1 Introduction
The West Siberian sedimentary basin is one of the world's largest oil and gas bearing basins. Hundreds of oil and gas fields have been discovered here, and several billion tons of oil and several trillion cubic meters of gas produced. In the West Siberian basin area, over 200,000 wells have been drilled and millions of seismic line kilometers shot. It should seem that the sedimentary cover geology of this basin is already well studied. However, 3D seismic surveys have allowed one to reveal a wide spread of a special type of tectonic dislocations – a system of relatively low-relief strike-slip faults within the basement section – that are the cause of complex folding and faulting in the overlying sedimentary cover holding hydrocarbon deposits. Strike-slip faults are well known and thoroughly described in hundreds of publications. However, we are not aware of any other linear strike-slip fault systems such as those encountered in West Siberia, practically similar to physical models, where they are indeed numerous. It is obvious here that the basement itself, in addition to the sedimentary cover, is impacted by the strike-slip motion. Therefore, we believe that observations highlighted in this article would be of interest to the specialists of tectonics and tectonophysics, as well as to geologists involved in the search for, exploration and development of hydrocarbon deposits.
2 General characteristic of the study area
The West Siberian oil and gas bearing basin (Fig. 1) covers an area of over 2.6 million sq. km. The basin geology is highlighted in numerous publications, but in discussing the issues of this article special mention should be made of fundamental works by Kontorovich et al. (1981), Skorobogatov et al. (2003), Surkov and Zhero (1981) and Vyssotski et al. (2006). The crystalline basement of the West Siberia Basin is overlain by a severely dislocated and partly metamorphosed Paleozoic sedimentary pile intersected by effusive rocks. This series is in turn overlain by a platform-type perfectly persistent Meso-Cenozoic clastic cover, which is hundreds of meters thick at the basin edges and up to a 2–5 km in thickness at the central part of the basin. The sedimentary cover thickness increases from south to north. The Jurassic-Cretaceous part of the sedimentary cover hosts most if not all hydrocarbon accumulations discovered so far.
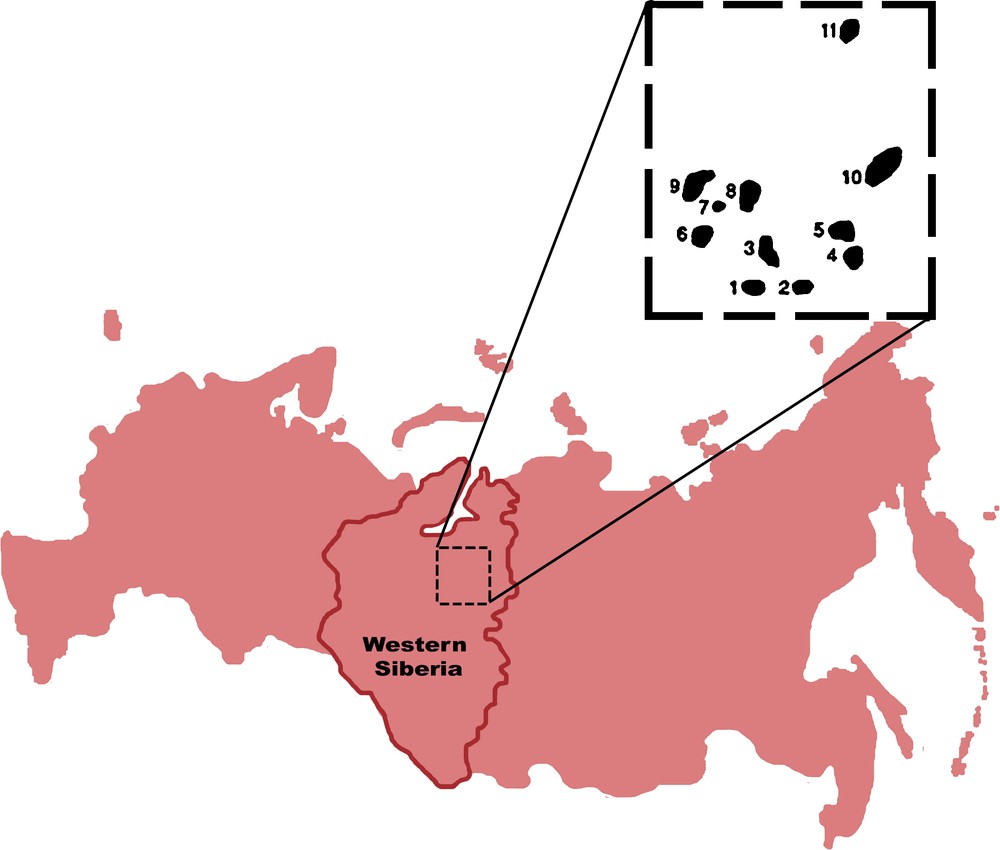
West Siberian oil and gas bearing province. Dashed rectangle outlines the study area. Fields discussed in this article are numbered as follows: 1. Novogodneye. 2. Yarayner. 3. Yetypur. 4. Kharampur. 5. North-Khrampur. 6. Komsomol. 7. Metelnoye. 8. Gubkin. 9. North-Komsomol. 10. Chasel. 11. Russkoye.
Province de Sibérie occidentale renfermant pétrole et gaz.
The study area is located in the central part of the basin outlined with a dashed rectangle in Fig. 1 where numbered black-color spots indicate geologic highs (oilfields) under discussion in this article. A thorough paleostructure analysis shows that numerous highs of the 1st, 2nd and 3rd order have a complex development history and are classified as two types – old structures with intermittent growth episodes inherited from the basement, and newly-formed inversion features in the sedimentary cover horizons.
3 Strike-slip fault structures (SSFS)
3D seismic surveys completed on a large number of structures helped to identify deformations in the sedimentary cover that suggested the presence of strike-slip faults in the underlying basement. The strike-slip faults are expressed in the sedimentary cover as systems of en-echelon downthrows and upthrows over the basement strike-slip fault sutures. The en-echelon systems complicate crestal parts of the highs and flanks and periclinal parts of the structures, as well as interstructure zones. Let us consider the strike-slip fault manifestations using the two-dome Yetypur linear high (2nd order structure) as an example. A large hydrocarbon field is associated with the Yetypur linear high, which was studied in detail with 3D seismic profiles and is therefore a perfect site to study the overall structural grain of strike-slip zones. Fig. 2 shows dip maps for a series of Jurassic and Cretaceous horizons, while Fig. 3 shows three east-west seismic profiles from a 3D cube that cross the northernmost and highly faulted dome. Faults imaged in the seismic profiles and mapped in the sedimentary cover are recognized as shallow accommodations of deeper basement faults with numerous structural characteristics such as en-echelon faults that radiate out in a fan-like manner from a narrow zone at the basement top, en-echelons folds, linear troughs recording episodes of pre-strike-slip fault extension over the main basement strike-slip fault. The faults in the sedimentary beds extend in a regular manner from the lowest sedimentary ones above the basement (3500 m) as far up as the base of the Quaternary (150 m), the entire sedimentary cover thus documenting this deformation process.
