 2 × 10−10 an−1· Φ(t) is a constant in this model and is determined by the fact that the present-day MORB K concentration is 4 times lower than the primitive K content of the bulk Earth. Therefore, Φ(t)=Φ = −ln(1/4)/4.5 × 109 = 3.1 × 10−10 yr−1. For reasons of mass conservation, δ(t) has to follow the same law (versus time) than Ψ(t).
4 Results and discussion
Examples of results are represented on Figs. 4 and 5. A model of mantle degassing without any atmospheric recycling is able to explain the 40Ar/36Ar ratio with a remaining argon fraction in the mantle of 0.65% after the first stage of degassing. It is, however, not possible to get the present-day 40Ar/36Ar ratio (24 000) if there is a recycling with such a remaining fraction. With a remaining fraction g of 10−4 and a present-day δ of 0.55 × 10−12 yr−1, it is possible to obtain both the 38Ar/36Ar and the 40Ar/36Ar of the present-day MORB source (after 4.4 Ga of subduction). The value of δ of 0.55 × 10−12 yr−1 corresponds to a total 36Ar of subducted argon in the convecting mantle equal to ∼7 × 107 ccSTP/yr. Higher subduction proportion leads to 40Ar/36Ar ratios of only few thousands, much lower than the MORB present-day value. Higher residual fraction of 36Ar left after the first degassing event leads to a lower 38Ar/36Ar ratio than that of the air.
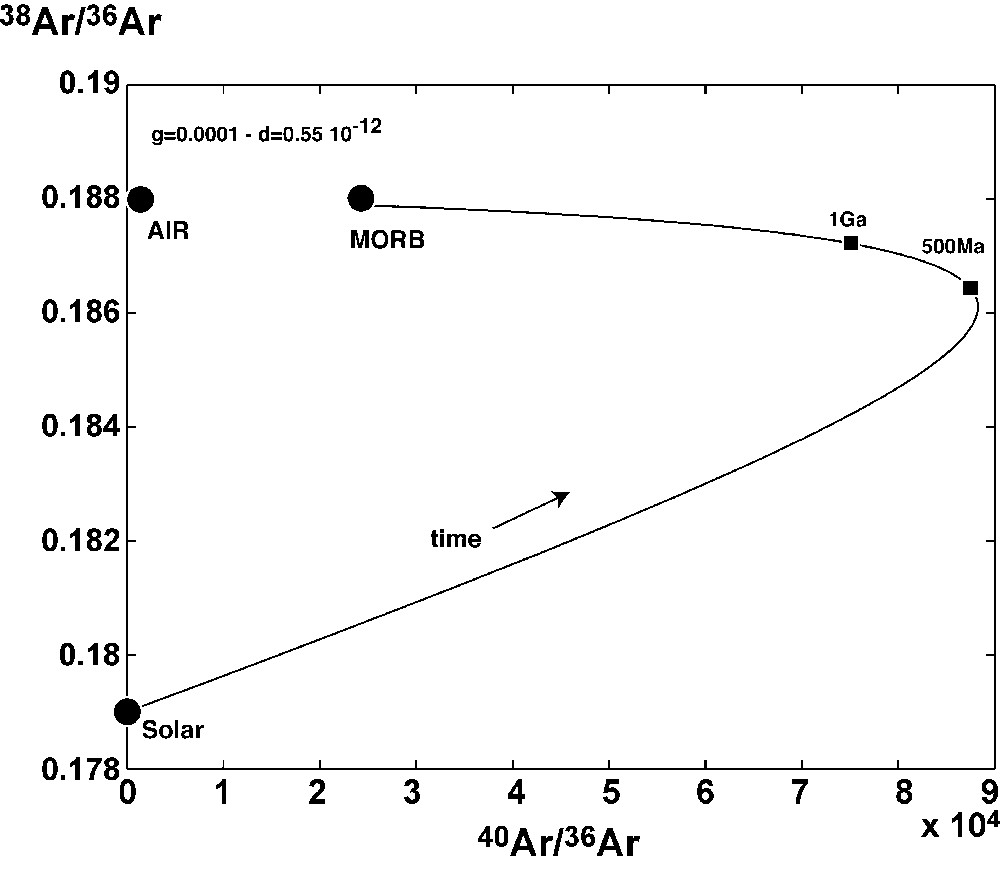
Example of mantle evolution with atmospheric noble gas recycling. This was calculated using the following parameters: Ψ = 2 × 10−10 yr−1, g = 0.01% (actual). The subduction constant δ is 0.55 × 10−12 yr−1.
Fig. 4. Exemple d’évolution de la composition isotopique de l’argon dans le manteau, avec recyclage de gaz atmosphériques. Dans cet exemple, les paramètres suivants ont été obtenus pour expliquer la composition isotopique actuelle du manteau source des basaltes de dorsale : Ψ = 2 × 10−10 an−1, g = 0,01%, δ = 0,55 × 10−12an−1 (valeurs actuelles).
