1 Introduction
Climate fluctuation has been one of the main forces driving the dynamics of biodiversity on Earth. The response of ecosystems to climate changes is thus a central issue in the evolution of life and can only be understood through detailed analyses of the relationships between organisms and environment and among organisms. Biotic interactions are the mechanisms that link together the individual pieces and they must be understood simultaneously at proximal (ecological mechanisms) and ultimate (evolutionary processes) levels.
Obligatory interactions, i.e., interactions in which at least one partner depends on the other, are among the most fundamental to the functioning of ecosystems. They are either mutualistic, i.e., interactions that are beneficial for the interacting species, or parasitic, i.e., interactions that are beneficial for one species but detrimental to the other. Reciprocal benefits improve the ecological success of the interacting species and, at the ecosystem level, play key roles in nutrient cycles and energy flow. Mutualistic and parasitic interactions both generate biodiversity, by accelerating speciation (Hoffmeister and Martin, 2003; Margulis and Fester, 1991; Mereschkowsky, 1926), and maintain biodiversity, as specialization facilitates coexistence (Poisot et al., 2011). In the context of global change, mutually beneficial interactions are important for the functioning and stability of ecosystems (Danovaro et al., 2008). Moreover, mutualisms are key factors in the adaptation and survival of species, but obligatory mutualisms are more sensitive to environmental changes than facultative mutualisms (Kiers et al., 2010).
Obligatory interactions are expected to be particularly sensitive to climate change because the two partners may respond differently to changes but each still depends upon the other for its survival. Their sensitivity and their structuring role in ecosystems make them good models for studying the consequences of current and past climate changes at the scale of the ecosystem. Understanding the mechanisms underlying responses of obligatory interactions to abrupt past climate changes should help us understand changes in ecosystems that are happening now and predict what may happen in the future.
The effect of current and past climate changes has been thoroughly investigated for species considered individually. Species and population histories are usually inferred either from the palaeontological record or from genetic data on current populations using phylogeography and population genetics (Hewitt, 2004; Vegas-Vilarrubia et al., 2011). Interest in the response of species communities to changes has grown only recently (Urban et al., 2012). In addition, reconstruction of past vegetation composition through pollen records, correlated with reconstruction of past climate fluctuation using physical and chemical proxies, allows to assess the intensity of past changes and the related response of ecosystems. However, the mechanisms underlying these responses are rarely investigated.
Most of the periodic climate fluctuations that occurred in the past are due to periodic variation in parameters of the Earth's orbit, called Milankovitch oscillations (Berger, 1988). Time scales of these climate fluctuations, ranging from tens of thousands of years to over a hundred thousand years, are likely to have driven evolutionary changes in species and populations. Milankovitch oscillations combined with tectonic organization are thus considered a major driver of evolution, shaping life history traits, interspecies interactions, and global patterns of biodiversity at different scales (Dynesius and Jansson, 2000; Webb and Bartlein, 1992).
In this paper, we review the potential effects of climate change on obligatory interactions, and search the literature for evidence of such effects in the recent past, mostly the Quaternary period. The paper is structured around three categories of effects of climate change on populations that are expected to have shaped the evolutionary history of obligatory interactions: fragmentation of populations, range shifts, and partner extinction due to extreme and abrupt climate events. For each category we first present the predicted response of populations in obligatory interactions, and then illustrate these responses with examples from the literature.
2 Fragmentation of populations
Climate fluctuation induces changes in temperature and rainfall that are spatially heterogeneous, because physical characteristics of the landscape strongly influence local environmental conditions. Thus, even if climate becomes globally unfavourable to a given species, this species can still be maintained in isolated places with particular microclimates. Areas with sharp altitudinal variation are classical examples, where species can cope with climate change by shifting their altitudinal range distribution, which requires migrating over much shorter distances than in flat landscapes (Loarie et al., 2009). Other examples are the possible maintenance of biota requiring humid conditions along riversides in otherwise dry landscapes (Born et al., 2011; Colyn et al., 1991), or the maintenance of warm-adapted biota on sun-exposed slopes in otherwise cold landscapes (Graham et al., 2005, 2006; Schonswetter et al., 2005). Landscape heterogeneity may thus allow the persistence of species or communities throughout cycles of climate change.