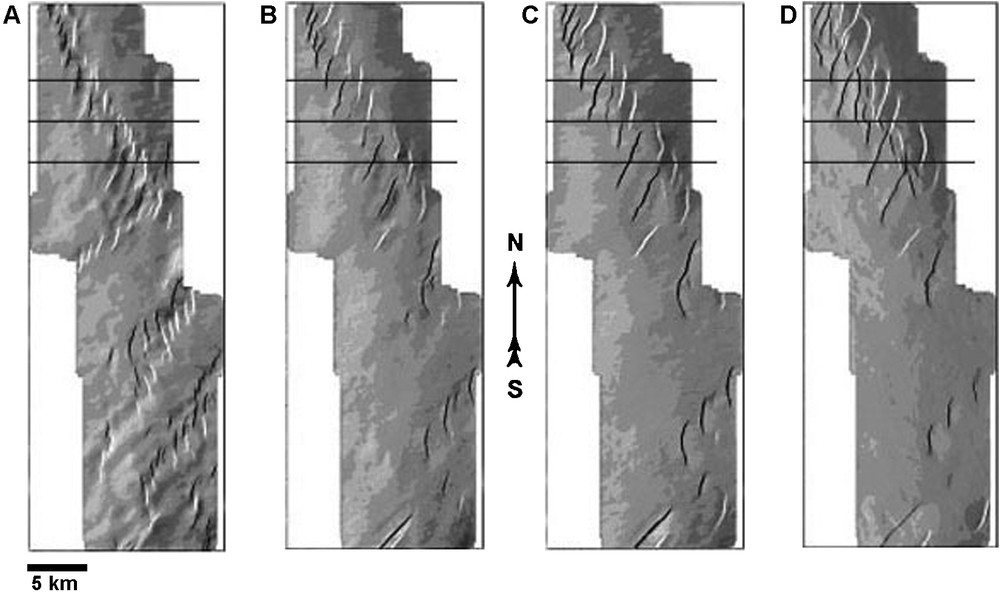
Dip maps for Jurassic and Cretaceous reflectors in the Yetypur area. The maps show en-echelon fault systems at different stratigraphic levels. А. Top of the Jurassic (Horizon B). B. Lower Cretaceous (Horizon ВР12). С. Middle Cretaceous (Horizon РК1). D. Top of Upper Cretaceous (Horizon C). Three east-west lines on dip maps show positions of seismic profiles presented in Fig. 3.
Cartes de pendage pour les réflecteurs jurassiques et crétacés de la zone de Yetypur. Les cartes montrent les systèmes de failles en échelon à différents niveaux stratigraphiques. A. Sommet du Jurassique (Horizon B). B. Crétacé inférieur (Horizon PK1). C. Crétacé moyen (Horizon BP 12). D. Sommet du Crétacé supérieur (Horizon C). Trois lignes est-ouest sur les cartes de pendage indiquent les positions des profils sismiques sur la Fig. 3.

East-west vertical seismic sections (from a 3D cube) (volume) passing through the northern dome in the Yetypur area. Fault systems are seen to disrupt practically the entire sedimentary section from the basement (Horizon A) to base of the Quaternary (the tie of other horizons is shown in Fig. 2). A horizontal reflection at 0.95 s was formed at the base of a Cenomanian gas deposit.
Sections verticales sismiques est-ouest (à partir d’un cube 3-D) (volume) traversant le dôme nord de la zone de Yeti-Pur. On voit que les systèmes de failles affectent pratiquement l’ensemble de la couverture sédimentaire depuis le soubassement (Horizon A) jusqu’à la base des dépôts quaternaires (Le calage d’autres horizons est indiqué sur la Fig. 2).Une réflexion horizontale à 0,95 s’est formée à la base d’un dépôt de gaz cénomanien.
The northern dome is dissected by two strike-slip fault systems almost perpendicular to each other, one of them fading out almost completely at the top of the Jurassic (average depth of 2300 m), while the other system cross cuts the entire sedimentary cover up to the base of the Quaternary. The southern part of the linear high is covered by several en-echelon systems that have common SW-NE direction.
Note should be made that SSFS in this area have features in common with SSFS in many other areas. The length of the faults and angles at which the en-echelon faults deviate from the en-echelon system axis increase towards the surface. The en-echelons at either side of the main deformation trend have different dip directions. This fact is depicted by the en-echelon color pattern on dip maps: at one side of the axis the en-echelons are black, at the other side they are white. Note also a stepwise decrease in the number of en-echelon faults at the transition between the Jurassic and the Cretaceous layers. This may be attributed to the lithology of this interval: it is predominantly shaly, less rigid and more plastic as compared with the intervals above and below which are sandy-shaly. The ductile interval significantly changes the stress field, thus inducing a decoupling between the shallow horizons and the deepest part of the sedimentary layers and underlying basement.
The system of en-echelon faults, observed here, is highly similar to sand-box models proposed in many publications. Thus, Fig. 4 shows models borrowed from Sylvester's work (Sylvester, 1988) that resemble to a considerable degree the outer geometry of en-echelon faults observed in our subsurface data. At the same time, the models do not include some essential specificities of the real faults. First of all, note different attitudes of en-echelon faults at either side of the en-echelon system axis. Accordingly, vertical throw of the en-echelon faults is close to zero near the en-echelon system axis. Second, en-echelon faults at either side of the axis are made up of separate fragments isolated from one another, even though they may sometimes be connected in a single fault line. In addition, as already noted above, a graben-shaped low is formed along the en-echelon zone axis due to rock extension. The differences noticed between real strike-slip deformations and the published models are decisive when considering the geometry of hydrocarbon traps formed in the en-echelon fault zones.

En-echelon fault models above basement strike-slip faults as proposed by Sylvester (1988): 1. “Tulip” structure formed under additional transversal extension. 2. “Palm tree” structure formed under additional transversal compression.