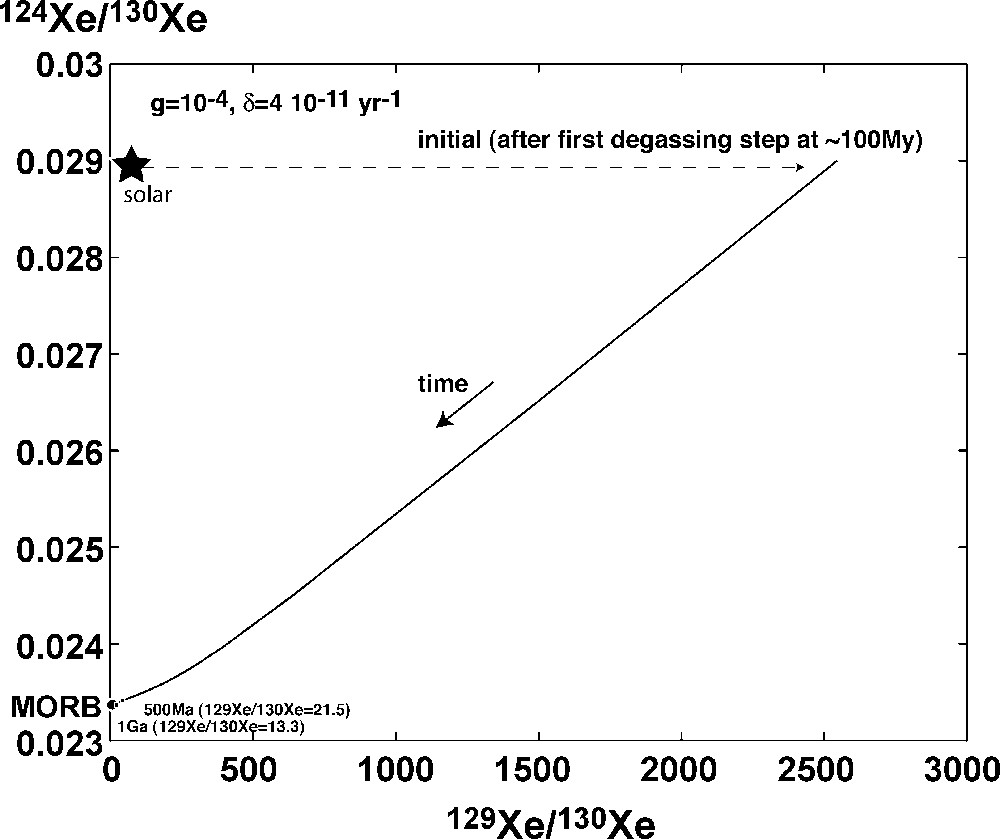
Result of the subduction model for xenon with a remaining fraction of 10−4 for the 130Xe after the first degassing event (here at 100 My after CAI formation) and a present-day fraction of the total atmospheric budget that is subducted of 4 × 10−11 yr−1. Note that the 129Xe/130Xe ratio was, in this model, higher in the Archean (∼13–21).
Fig. 5. Résultats du modèle de subduction de xénon atmosphérique dans le manteau. La même proportion de xénon restant dans le manteau après le grand événement de dégazage (100 Ma après les CAI) que pour l’argon est utilisée (10−4). Pour expliquer les rapports isotopiques, un taux de subduction actuel de 4 × 10−11 an−1 est nécessaire. Notez que le rapport 129Xe/130Xe à l’Archéen est compris entre 13 et 21.
The results are identical for the xenon in the sense that an important first-step degassing is required. Fig. 5 shows the 124Xe/130Xe versus 129Xe/130Xe as a function of time. It is possible to get to present-day 129Xe/130Xe for a fraction of remaining 130Xe in the mantle of 10−4, the value required for argon. To get MORB-like 129Xe/130Xe ratio (∼8), a minimum present-day δ of 4 × 10−11 yr−1 is necessary, which corresponds to a subduction flux of 6 × 105 ccSTP/yr of 130Xe. It is important to note that the 129Xe/130Xe ratio in the Early Archean is of a few hundreds. 3.5 Ga ago, this ratio was ∼13.
The flux of subducting oceanic crust is ∼5 × 1016 g/yr. The mass flux of subducting sediment is ∼10 times lower. Staudacher and Allègre have estimated the mean concentration of 36Ar and 130Xe in altered oceanic crust and sediments [43]. They obtained 36Ar = 2.7 × 10−8 ccSTP and 130Xe = 4.3 × 10−11 ccSTP for oceanic sediments and 36Ar = 3.2 × 10−9 ccSTP and 130Xe = 7.4 × 10−12 ccSTP for the oceanic crust. Without loss from the slab, the total flux of subduction 36Ar would be ∼3 × 108 ccSTP/yr and ∼6 × 105 ccSTP/yr for 130Xe (if all the oceanic crust is similar to altered pillows).