During glacial periods, forests were considerably more restricted at all latitudes than in the current interglacial period and some forest-restricted species managed to persist only in localized regions—referred to as forest refugia or glacial refugia—presenting relatively favourable (warm, wet) climatic conditions (Hewitt, 2000; Petit et al., 1999). As climates warmed after glacial periods, surviving populations expanded to reach their contemporary ranges. That places existed where populations of one or more species persisted during climatic fluctuations is widely accepted. The role of refugia, i.e. places where a community or a whole ecosystem persisted, is globally validated in ecosystems of temperate and boreal regions (Schmitt, 2007). For tropical regions, no consensus about the role of forest refugia in the dynamics of biodiversity during climate fluctuations has been reached. Current debate concerns two points. First, the role of refugia in the evolutionary diversification of tropical biota remains unclear (see for instance Knapp and Mallet, 2003), with some hypotheses emphasizing lower extinction rates and allopatric divergence in isolated stable refugia (Hewitt, 2000; Knapp and Mallet, 2003) and others emphasizing rapid evolution in response to environmental change in less stable areas (Bush, 1994; Smith et al., 2005). Second, in Amazonia, for which forest refugia hypotheses were first proposed, the existence of refugia for whole ecosystems has been questioned. Palynological evidence concerning forest fragmentation during the Last Glacial Maximum and more recently during the Little Ice Age of the Holocene remains open to conflicting interpretations (Colinvaux et al., 2000; van der Hammen and Absy, 1994). For central Africa, questions now focus on the ‘universality’ of potential refugia. Did species survive in a limited common number of areas, or did they survive in one or more of numerous patches, depending on their specific ecology? Although there is much controversy about the existence of “universal” refugia, it is clear that many species have maintained viable populations in multiple isolated spots throughout climate fluctuations, in temperate as well as tropical biota (Hardy et al., this issue; Qiu et al., 2011; Shafer et al., 2010).
Cycles of climate change have resulted in cycles of expansions and contractions of species’ distributional ranges. The consequences of isolation of populations depend on life history characteristics, size of the isolated population, and duration of isolation. Species range variation and population isolation have shaped the demographic history of species, and are likely to have promoted population genetic structuring, species diversification through vicariance, or species extinctions. Theoretical optimal refugia may have been different for partner species and two predictions can be made. First, the stronger the dependence between partners, the stronger the impact of climate change is likely to be. In this context, species involved in obligatory interactions are probably more prone to extinction than species involved in facultative interactions. Secondly, as obligatorily interacting species may have quite different life history characteristics, population isolation is likely to affect each partner in different ways (Álvarez et al., 2010). In particular, interactions between large plants or animals and microorganisms, even if obligatory, could show incongruent traces of past events. Microorganisms have usually much greater dispersion ability than plants or animals. In symbiotic interaction the partner with the longer lifespan is usually referred to as the “host” and the partner with the shorter generation time and/or greater dispersal ability as the “symbiont”. In symbioses between plants or animals and microorganisms, a prediction from differences in life-history traits is that the host should display phylogeographic patterns that are consistent with those previously documented in other similar organisms of similar size, and that the symbiont is more likely to show either no phylogeographic pattern at all or patterns that are clearly different from that of the hosts, despite a common history.
Extensive gene flow after recolonization may erase genetic traces of population history. In the case of several isolated refugia, genetic drift may affect more strongly the partner with the lowest effective population size and results in a stronger phylogeographic pattern for this partner. Conversely, population isolation may lead to strong differentiation in species that have short generation times and high mutation rates, and lead to the formation of local variants in only one of the partners. Such variants may prove to be reproductively isolated after secondary contact, so that population fragmentation would lead to speciation in one partner but not in the other, or in both partners but at different paces. We could not find in the literature such a pattern of speciation that could be linked to past climate change, although we are confident that it should occur in some model systems. Model systems with a high specific diversity pre-dating the Pleistocene, with a wide distribution range and involving organisms with very different life-history traits should be targeted in priority, because they provide a large number of repeated situations where speciation of one partner could have occurred after fragmentation periods. Such model systems include the fig/fig wasp and the Yucca/Yucca moth nursery pollination mutualisms, where pollination of the plant strictly depends on specific insects that can only develop at the expense of a subset of the seeds; the ant-plant symbioses, where a plant hosts and feeds ants in return for protection; and the nutritional symbioses between aphids and bacteria that synthesize amino acids for them. The following three examples illustrate the predictions made above on the consequences of population fragmentation on population genetics of interacting species.
