1. Introduction
Global warming, limited fossil fuel reserves, and urban pollution (transport being responsible for 30% of CO2 emissions) show the urgent need to move from an oil-based economy to one based on low-carbon energy. Such a need argues for seeking new sources of energy that are more environmentally friendly, such as renewable energies (solar, wind, geothermal, biomass, etc.) with a low CO2 footprint. It is important to point out that some of these energies are very abundant: for example, the sun sends 10,000 times more energy to our planet than we need. Remarkable technological advances in the development of photovoltaic cells have made it possible to better control the conversion of solar energy into electrical energy with high efficiencies (⩾20%) and low cost (⩽3 US cents per kWh). This energy source has thus become competitive with conventional energy sources such as coal or nuclear power. Renewable energy sources, however, are intermittent, leading to large fluctuations. Of course, neither the wind nor the sun blows or shines on command. It is therefore necessary to couple energy conversion systems to energy storage systems in order to be able to generate the desired electricity after the fact, on demand. Similarly, the irreversibly initiated transition from thermal vehicles to electric vehicles requires sufficient on-board energy capable of ensuring the autonomy of the vehicle, which again requires high-performance storage systems. The major challenge of the next twenty years will therefore not only be a problem of energy conversion, but also a storage problem, an indispensable step to better manage the energy resources of our planet.
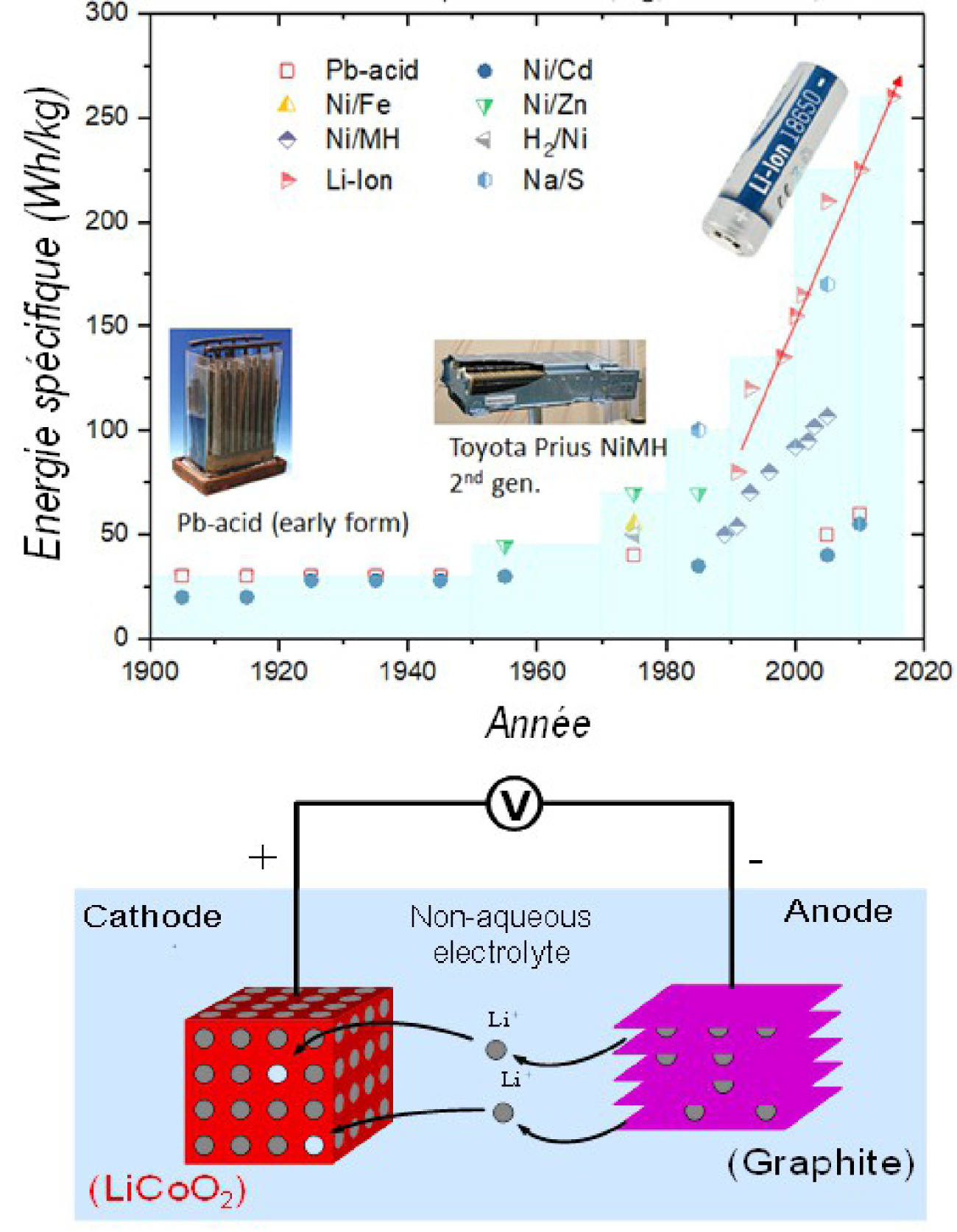
Evolution of specific energy for different battery technologies as a function of time (top) and operating principle of a Li-ion battery (bottom).