Modèles de failles en échelon au-dessus de décrochements dans le soubassement, selon Sylvester (1988) : 1. Structure en « tulipe » formée sous l’extension transversale additionnelle. 2. Structure « en palmier » formée au-dessous de la compression transversale additionnelle.
Revisit Fig. 3 and note that en-echelon shears in the most powerful shear system disrupt the entire sedimentary section up to the base of the Quaternary. This observation demonstrates that shear systems were still active during Neogene-Quaternary tectonics that possibly re-activated the older fault system. These deformations are likely primary response of the crust to the latest stresses.
It is already noted above that the en-echelon fault systems fade out at different stratigraphic levels. We do not associate this fact with a chronology when the strike-slip faults were formed in the basement. A more logical explanation would be that en-echelon fault systems fade out in different levels because of different energy or strike-slip magnitude. The relation between the thickness of a deformed sedimentary section and basement strike-slip magnitude established in the modeling experiments (Naylor et al., 1986), where the relation between the strike-slip magnitude and the thickness of the deformed overlying sedimentary section was shown to be 0.3–0.8, may be the basis for assessing the range of strike-slip magnitudes as being a few hundred meters to a few kilometers.
The situation observed in the Yetypur linear high appears to be much similar to a number of other localities in the studied area. Fig. 5 shows dip maps at the B boundary, associated with the top of the Jurassic for several areas that demonstrate other en-echelon systems. More examples are considered in (Belyakov et al., 2002; Elmanovich et al., 1998; Gogonenkov et al., 2002, 2007; Timurziev and Gogonenkov, 2007; Gogonenkov and Timurziev, 2009, 2010; Koronovsky et al., 2009; Timurziev, 2009a, b, 2010).
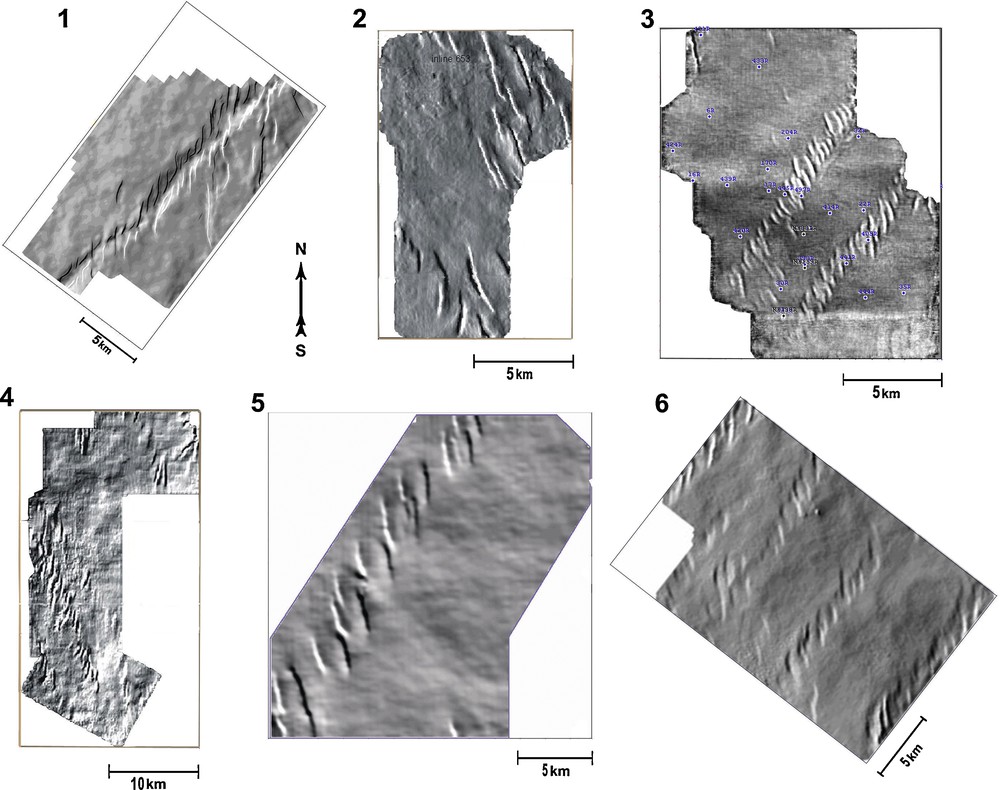
Examples of strike-slip dislocations in a number of exploration areas; strike-slip dislocations are identified along top of the Jurassic Horizon B on dip maps: 1. Metelnaya area. 2. North-Kharampur area. 3. Komsomol area, western dome. 4. Kharampur area. 5. Chasel area. 6. Yarayner area.
Exemples de dislocations de décrochement dans un certain nombre de zones explorées ; des dislocations de décrochement sont identifiées le long de l’horizon B (sommet du Jurassique) sur les cartes de pendage.
4 Parameter estimates for en-echelon fault and fold systems
On behalf of an oil company, more than 6000sq. km 3D seismic data and over 13,000 line km 2D seismic data were used to map all the faults in an area of 21,000 sq. km encompassing five large anticlinal structures, and the oil and gas deposits associated with them. Fig. 6A is a structural map at the top of the Jurassic (Horizon B), while Fig. 6B shows all the faults extracted from seismic data along the same horizon.