The European plant Arum maculatum needs specific psychodid flies for pollination (Espindola et al., 2011). However, the interaction is antagonistic, because the flies are lured and trapped by the plant and do not get any benefits. The interaction is thus obligatory for the plant, but not for the insect. This plant shows a phylogeographic pattern over Europe that is similar to that shown by many other independent organisms (Espindola and Álvarez, 2011), and consistent with recolonization of Europe from two or three glacial refugia (Hewitt, 2000). The two main pollinators, however, did not show any phylogeographic pattern (Espindola and Álvarez, 2011). In this case, it was not surprising that the psychodid flies did not display a phylogeographic pattern congruent with that of the plant because they may have maintained independently of the plant. Moreover, psychodid flies are capable of long-distance migration (Hardy and Cheng, 1986) and extensive gene flow, which may have erased any genetic signature of population history.
Another, more surprising, example of differential impact of climate change on partners in obligatory interactions comes from population genetic structure of a plant and its symbiotic ant in western Central Africa. Barteria fistulosa is called a myrmecophyte, or ant-plant, because it has hollow cavities (swollen twigs) that are used by the ant Tetraponera aethiops as a nesting site. The ants protect the plant against herbivores and competing vegetation (Dejean et al., 2008; Janzen, 1972; McKey, 1974). The association is thus qualified as a protection mutualism, and in this case, is obligatory and symbiotic: the ants have never been found anywhere else than in this plant species, and healthy plants are always occupied by these ants. As seeds and founding queens disperse independently, the association has to form anew at each generation, and the transmission is said to be horizontal. This symbiosis occurs in the rain forests of Central Africa, and the population genetics of both partners have been studied in the Lower Guinea domain, mainly in Cameroon and Gabon (Piatscheck, Peccoud, Born, McKey, Blatrix, unpublished). The plant shows a strong genetic differentiation between northern and southern Lower Guinea, a pattern that has been documented in several other tree species (Hardy et al., this volume). This pattern is most likely attributed to cycles of forest contraction and expansion in the Pleistocene and/or Pliocene, followed by recolonization and secondary contact of populations from distinct refugia (Debout et al., 2011). Interestingly, the ant partner of B. fistulosa does not show such a strong north/south genetic differentiation, indicating that population fragmentation following climate fluctuations does not necessarily affect partners of obligate symbioses in the same way.
A clear example of contrasted responses of symbiotic partners to past climate change comes from the comparison of the population structures of fungus-growing ants and their fungal and bacterial symbionts. Fungus-growing ants form a monophyletic group of more than 200 species in the American continent that grow a particular fungus for food. To protect the fungal garden from parasites, ants grow a diverse array of bacteria that produce various antibiotics (Sen et al., 2009). Colonies are founded by queens that take a piece of the fungal garden from their mother colony before dispersal, so that fungal cultivar transmission is pseudo-vertical (Chapela et al., 1994; Mueller, 2002). Workers of the fungus-growing ant Trachymyrmex septentrionalis host Pseudonocardia bacteria on their cuticle (Currie et al., 1999b, 2006). These bacteria protect the fungal garden against the specialized pathogen Escovopsis (also a fungus) by producing somewhat specific antibiotics (Currie et al., 1999a, 1999b). Although most of the fungus-growing ants are tropical species, T. septentrionalis is found throughout the eastern US. It has thus experienced strong population fragmentation following cycles of Quaternary glaciations. Population-genetic structure of the ant, based on nuclear and mitochondrial sequences, shows two mostly allopatric, eastern and western, genetic groups that likely diverged in the Pleistocene (Mikheyev et al., 2008). A similar genetic discontinuity in this part of the US is also observed in many other organisms and is attributed to diversification in allopatric refuges during glacial cycles of the Quaternary (Soltis et al., 2006). Surprisingly, although microbial symbionts are pseudo-vertically transmitted, population genetic structures of the fungal cultivar and of Pseudonocardia bacterial strains both show a pattern clearly different from the ant pattern (Mikheyev et al., 2008). Past climate changes had a very different impact on macroorganisms and microbes, although they live in obligatory symbiosis and symbiont transmission is pseudo-vertical. A possible explanation for this pattern is that symbiotic fungi and bacteria can also disperse independently from the founding queens of their ant host, as shown in other fungus-growing ant species (Green et al., 2002; Mikheyev et al., 2006, 2007), and thus, have different population dynamics.
The three cases above involve three different types of interactions. One is not obligatory for one of the partners. The two others are reciprocally obligatory but differ in their mode of transmission (horizontal or vertical). In all three cases, the genetic consequences of climate fluctuations were different between partners, even in the tightest interaction with vertical transmission. As these interactions are obligatory, species must have shared the same refugia. The differential impact of population fragmentation on partners is thus mostly due to differences in life-history traits. Even the type of interaction (reciprocally obligatory or not, transmitted horizontally or vertically) seems to be less important than differences in life-history traits.