From our calculations, we estimated a minimum flux of 36Ar and 130Xe of 7 × 107 and 6 × 105 ccSTP/yr, respectively. This suggests that the dehydration, and the associated loss of noble gases from the subducting slab, or from the mantle wedge if this one is re-injected in the convecting mantle, is small (∼80% for Ar and 0% for Xe) and that an important proportion of the rare gases from the oceanic crust and the sediments has to be re-injected into the mantle if one wants to ‘mask’ the solar argon and xenon primordial composition.
4.1 Comparison with Archean rocks
One of the major results of this model is that both the 40Ar/36Ar and the 129Xe/130Xe were much higher in the Archean than today. There are only few noble gas data on Archean rocks due to alteration and radiogenic production problems. Diamonds, cherts and fluid inclusions in hydrothermal deposits are the only few samples with mantle-like signatures [11,16,31,37]. For the 129Xe/130Xe ratio, it has been argued that it can derive from neutron capture by 128Te, giving 129I, and then decaying into 129Xe. However, Moreira [26] has shown than in this case, due to the double beta decay of the 130Te (having the same abundance as 128Te), the 129Xe/130Xe ratio will decrease, rather than increase. Therefore, the use of the 129Xe/130Xe ratio is appropriate for Archean samples.
As we said, there are only few reliable noble gas data on Archean rocks. Archean cherts from Australia have been analyzed for xenon isotopes [37]. Although it is not clear how cherts acquired a mantle signature for the xenon, these samples show a 129Xe/130Xe of 7.5 and fall on the MORB line on a 129Xe/130Xe-136Xe/130Xe isotopic diagram. 128Xe/130Xe are not precise enough to distinguish between air and solar compositions. Fluid inclusions in 3.5-Ga hydrothermal deposits from the North Pole area (Pilbara Craton, Australia) have been analyzed [31]. Using the correlation between 40Ar/36Ar and the SO42−/Na+, these authors were able to derive the 40Ar/36Ar of the mantle at this epoch around 16 000. However, the 38Ar/36Ar ratios are not published and cannot be used to constrain the possibility of argon subduction (Fig. 4). Although the ages of diamonds are questionable, it has been argued that they may be as old as 3 Ga and certainly older than 1 Ga. All the diamonds analyzed so far do not show any 129Xe/130Xe ratio higher than ∼8 [11,16]. They also show atmospheric 128Xe/130Xe.
An anorthosite from Greenland, dated from 3.5 Ga, has been analyzed for xenon and also show MORB-like xenon with 129Xe/130Xe of 7.02 ± 0.09 and a 136Xe/130Xe of 2.27 ± 0.02 [18]. The 131Xe/130Xe and 132Xe/130Xe ratios show clear excesses compared to air, suggesting little atmospheric contamination. Again, it seems that the 129Xe/130Xe ratio in the Early Archean was not higher than the present-day MORB ratio.
The few precisely measured 128Xe/130Xe ratios do not show a solar signature (the anorthosite from Greenland has a ratio of 0.477, similar to the present-day air ratio, as well as the diamonds).
From all these results on Archean samples, deriving from a mantle source, it appears that the 129Xe/130Xe ratio was not very different from the present-day MORB ratio, suggesting that the model of xenon subduction is not appropriate.
4.2 Subduction of noble gases in the OIB source?
Our calculation was applied to the MORB source. However, it has been shown that the OIB source also has atmospheric 38Ar/36Ar argon [45,46]. The case of the 124–126Xe/130Xe ratios is not solved yet due to the inadequate precision of the data. However, unpublished data from Galapagos, the hotspot having the most primitive neon on Earth, do not show clear 128Xe excess compared to air. Iceland samples do not show excesses either [45]. Therefore, we will have to admit that the OIB sources also have argon and xenon stable isotopic ratios similar to the air ratio (and not solar). The subduction of atmospheric rare gases in the OIB source is also a possibility. The model we have developed could apply to OIB. Nevertheless, if one assumes the OIB source is less degassed than the MORB source by a factor 10 or 100 [1], it appears much harder to subduct atmospheric noble gases in these sources (it requires very important subduction fluxes).
5 Conclusions
The subduction of noble gases in the mantle, although possible under some extreme hypothesis (e.g. high proportion of subduction, important first-stage degassing), does not explain the apparent atmospheric noble gas signatures in the mantle, especially in the oceanic island source. If subduction of atmospheric noble gas occurred, the 129Xe/130Xe ratios and the 40Ar/36Ar in mantle derived rocks would have been higher in the Archean than the present-day MORB source value. This is not observed in Archean rocks deriving from the mantle. Therefore, the scenario the most probable is that the rare gas isotopic fractionation (leading from solar to air ratios) occurred before or during Earth's accretion, either by solar wind implantation (Ne, Ar) or by adsorption (Kr, Xe) on the parent bodies.
Acknowledgments
The authors thank Marc Chaussidon, Bernard Marty, Philippe Sarda and Stéphane Labrosse for discussions. This is IPGP contribution number 2278.