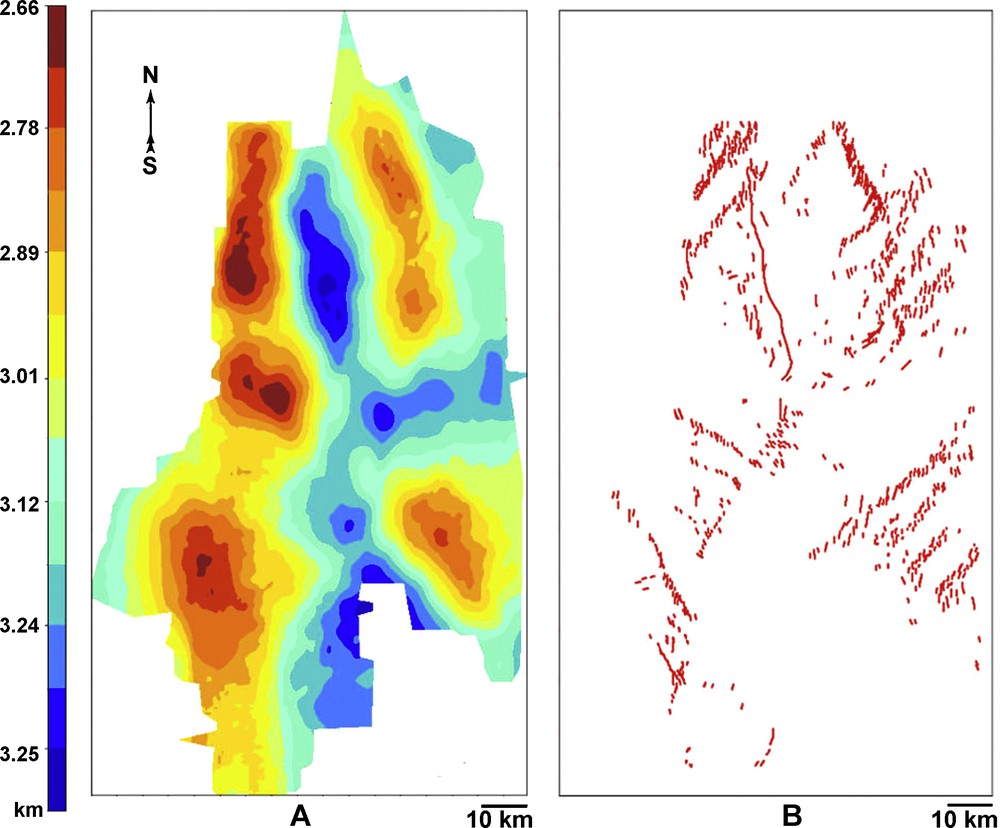
A. Structural map for top of the Jurassic in the area of a detailed analysis of disjunctive tectonics. B. Map showing faults at top of the Jurassic level.
A. Carte structurale du sommet du Jurassique dans la zone d’analyse détaillée de la tectonique de disjonction. B. Carte montrant les failles au niveau du sommet du Jurassique.
Only one “normal” fault was identified in the studied area; the fault is associated with the rupture and vertical displacement of sediments due to a fast growth of the northwestern high. All the other faults are elements of several en-echelon systems formed above the basement strike-slip faults. Most of the en-echelon faults were brought out by 3D seismic surveys. However, because 2D survey areas adjoin and overlap the 3D survey areas, it was possible to schematically follow a part of en-echelon systems on 2D survey areas as well, and get a more sound idea of the extent of en-echelon faults.
The en-echelon systems identified both beyond and within the study area, cross both the crests and flanks of anticlinal structures and interdome zones, with greater impact at the crestal culminations. It may be due to the fact that strike-slip deformations and en-echelon systems created by such deformations are instrumental in forming positive structures. Krapivner (1986) discusses this aspect in his work and notes that strong stresses occurring in the sedimentary overburden above the basement strike-slip faulting zones lead to the formation of a complex system of faulting and folding accompanied by dilatancy, i.e., growth of rock volume in the form of structural highs above the strike-slip zones.
The fault systems, imaged so far, allow assessment of quantitative parameters for en-echelon systems, basement strike-slips, and individual en-echelon faults. We will now consider statistics observed at the top of the Jurassic (Horizon B) in detail, and describe further how these statistical estimates are different at other horizons.
Table 1 presents inferred minimum, average and maximum parameter values. In the study area, 21 en-echelon systems were identified, whose average length is 15 km (maximum length is up to 29 km), while their average width is 2.033 km (maximum width is 4.8 km). En-echelon system azimuths in Fig. 7 correspond to two sectors: northeastern with average azimuth of 38°, and northwestern with average azimuth of 46°. Average angle between the two en-echelon systems is 84°, while the bisecting line is almost north-south, i.e. submeridian.
Estimations statistiques pour les systèmes en échelon et les failles en échelon de la zone d’étude.
Echelon Systems | ||||||||||||
No of Echelon Systems | En-echelon System Lengths | En-echelon System Widths | En-echelon System Azimuths | |||||||||
Value, m | Value, m | Value, deg. | ||||||||||
Min. | Av. | Max. | Min. | Av. | Max. | Min. | Av. | Max. | ||||
21 | 7,800 | 15,000 | 29,000 | 500 | 2033 | 4800 | 25 | 38 | 50 | |||
Echelon Faults | ||||||||||||
No of Echelon Faults | En-echelon Fault Lengths | En-echelon Fault Azimuths | Distance between En-echelon Faults | En-echelon Fault Rotation Angles from Strike-Slip Axis | ||||||||
Value, m | Value, deg. | Value, m | Value, deg. | |||||||||
Min. | Av. | Max. | Min. | Av. | Max. | Min. | Av. | Max. | Min. | Av. | Max. | |
401 | 102 | 1600 | 5155 | −60 | 0 | 60 | 250 | 929 | 2600 | 10 | 45 | 70 |
Fault Dip Angles to Vertical | Vertical Fault Throw Magnitudes | Stratigraphic Depths of Fault Penetration | ||||||||||
Value, deg. | Value, m | No of Faults | ||||||||||
Min. | Av. | Max. | Min. | Av. | Max. | |||||||
−30 | 0 | 30 | 2 | 14 | 82 | A–B (305) | A–M (34) | A–Q (25) |

Strike azimuths of basement strike-slip faults.
Azimuts d’orientation des décrochements dans le soubassement.
Individual en-echelon faults, identified in the study area, are 401 in number with an average fault length of 1.6 km (maximum length: 5.155 km), and the inter-en-echelon distance ranging from 0.25 km to 2.6 km, with an average of almost 1 km. One should keep in mind that the actual number of the en-echelon faults is larger, because only 3D seismic survey areas were examined, whereas 2D survey areas were used just for inferring the en-echelon fault zone size. It is essential that the en-echelon strike azimuths vary symmetrically relative to the north-south direction. It is precisely this north-south, i.e., submeridian, direction that is dominant among the multitude of the echelon faults identified in the area of study1. The en-echelon faults at the top of the Jurassic level rotate from the strike-slip fault axis at an average 45° angle, while dip angle is within ± 30° about the vertical axis. The vertical throw magnitudes of the en-echelon faults vary from 2 to 82 m and are 14 m on the average. The lower part of Table 1 shows the number of faults that penetrate the subsurface section from the basement (A) up to three shallower stratigraphic levels: (B) – top of the Jurassic, (M) – top of the Middle Cretaceous and (Q) – base of the Quaternary. Additional faults appear at the upper part of the section that do not reach the basement but fade out in the Cretaceous and the upper parts of the Jurassic.
At horizons distinct from Horizon B, the number of other seismically mapped en-echelon systems appreciably decreases upward in the section down to just 3 at the Neogene level. The length of the en-echelon systems stays the same, while their width rises to reach a maximum of 7.4 km. The northeastern and northwestern azimuth directions of the en-echelon systems also remain the same.