3 Range shift (relatively slow changes)
In the course of climate change, populations of interacting species may experience range shifts, either latitudinal or altitudinal. If climate changes are slow enough, populations should be able to follow spatial distributional changes of their optimal environmental conditions. Whether this actually happens depends largely on dispersal dynamics of the species. In many cases of obligatory interactions, partners appear to have quite different dispersal capacities. Thus, although partners depend on each other for successful range shifts, the dynamics of dispersal are expected to differ between partners. In this section we investigate how these differences can affect the evolution of interactions.
3.1 Synchronicity in range shift of partners
In asymmetric interactions, such as host-parasite interactions, dependence is not reciprocal, so that host range fluctuation can occur without pressure from the parasite. In contrast, range dynamics of the parasite depend on both the range dynamics of its host and its own intrinsic life-history traits, and are thus more constrained than the dynamics of the host during cycles of climate changes. Range shifts of a host can impair parasite local adaptation because of the loss of synchrony in host and parasite population dynamics. In extreme cases, this could eventually lead to the extinction of the parasite, but such events are hardly detectable. Conversely, most parasites have better dispersal capacities than their host, so that they are expected to easily track their host. Judging from the great diversity of life-history traits exhibited by parasites, population dynamics of parasites in response to host range shifts should vary greatly from one system to another, in a way that is hardly predictable. However, in reciprocally obligatory interactions, such as specific mutualisms, partners should shift their respective ranges in synchrony. In this case, range shifts are limited by the most constrained partner. We thus expect a strong synchrony in range shifts between partners of obligatory interactions, even in interactions involving species with different life-history traits, and unpredictable outcomes in asymmetric interactions. The three following examples will illustrate these predictions.
The North American beech, Fagus grandifolia, underwent a rapid northward range re-expansion at the end of the Last Glacial Maximum (Bennett, 1985). The migration rate implied by this rapid colonization is not compatible with the life-history characteristics of the tree, in particular its limited capacity for seed dispersal and long time to reproduction. This discrepancy indicates that low-density populations probably persisted close to the ice margin (Tsai and Manos, 2010). The occurrence of cryptic northern—and even Arctic—refugia for many taxa is now widely recognized both in North America and in Europe (Parducci et al., 2012; Provan and Bennett, 2008). Epifagus virginiana is a root-parasitic angiosperm specific to F. grandifolia. As it can only grow on this tree species, we may expect that it tightly tracked its host during post-glacial colonization. However, the genetic pattern of colonization differed from the pattern of F. grandifolia, showing that the parasite lagged behind its host (Tsai and Manos, 2010). In fact, E. virginiana does not seem to be able to establish stable populations at low host densities. This suggests two conclusions. First, this parasite was probably absent from Fagus populations near the ice margin. Second, it could have moved northward only when its host had reached high densities in newly colonized areas.
Parallel phylogeographic patterns of colonization following the Last Glacial Maximum have been documented in a gobiid fish species (Ctenogobiops feroculus) and its mutualistic alpheid shrimp (Alpheus djeddensis), involved in a frequent and widespread obligatory mutualism in shallow coral reef lagoons of the Indo-Pacific region. In this interaction, the shrimp digs a burrow that is shared with the goby, which, in return, warns the shrimp of predators (Karplus, 1987). The interaction is obligatory as the partners cannot survive individually, and accordingly, are never found living independently in the field (Thompson, 2003). During the Last Glacial Maximum, sea level in the region was more than 100 m lower than currently (Bard et al., 1996), meaning that many of the shallow lagoons presently found in the eastern part of the mutualism's range did not exist or were dramatically reduced. Following deglaciation, sea level rose and these reefs could have formed by species migrating from deeper lagoons in the western part of the region (Paulay, 1990). Interestingly, both the fish and the shrimp showed a similar phylogeographic pattern of recent eastward expansion (Thompson et al., 2005). This pattern was not fully congruent with those exhibited by six other lagoon fishes (Fauvelot et al., 2003; Thacker, 2004), suggesting that similarity of the patterns shown by Ctenogobiops and Alpheus was likely caused by the obligatory and mutualistic nature of their interaction.