There are 71 en-echelon faults at the Neogene level and their average width increases to 2.3 km. The distances between the en-echelon faults vary from 0.5 to 2.5 km with a 1.2 km average. The direction of the en-echelon faults is also north-south, i.e., submeridian, at this level. On average, the en-echelons faults generally tend to rotate to a greater angle from the strike-slip fault axis, which is 50° at the Cenomanian surface. While average vertical throw is 14 m at Horizon B, it grows towards the Cenomanian where it is 26 m.
Analysis of strike-slip fault systems in the study area shows that the SSFS display the following patterns:
- • a characteristic en-echelon basement-involving strike-slip fault system is formed at the base of the sedimentary cover and penetrates to various stratigraphic level from the middle of the Jurassic section to the present-day surface;
- • the en-echelon faults are more numerous but have a smaller strike extent at the base of the sedimentary section. Upward in the sedimentary section, the overall extent of en-echelon fault rises, whereas the number of individual en-echelons faults decreases;
- • the strike-slip zone effects show up as a widening in a fan-like manner up the section from a narrow corridor at the base of the sedimentary cover up to its maximum lateral extent at some depth determined by the basement strike-slip magnitude;
- • the fault systems are distinctly positioned along two conjugate directions: SW to NE and SE to NW. No strictly east-west or north-south en-echelon system axes were identified;
- • in the study areas, the NW-striking faults are dextral, whereas NE-striking faults are sinistral.
5 Strike-slip fault structures (SSFS) development geography
For a well-grounded prediction of SSFS development in the rest of the West Siberian Basin, we used a structural map for the top of the Albian-Cenomanian (Kontorovich and Nesterov, 2000) to prepare Fig. 8. This map clearly shows a range of large NNW-trending linear highs (310°–320° azimuth). All of such linear highs that fall within the zone of our study, i.e., Vyngayakh, Vyngapur, Yetypur, Gubkin and others, experienced strike-slip dislocations. This provides grounds for suggesting that other linear highs along this axis farther north and farther south may have experienced strike-slip dislocations as well. A tentative analysis of data from areas located to the south, i.e., the Varyogan linear high, the Tagra area, and others, and to the north, i.e., the Medvezhiy linear high, of the studied area, and a number of areas on the Yamal Peninsula and in the Gulf of Ob fully confirms our assumption. On a structural map at the top of the Cenomanian, this structural line is expressed as a trend of en-echelon-type linear highs linking the deep-seated West Siberian tectonic suture related with the Khuduttey branch of the Koltogor-Urengoy graben-rift (Surkov and Zhero, 1981). It is important to note that west of this range of linear highs, neither 3D nor 2D seismic data give grounds to believe that SSFS spread westwards. Therefore, this linear highs may be regarded as the western boundary of the SSFS development zone. Another structural line, expressed as the range of en-echelon-type junctures of the Kharampur, Chasel, Russkiy, Tazov and other linear highs that extend towards the Bolshekhet depression and Yenissei-Khatanga downwarp, is presumably related to the Khudosey graben-rift fragments that were activated during the neotectonic phase. This structural line may be assumed to form the eastern boundary of the SSFS development zone.

Structural map for the West Siberian basin at the top of the Albian-Cenomanian (Kontorovich and Nesterov, 2000) shows positions of regional supra-order linear mega-structures, e.g., Khuduttey and Khudosey fault, with which linear highs and linear mega-highs of the 1st and 2nd order are associated and complicated by strike-slip fault structures (SSFS). A rectangle outlines the area discussed in this article.
Carte structurale pour le géosynclinal de la Sibérie occidentale le long du sommet de l’Albo- Cénomanien (Kontorovich et Nesterov, 2000) montrant les positions de méga-structures linéaires régionales d’ordre supérieur (failles de Khuduttey et Kudhosey) auxquelles des crêtes et des méga-crêtes linéaires de 1er ou 2e ordre sont associées et compliquées par SSFS. Un rectangle indique la zone étudiée dans cet article.
A southward spreading of SSFS along this structural line is suggested by a number of indirect evidences within the Vasyugan oil bearing region of the Khanty-Mansi Autonomous District on the structures of the Bakhilov, Alexandrovskiy and Mezhdurechenskiy linear highs including the Bakhilov, Khokhriak, Permiak and Kolik-Yogan highs.
Both axes form a fan that opens up to the north. The northern boundary of the strike-slip dislocations is still undefined. However, the available data from Barents and Kara seas (Krapivner, 1988, 2007; Verba, 2007) indicate an extension of the basement strike-slip faults and related en-echelon faults within the sedimentary cover into offshore areas. Based on acoustic measurement and shallow drilling data from much of the Barents Sea Russian sector, Krapivner (2007) showed that the youngest, i.e. Neogene-Quaternary to Recent deformations can be interpreted as en-echelon systems developing in the sedimentary cover over basement-involving strike-slip zones. Fig. 9 from Krapivner (2007) is an acoustic measurement profile exhibiting distinct low magnitude faults in the near-surface strata overlain by a thin layer of silty sediments. Fig. 10 from Verba (2007) is a seismic profile recorded in the northeastern Barents Sea (profile line location is shown in the inset) that displays distinct tectonic “flower” structures that identified two systems of en-echelon faults over underlying bedrocks.
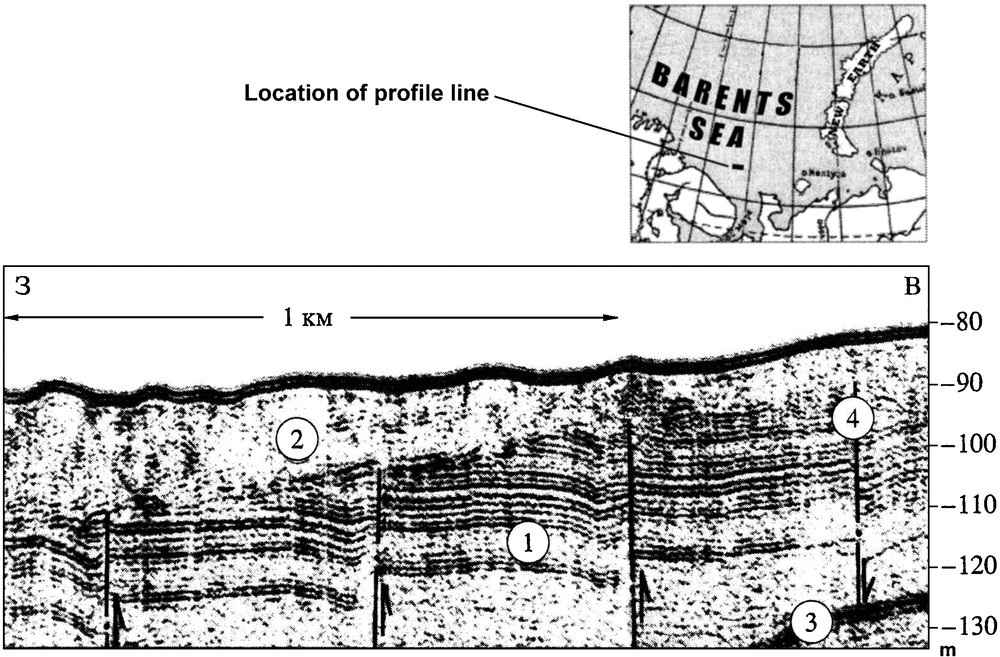
An example of low-separation rupture on the Barents Sea shelf derived from acoustic measurement data (Krapivner, 2007). 1. Upper Cretaceous rocks. 2. Consolidated recent sediments (diamicton). 3. A multiple reflection from the sea floor. 4. Faults. Inset shows profile line location.