In North America, the Joshua tree (Yucca brevifolia) and its pollinating moths Tegeticula synthetica and T. antithetica provide another example of concerted range dynamics during Pleistocene climatic fluctuations. The plant can only be pollinated by these two moths (Pellmyr and Segraves, 2003). The moths actively pollinate flowers, in which they also lay their eggs; the larvae feed on a proportion of the developing seeds. The two moth species can only develop on Y. brevifolia, so that the interaction is mutualistic and obligatory. In addition, two moths of the genus Prodoxus, the sister genus to Tegeticula, depend on Y. brevifolia to complete their life-cycle but do not pollinate the plant, and are thus considered parasites of the system (Pellmyr et al., 2006). Palaeodistribution models and palaeorecords (fossil packrat middens) indicate that Y. brevifolia did not experience range reduction during glacial cycles, but shifted its range northward or southward periodically (Smith et al., 2011). The five interacting species all showed genetic signatures of concerted population expansion during the Late Pleistocene (Smith et al., 2011), even though the plant's range appeared not to have become substantially larger. Though population expansion is difficult to link precisely to cycles of glaciations, it remains that the interdependent species responded concomitantly to global changes. In this case, even the parasitic species followed the same dynamics, showing that they closely tracked population changes of their host.
3.2 Selection for particular phenotypes at colonization fronts
Although both partners of obligatory interactions should track climate change simultaneously, the genetic and phenotypic consequences of range shift are likely to differ according to life-history characteristics, and thus to be different between partners. The selection of particular phenotypes at colonization fronts, especially phenotypes that facilitate dispersal, is well known for species considered individually (Excoffier et al., 2009; Thomas et al., 2001; Travis and Dytham, 2002). This process, known as spatial sorting (Shine et al., 2011), is expected to affect organisms in different ways depending on their life-history traits. Mutualistic interactions between species with the most divergent life-history traits are thus the most likely to show signs of weakening at colonization fronts. A host individual at a colonization front can be viewed as a new habitat to be colonized by the symbionts. Symbionts with a phenotype allowing more rapid colonization of the host are thus expected to be prevalent at the colonization front, at least temporarily, either through selection or spatial sorting. If the phenotype of the best colonizer is also less mutualistic (i.e., provides less benefit to the host), such a process could potentially lead to destabilisation of the mutualism, at least locally, or could be transient, according to the intrinsic characteristics of the species, such as dispersal capacity, generation time, and competition between phenotypes. Unfortunately, we are aware of only one study model in which the consequences of colonization-front dynamics on obligatorily interacting species have been investigated.
The myrmecophytic plant Leonardoxa africana subspecies africana is endemic to a short and narrow strip of rain forest along the coast of Cameroon (McKey, 2000). It has hollow twigs which host the mutualistic ant Petalomyrmex phylax (Fig. 1). This ant protects the plant against phytophagous insects (Gaume et al., 1997). However, a small proportion of individual plants is occupied by another ant species, Cataulacus mckeyi (Fig. 2), which only nests in this plant but does not protect it (Gaume and McKey, 1999). This ant is thus considered a parasite of the mutualism. Population genetics data indicated that the three interacting species have undergone a recent southward expansion (Dalecky et al., 2007; Léotard et al., 2009). Although it is difficult to date the timing of this event, it is compatible with Holocene climate fluctuations. As the plant grows only in the shade of the understorey of coastal rain forest, it is highly likely that range expansion of the system is coincident with the last period of substantial forest expansion in this region. Both ant species show phenotypic traits associated with higher dispersal ability at the colonization front than in other parts of their range: higher ratio of sexual females relative to workers, larger wing size and body mass of foundresses (Léotard et al., 2009). This distributional pattern of phenotypes is likely a consequence of spatial sorting (Shine et al., 2011). As a consequence, colonies of the mutualistic ant species invest less in colony growth at the colonization front, and thus, less in plant defence (only workers participate in plant defence). Moreover, behavioural investment by individual workers in plant defence was lower at the colonization front (Vittecoq et al., 2012). Thus, both the reduced investment in growth relative to reproduction and the lower behavioural investment in defence per individual worker result in a strategy that is less mutualistic as a whole at the colonization front. In contrast, the plant did not show any sign of a more dispersive phenotype at the colonization front, nor any sign of a less mutualistic phenotype (Léotard et al., 2009). This plant has a very slow growth rate and a very long lifespan, suggesting a generation time longer by orders of magnitude than that of its mutualistic ant. As the less mutualistic ant strategy at the colonization front is expected to be transient (it should be replaced by the usual ant phenotype as the colonization front moves forward), there is probably not enough time for the plant to evolve a less mutualistic phenotype, and thus the mutualistic association is maintained. In this case, past climate change has not induced a breakdown of the mutualism, but the example illustrates how such a breakdown could occur, in other obligatory interactions with different life-history traits.
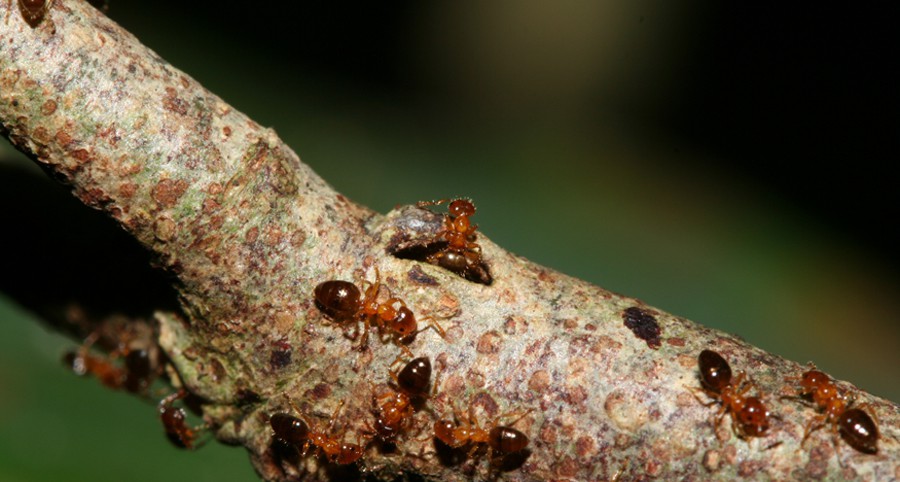
The African plant Leonardoxa africana subsp. africana has hollow twigs that are used as nesting sites by its ant symbiont, Petalomyrmex phylax. The ant protects the plant against herbivorous insects and provides it with nutrients. The two species have a mutualistic interaction and are strictly dependent on each other.
La plante africaine Leonardoxa africana subsp. africana a des tiges creuses utilisées comme nid par sa fourmi symbiote Petalomyrmex phylax. La fourmi protège la plante des insectes phytophages et lui fournit des nutriments. Les deux espèces ont une relation mutualiste et sont strictement dépendantes l’une de l’autre.
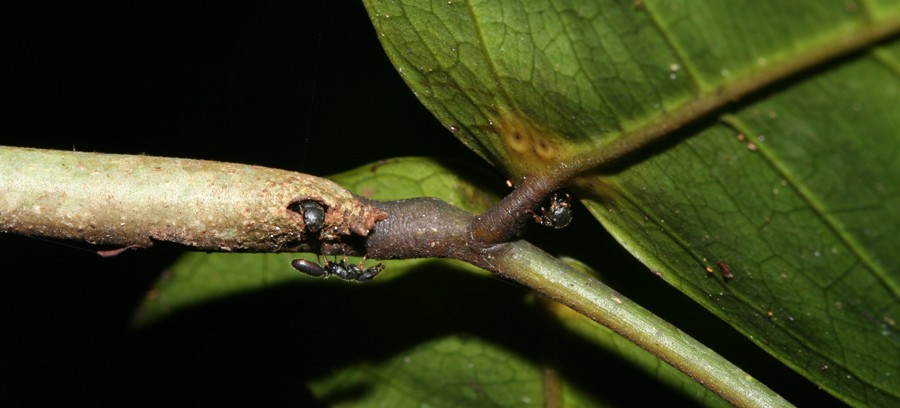
The ant Cataulacus mckeyi is considered a parasite of the symbiosis between the plant Leonardoxa africana subsp. africana and the ant Petalomyrmex phylax, because it occupies the plant but does not protect it against herbivorous insects.
La fourmi Cataulacus mckeyi est considérée comme un parasite de la symbiose entre la plante Leonardoxa africana subsp. africana et la fourmi Petalomyrmex phylax, car elle occupe la plante, mais ne la protège pas des insectes phytophages.
4 Potential partner extinction due to abrupt and short-term events of climate change
Apart from progressive climate changes due to long-term fluctuations (such as Milankovitch oscillations), organisms are recurrently exposed to extreme climate events of short duration. Such events can affect the whole surface of the globe and last only for a few months. They are thus difficult to detect in the palaeontological record, but may have dramatic consequences on living organisms. The fundamental difference between progressive and abrupt climate changes is that species do not have time to adapt or shift range when changes are abrupt. Note that current climate change is sufficiently rapid that it may be experienced as abrupt by tropical organisms (Williams and Jackson, 2007). If the change exceeds the range of tolerance of a species, it will disappear. The potential outcomes of abrupt climate changes can be predicted as follows. If the change is within the range of tolerance of both species, the interaction can persist but may be severely affected. Alternatively, the change can be out of the range of one of the interacting species. In this case, the outcome for both species will depend on whether or not the less affected species will be able to survive on its own until the more affected partner recovers. The outcome will thus depend on a combination of the following parameters: life-history traits of the two partners, duration of the climatic event, degree and nature of dependency between the partners, dispersal ability of the affected partner, and geographical extent of the event (which determines whether or not the affected partner will be able to recolonize empty host niches from source populations). The following examples illustrate two of these possible outcomes.