Exemple de rupture lente sur le plateau continental de la mer de Barents, d’après les données de mesures acoustiques (Krapivner, 2007). 1. Formations du Crétacé supérieur. 2. Sédiments consolidés récents (diamicton). 3. Réflexion multiple à partir du plancher marin. 4. Faille. L’encart indique la localisation de la ligne de profils.
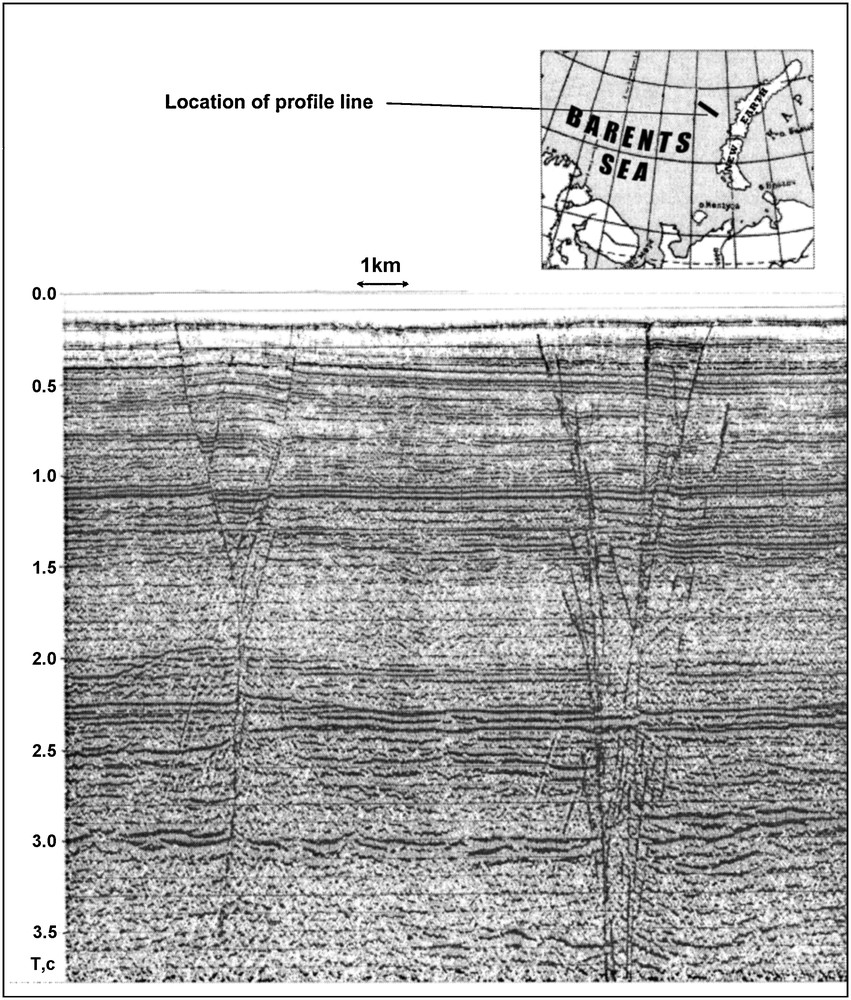
A portion of seismic line in the northeastern Barents Sea (inset shows profile line location) where “flower” tectonic structures are recorded allowing inference of two systems of en-echelon faults over strike-slip faults in the underlying bedrocks (Verba, 2007).
Portion de ligne sismique dans le Nord-Est de la mer de Barents (l’encart indique la localisation de la ligne de profils), où des structures tectoniques « en fleur » sont enregistrées, permettant de déduire la présence de deux systèmes de failles en échelon au-dessus des décrochements dans les soubassements rocheux (Verba, 2007).
Characterizing parameters of strike-slip systems, Krapivner (2007) notes that:
- • vertical displacement magnitude does not commonly exceed 10–30 m;
- • contiguous faults are a few kilometers to few hundred meters apart;
- • fault widths successively decrease down the section;
- • faults of insignificant length can merge into linear zones;
- • lengths of other individual faults vary 1.5–3 to 5–8 km.
Such characteristics perfectly agree with statistical data on West Siberian strike-slip systems presented above in Section 4.
6 Possible nature of West Siberian strike-slip dislocations
As already discussed above, strike-slip deformations form two distinct conjugate systems: southeast to northwest and southwest to northeast. This is well shown by quantitative assessment of strike-slip dislocation axes within a relatively restricted area (Fig. 6), and it is confirmed by a series of surveys in other areas. What could have been the cause of such powerful and highly unusual dislocations? To answer this question, we rely on the physical modeling data. On the left of Fig. 11 are shown en-echelon system axes (solid lines) identified on 3D data; the en-echelon systems are associated with basement-involving strike-slip faults. Dashed lines indicate axes predicted from 2D data. The right-hand part of Fig. 11 is an illustration borrowed from Hoeppener et al. (1969) characterizing shear fractures in a homogeneous viscous-elastic model subjected on one side to a horizontal compression along the meridional axis. It is seen that deformations create a conjugate system of shear fractures at an angle of about 80° oriented towards the maximum compressional stress axis. Ellipses illustrate the deformation of circles on the model surface.
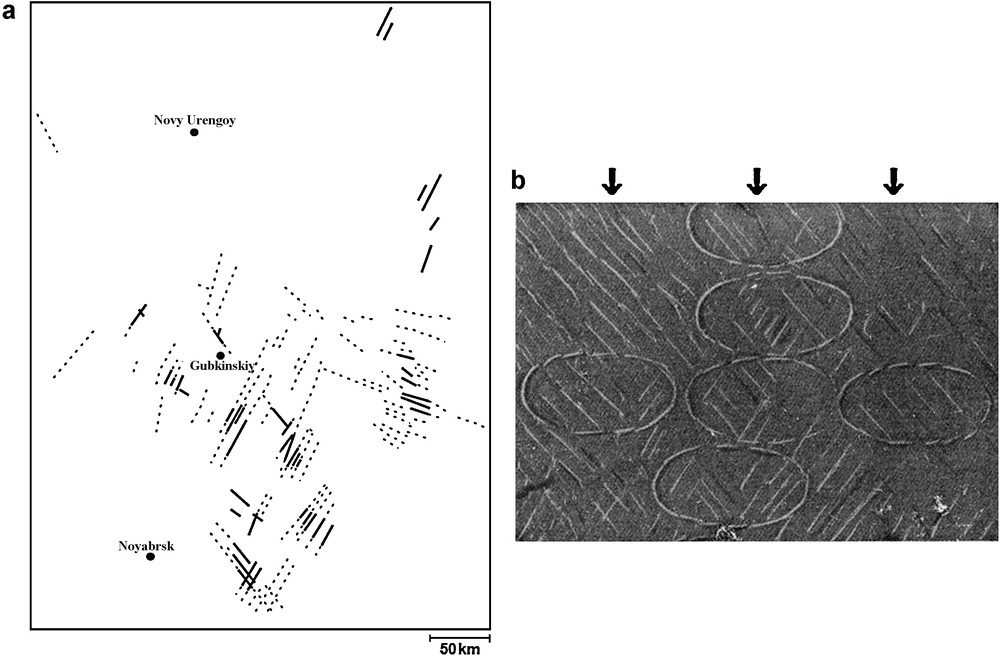
Spatial and azimuthal distribution of axes of en-echelon system associated with basement strike-slip faults, (a) is compared with Hoeppener et al. (1969) where one-sided horizontal compression acts in a viscous-elastic model (b). It can be seen that a system of shear fractures occurring in the model under compression is very similar to that observed in the real subsurface (a). Note also the similarity between shear fracture angles: the acute 80° angle of the model is directed along the compression axis; in the real subsurface, the acute angle is 84° and it is directed practically along the meridian.
La distribution spatiale et azimutale des axes du système en échelon, associés aux décrochements du soubassement (a), est comparée à Hoeppener et al. (1969), où la compression horizontale considérée agit dans un modèle visqueux-élastique (b). On peut noter qu’un système de cisaillements qui se produit dans le modèle sous compression est très semblable à celui qui est observé dans la sub-surface réelle (a). On peut noter aussi la similarité entre les angles de fracture de cisaillement : l’angle aigu de 80° est dirigé le long de l’axe de compression ; dans la sub-surface réelle, l’angle aigu est à 84° et est dirigé pratiquement le long du méridien.
The shear fractures in the model are very similar, almost analogous, to those observed in the real subsurface data. As in the modeling experiment, the angle between conjugate shear fractures is close to 80° with the bisectrix directed to the north along the meridian.
The above discussion allows one to explain why the conjugate shear fractures in the Paleozoic section of the study area are caused by a powerful and geologically short-term pulse of north-south compression operating in the central West Siberian Plate at the turn of the Tertiary and Quaternary. This has generated a system of shear fractures in the crust. Each of such shear faults or the most intense of them was responsible for forming complex systems of deformations in the overlying Meso-Cenozoic sedimentary sequence. The distinctive feature of the deformations is a series of en-echelon faults above the shear fractures in the basement. Consider now geodynamics that can possibly be responsible for such powerful directional compression pulse. Analysis of plate tectonics of the Eurasian northern margins (Sobornov, 2007; Sobornov and Yakubchuk, 2006) shows that there was a period of tectonic relaxation during the Eocene when the Urals were practically all eroded, while West Siberia and northern areas of the East European platform were already tectonically accreted. Tectonic reactivation of this vast region followed during Oligocene and it is still going on. Individual blocks along the periphery of this region have experienced abrupt deformations, while the eroded Ural mountain system rises again, and the Arctic Ocean opens up at a faster rate. This regional process apparently included one or more powerful north-south compression cycles of the Eurasian margins. As a consequence, a unique system of conjugate shear fractures emerged on the Arctic shelf and extended as well into the northern and central parts of the West Siberian Basin.
7 Strike-slip tectonics, and hydrocarbons exploration and production
The West Siberian lowland is one of the World's largest hydrocarbon-bearing basins where hundreds of oil and gas fields were discovered and put under production. A large number of the fields are located in a region disturbed by strike-slip deformations. These oil fields share a whole number of distinctive characteristics directly related with the dislocations described above.
There is first of all an unusually high number of productive beds. In zones with no SSFS only three to five beds are productive which are located in direct proximity to the top Jurassic Bazhenov Horizon which is regarded to be the main oil source formation, whereas, in the basin, tens of beds are productive as a rule in zones with SSFS. The oil bearing reservoirs are distributed over the entire Jurassic-Cretaceous section. Thus in the Yetypur field (Figs. 2 and 3), more than 40 hydrocarbon-saturated reservoir horizons are identified in the northern dome and 36 others in the southern one. The largest Cenomanian trap in the Yetypur field can be easily mapped by a flat reflection from the gas-water interface at 0.95 s, as indicated by sections in Fig. 3. Similar situations are observed practically in all of the fields where SSFS affect the entire Jurassic-Cretaceous section. This is not surprising. En-echelon faults in the SSFS zones should be more properly referred to as detachments, because it is precisely the tectonic decoupling of one fault side from the other that took place due to extension forces, rather than a vertical propagation of the fault rupture. These deformation features, while penetrating the whole sedimentary section, formed effective pathways for vertical migration of fluids between both beds within the Jurassic-Cretaceous sequence and dislocated Paleozoic rocks and crystalline basement where gaseous and fluid hydrocarbons may have matured and migrated later through the SSFS channels into the overlying sedimentary cover to fill up numerous traps. The gaseous hydrocarbons migrate faster and fill up uppermost traps in the Uppermost Cretaceous and Neogene reservoirs. The reverse exceptionally took place as well when gas got stored in the Jurassic or Lower Cretaceous, whereas a number of overlying traps were filled with liquid hydrocarbons.