It is essentially in very recent time that the effect of such events on interacting species can be assessed, because their traces are likely to be lost quickly in the palaeontological record. El Niño–Southern Oscillation (ENSO) is a climatic event in the Pacific Ocean that occurs every three to seven years and lasts for several months. It corresponds to an abrupt increase of sea surface temperatures in the eastern Pacific. Climatic consequences are perceived over the whole Pacific region. Although most species are adapted to those fluctuations, particularly extreme events can have dramatic consequences on some ecosystems. Records of the ENSO are thus good opportunities to assess how obligatorily interacting species respond to abrupt climate changes.
Fig trees (more than 700 species in the genus Ficus) depend for their pollination on specific wasps (Agaonidae) that develop in a subset of the seeds. This nursery pollination mutualism is very similar to the one described above between Yucca trees and their Tegeticula moths. The two partners strictly depend on each other. The ENSO event of 1997–1998 caused an extreme drought in Borneo, which caused the loss of leaves and fruits in several species of fig trees. The duration of the drought exceeded the lifespan of the pollinators, so that although the trees survived the drought, pollinators became locally extinct (Harrison, 2000). Six months after the drought, pollinators had not yet recolonized and fertilised figs were absent. Similarly, Hurricane Andrew in 1992 caused the loss of leaves and fruits of a Ficus species in Florida, and caused presumably local extinction of the pollinator (Bronstein and Hossaert-McKey, 1995). However, in both cases, the long lifespan of the trees allowed recovery of the mutualism. Pollinating wasps, with short generation times and high dispersal capacities, spread from pockets where they had survived. Other organisms dependent on the fig/wasp mutualism may not have been so fortunate. Fig trees are considered keystone species in tropical ecosystems because many species directly rely on figs for food (Shanahan et al., 2001). The breakdown of the fig/fig wasp mutualism, even temporarily, may thus have dramatic consequences on local communities because production of figs depends on their pollination by fig wasps.
Responses of corals to the ENSO provide another case for studying the effect of abrupt climate change on obligatory interactions. Corals are sessile animals, many of them forming a calcareous skeleton that constitutes the structural basis for reef ecosystems. Like many other organisms in these ecosystems, they host intracellular microorganisms, dinoflagellates of the genus Symbiodinium, which provide their host with products of photosynthesis and facilitate calcification of the skeleton (Muscatine and Porter, 1977; Stat et al., 2006). Corals are strictly dependent on their Symbiodinium symbionts. An increase in sea temperature induces the production of oxygen radicals by the symbionts, damaging cells of the host and eventually leading to the expulsion of the symbionts (Lesser, 2006). This process is known as bleaching because of the associated change in coral colour. Bleaching thus corresponds to the breakdown of the mutualism and can lead eventually to the death of corals and to dramatic changes in the structure and functioning of reef ecosystems (Baker et al., 2008). Bleaching has become more and more frequent since the 1980s and the most severe events are associated with the ENSO (Stat et al., 2006). Reconstitution of the Holocene history of coral reef accretion on the Pacific coast of Panama revealed that coral reefs collapsed 4000 years ago, for a 2500-year-long period (Toth et al., 2012). Death of corals was mainly due to increased variability of the ENSO. Such levels of variability are expected for the next century. Responses of coral reefs to Holocene climate fluctuation are thus relevant to understand how marine ecosystems will respond to current climate change.
5 Prospects and challenges for the future
There is no doubt that past climate changes have shaped the evolutionary history of interacting species. Analysing how obligatory interactions responded to these changes could contribute to a framework for understanding and predicting ecosystem response to current climate change. However, the current state of knowledge is not yet ripe for drawing scenarios. In the course of the present review, we identified two main gaps that need to be tackled in the future.