Despite the relatively low magnitude of the vertical offset of en-echelon faults, such deformations as a rule broke up productive beds into numerous individual fault-sealed traps. As dislocation magnitudes and stereometry of the en-echelon faults are variable from bed to bed, the shapes and sizes of the traps are unique to each bed. Therefore building geologic models and estimating hydrocarbon reserves in such fields is much more expensive than handling simpler prospects. As a consequence prospecting and exploration of hydrocarbon fields becomes a challenge in the SSFS zones. 3D seismic surveys must be carried out as early as productivity of a structure has been established. Designing well patterns should anticipate probable multipay arrangement of the field and complex geometry of the faults. Optimal wellbore geometry of exploratory wells should be properly designed to successively penetrate the shallowest parts of potential traps at all stratigraphic levels. These should be deviated wells that would follow the trend of the main strike-slip faults.
An additional extraordinarily significant characteristic of productive beds located in the SSFS zones is an intense reservoir fracturing which enhances reservoir permeability very much, hence flow rates of production wells. Rock fracturing is a direct consequence and physical manifestation of rock dilatency generated by tectonic deformations. Young age of the deformations allows valid assumption that the related fracturing has not healed yet and is still active in providing rock transmissibility. Enhanced reservoir permeability in both SSFS zones and directly above them was noted by researchers both before and during this study time (Afonin, 2008; Bespalova and Bakuyev, 1995). The impact of fault zones established in shallower beds indicates that macrofault zones, imaged by 3D seismic surveys (a minimal fault separation magnitude confidently identified by 3D seismic is 2–4 m), extends far up for hundreds of meters in terms of microfracturing formation and thereby increases well production rates. Study in the Kharampur field (Afonin, 2008) shows that the wells located closer than 1.5 km from the en-echelon faults have on average twice as high a production rate than the wells located more than 2.5 km away. It is especially important to note that the fracturing is directional. The fractures are quasi-parallel to the meridional direction of en-echelons maximum compression. Horizontal permeability anisotropy should be taken into consideration when determining the geometry of deviated and horizontal wells, and also when designing well patterns for injection and production wells in order to ensure that oil displacement is at its maximum.
8 Conclusion
The large amount of experimental data accumulated to date allows us to assert that a new geologic phenomenon was identified in much of the West Siberian oil and gas bearing basin, i.e., the extensive development of a basement-involving SSFS. The SSFS has caused a complex folding and faulting pattern in the overlying sedimentary cover that accommodates numerous oil and gas reservoirs. Judging by the age of rocks subjected to strike-slip dislocations, SSFS were formed at the turn of Tertiary/Quaternary periods. Individual faults extend vertically up to the base of the Quaternary section. Despite the fact that some of SSFS fade out at deeper levels, e.g., at the top of the Jurassic and Lower Cretaceous–the SSFS age should be dated to the Neogene-Quaternary on the grounds that their smaller penetration interval is due to the low energy, i.e., low magnitude, of the original basement displacement. Numerous strike-slip dislocations in the basement are part of a single system of conjugate shear fractures that were caused by a pulse of horizontal pressure along the north-south (submeridian) direction. The nature of shear fractures in the basement and the angle between the shear fractures are practically similar to the results of modeling a pure strike-slip fault. Such a similarity allows us to suggest that the formation of strike-slip dislocations evolved according to the above-mentioned modeling experimentations. The data gathered to date allow identifying the strike-slip deformation zone boundaries. The boundaries of the deformed zone comprise the Khudottey and Khudosey rift zones. The deformation zone widens northwards from the southern margin of the West Siberian Basin and extends into the offshore areas of the Kara and Barents Seas.
The SSFS in the sedimentary cover were studied in detail. En-echelon fault systems that formed as a result of strike-slip faults creating spatial “flower” structures are the most distinctive feature and principal diagnostic indicator of the strike-slip faults. Quantitative measurements of SSFS parameters showed that strike-slip zones extend for tens of kilometers, whereas offsets in the basement can reach hundreds of meters in width. En-echelon fault systems, formed above the basement strike-slip faults, are a few hundred meters wide in the lower part of the sedimentary section and grow up to a few kilometers in the Upper Cretaceous. The echelon fault planes are curved spatially. They have more gentle dip angles ranging form 45° to 50° in the upper part of the section and steeper dip angles ranging from 70° to 90° near the basement. It is important that echelon fault azimuths are highly persistent throughout the area and vary little about the main meridional trend, fixing thereby the maximum compression axis. Rock fracturing is naturally related with this trend in the strike-slip deformation zones.
The geologic structure specificities, discussed above, apparently control oil and gas field characteristics to an appreciable degree. The presence of powerful vertical fluid migration channels leads to an abnormally high productive bed count: 40 and more productive strata spanning an interval ranging from the Lower Jurassic to the Neogene. The en-echelon faults that initially formed gaping fluid migration channels closed under the lateral compression during the concluding phase upon relaxation of the pressure pulse, and finally formed tectonic barriers. The barriers break up each productive bed into a series of individual traps that need to be studied and geometrized. Exploration and estimation of hydrocarbon reserves, feasibility study of development and production, and subsequent monitoring of such fields is much more challenging than handling tectonically “quiet” fields.
Quite many oil and gas fields, located in the SSFS zones, were prepared for and put under production based on 2D seismic data, without having an accurate idea of the real tectonic framework nor correct geologic models. Many today field development problems originate from incorrect geologic models. Such fields urgently need 3D surveys, corrected geologic models and rehabilitation plans.
The West Siberian Basin is not unique because similar strike-slip dislocations and associated en-echelon fault systems occur also in the offshore areas of Vietnam, in the Algerian Illizi Basin and in the Timan-Pechora Basin of the Russian Platform. But it is only in West Siberia that SSFS are documented at such regional scale, and that no other type of faults is present in the sedimentary cover. This allows such dislocation type to be seen in pure form “as in a textbook case”.
Although a good deal of investigations were carried out in the already well studied field, and theoretical analyses had been performed and models built, many issues related to the development of strike-slip dislocations, the impact of such dislocations on the formation of oil and gas fields, and on an effective development of productive zones in the SSFS environment remain unresolved and will require collaborative efforts of oil companies and tectonophysics researchers.
Acknowledgements
The authors are grateful to the respective management of RosNeft and GazpromNeft for permission to use their seismic data. We are also thankful to a large team of CGE geophysicists who processed and interpreted the seismic data used in this publication.
1 Among over 20 areas studied, only one (i. e., North-Komsomol area) exhibits echelon faults striking east-west indicating special stress conditions in this zone.