5.1 Dating demographic and evolutionary events
Precise dating of biological events is mandatory for reliable correlation with climatic events. Climate fluctuations can be traced in the palaeontological record with relatively good precision, but this is rarely the case for demographic and evolutionary events that affected organisms. Past remains of organisms can be dated with relatively good precision using geological features. However, the quality of traces left by organisms in the palaeontological record varies greatly depending on the type of organism, so that for most groups of organisms it is impossible to reconstruct their recent evolutionary history from fossils. Dating biological events from molecular data is a promising area that is already widely used. However, in most cases it lacks the required precision. Dating events from DNA sequences relies on the hypothesis of a molecular clock, i.e. a constant evolutionary rate within and among focal lineages and over time (Zuckerkandl and Pauling, 1965). However, it is now widely acknowledged that mutation rates and speed of evolution are highly variable (Ayala, 1997; Britten, 1986). As a consequence, to be reliable, the molecular clock needs to be calibrated within each lineage and for each type of DNA sequence (Yang and Rannala, 2006). Nevertheless, the use of relaxed molecular clocks that account for rate variation across lineages has improved the confidence in dating (Drummond et al., 2006). Calibration can be achieved using absolute dates of fossils. Unfortunately, for most taxonomic groups we lack fossils of appropriate age. Increasing knowledge of the processes shaping the evolution of populations and species allows building increasingly accurate models of population dynamics and evolution. In addition, statistical methods relying on Bayesian inference allow dating demographic events (Heled and Drummond, 2008). Molecular dating thus holds promise for a better understanding of the biological effects of past climate changes. An interesting feature of species in obligatory interaction is that they share the same biogeographic history. Interdependency between partner species may prove to be a useful constraint for calibrating models of evolution in a comparative framework. Obligatorily interacting species are thus particularly relevant for investigating the effect of climate change on ecosystems.
5.2 Need for more studies on obligatory interactions, and for a focus on particular biological models
Our review of the literature revealed that relatively few data on the evolutionary history of obligatory interactions have been explicitly interpreted in the context of past climate changes. This may be due in part to the difficulty of timing biological events. However, focussing research efforts on particularly appropriate models may help resolve this issue. Biotic interactions, and in particular symbiotic and mutualistic interactions, are more prevalent in the tropics (Schemske et al., 2009). For instance, the number of parasitic species per host species and the proportion of plants producing extrafloral nectar are higher in the tropics (Coley and Aide, 1991; Guernier et al., 2004; Lindenfors et al., 2007). Moreover, species interactions in the tropics are globally more specific and specialized, probably because strong climatic variation at all time scales in temperate areas favours more generalist species (Dynesius and Jansson, 2000). The greater prevalence of obligatory interactions in the tropics might thus be caused by a higher stability of tropical ecosystems. It would be worth comparing prevalence of obligatory interactions in tropical ecosystems that differ in stability with respect to climate changes, such as riparian and mountain top ecosystems, in order to test for the role of stability in diversification of interactions. Appropriate biological models for studying the effect of climate change on obligatory interactions should thus be easier to find in the tropics. Although climate changes since the beginning of the Quaternary were less pronounced in the tropics than in temperate area, the effect on ecosystems was probably as dramatic, because tropical species are more specialized, and thus more sensitive to changes. Reconstruction of past climate fluctuations and shifts in distribution of ecosystems in the tropics are not as well-resolved as in Europe or North America. However, a growing body of studies is rapidly improving the resolution of climatic and vegetation reconstruction (Hessler et al., 2010; Lezine et al., 2013). Ant-plant symbiotic interactions (i.e., those between myrmecophytic plants and their specific ants, Fig. 1) have a set of features that make them good candidates as model systems. They are known only from the tropics but are widely distributed there. They are very diverse, involving several hundred plant and ant species, and encompass a large range of specificity between the ant and the plant, including highly specific interactions, allowing for comparative analysis. A highly interesting feature of these interactions is that they do not seem to be evolutionarily stable. Indeed, most ant-plant symbioses originate from independent events that rarely lead to evolutionary radiations (Davidson and McKey, 1993). It seems that this type of interaction can easily appear in the course of evolution, but can also easily break down. This is illustrated by attested cases of reversal from myrmecophytism to free-living in phylogenies of genera with myrmecophytes (Blattner et al., 2001; Davies et al., 2001; Razafimandimbison et al., 2005). Changes in environmental conditions, including climate fluctuation, are likely to drive breakdown of these mutualistic interactions. The influence of past climate fluctuations should thus be easier to detect in such interactions, because they can be highly specific and specialised, and appear to be particularly sensitive to environmental changes.
Acknowledgements
Funding source: C3A project, from the ‘Sixth extinction’ programme of the French ‘Agence nationale de la recherche’.