One of the most efficient ways to store energy and deliver it on demand is to convert chemical energy into electrical energy via electrochemical devices such as fuel cells and batteries. Among the latter, Li-ion technology is now attracting much media attention due to attractive performances that challenge those of previous technologies (Pb-acid, Ni–Cd or Ni–MH based on aqueous electrolytes) (Figure 1a). In comparison, the energy density (Wh/kg) of Li-ion batteries is three to five times higher on average than other technologies. Li-ion batteries rely on the use of insertion materials (Li + sponges) as positive and negative electrodes, separated by an organic electrolyte based on lithium salts which ensures the path of the ions between the two electrodes while also being an electrical insulator (Figure 1b). As for the electrons supplied during the connection of the positive and negative poles of the battery, they come from oxidation-reduction reactions occurring in tandem at the electrodes. These reactions involve the insertion/extraction of Li+ ions within the host structure of the electrode material with concomitant injection/consumption of electrons. The electronic structure (Fermi level position) and crystallographic structure of the electrode material (number of vacant sites to accommodate Li+ ions) governs its redox potential and electrical capacitance, respectively. These characteristics, associated with the extreme reducing power of lithium (−3.09 V/SHE), mean that the transition from an aqueous electrolyte to an organic electrolyte makes it possible to obtain a battery voltage that is 3 times higher, as well as an energy density (Wh/kg) [product of potential (V) in volts and capacity (Ah/kg)] of up to 240 Wh/kg or 500 Wh/l. In comparison, the energy density of the 200-year-old Pb-acid technology is now about 40 Wh/kg.
This slow progress in the field of batteries contrasts with the exponential increase in the number of transistors that can be integrated into microprocessors, governed by the famous Moore law (doubling of the density of transistors every two years). Moreover, as a model, where would we be if the capacities of the batteries had followed a similar evolution to that described by Moore's law? If we take the Pb-acid battery (G. Planted — 1860) as a starting point, the energy stored per 1kg of battery should have equaLled the energy of the first atomic bomb by 1908, continuously supplying humanity with energy for 1:20 hours in 1950 and for 50,000 years in 2006. Unfortunately, this was not the case; this field of research is highly dependent on the caprices of chemistry and the unfortunately limited number of electrons that can be reversibly extracted from a chemical bond. The tripling of the energy density of Li-ion technology in 30 years was therefore a long-term task [Tarascon 2016] that began in 1950. The pioneers of this work, J.B. Goodenough, M.S. Whittingham, and A. Yoshino, received the 2019 Nobel Prize in Chemistry for identifying electrode materials and electrolytes still present today in Li ion batteries. The success of this technology is such that these batteries are seen as one of the driving forces for the rapid development of electric vehicles and, in the longer term, for the storage — and therefore the massive use — of renewable energies.

Changes in the price per kWh stored over the years (top) and construction sites of the many "gigafactories" planned in Europe (bottom).
Apart from the Nobel laureates, another key man, Elon Musk, played an essential role in the revolution that the world of batteries is experiencing today. An unparalleled visionary with an atypical background from the world of engineering and business (founder of Zip2, SpaceX, CEO of SolarCity and then Tesla), he made a worldwide commitment in April 2015 to provide all human beings, wherever they live, with clean energy in large quantities and at low cost thanks to batteries. He thus launched the construction of the first manufacturing plant for batteries with a capacity of several dozen gigawatt-hours, called gigafactory, in Nevada (USA), with an annual production capacity of 5 billion cells. In addition, most of the plant is powered by solar energy. Thus, on the basis of these announcements and achievements, the price per stored kWh has been divided by 10 over the last ten years with the objective of falling below €100 by 2025 (Figure 2a). All this, combined with the constant improvement in chemistry, explains the current boom in the electric vehicle industry with more than 400 models in 2019, the Li-ion battery being the keystone of this technology.
The vision of E. Musk is spreading and such battery factories are emerging everywhere in the world, including among Asian giants and in Europe through the European Battery Alliance which wants to ensure energy independence for the cutting-edge automotive industry of the Union. The first European gigafactory was set up in Sweden and a dozen others are planned (Figure 2a), notably a Franco-German project proposing two factories, one in Germany and the other in France, supported by European manufacturers in the industry (SAFT, Solvay, Siemens and others). Thus, the total amount of energy stored by Li-ion batteries in Europe is expected to increase from 10 GWh to 400 GWh at least over the next decade. This development of electric mobility is an asset to reduce our CO2 footprint, under the necessary condition that the electrical energy recharging these batteries comes from renewable energies or even from nuclear power plants, not from coal-fired thermal power plants (large CO2 emitters).
Although it is dominant, Li-ion technology, which has been on the market since 1990, can still be improved, particularly in terms of its energy density, its lifetime, its safety, and its environmental footprint. All these aspects make batteries a diverse, exciting, and innovative research topic. The format of this article means that I will not be able to present all the current and past work on batteries. The works described below have therefore been selected to give an overview of current key research studies, both applied and futuristic. These are aimed at (i) increasing the energy density of Li-ion technology via new material concepts, (ii) designing more eco-compatible batteries such as Na-ion batteries, and (iii) developing smart batteries via the injection of new functionalities. The issue of recycling is then addressed.
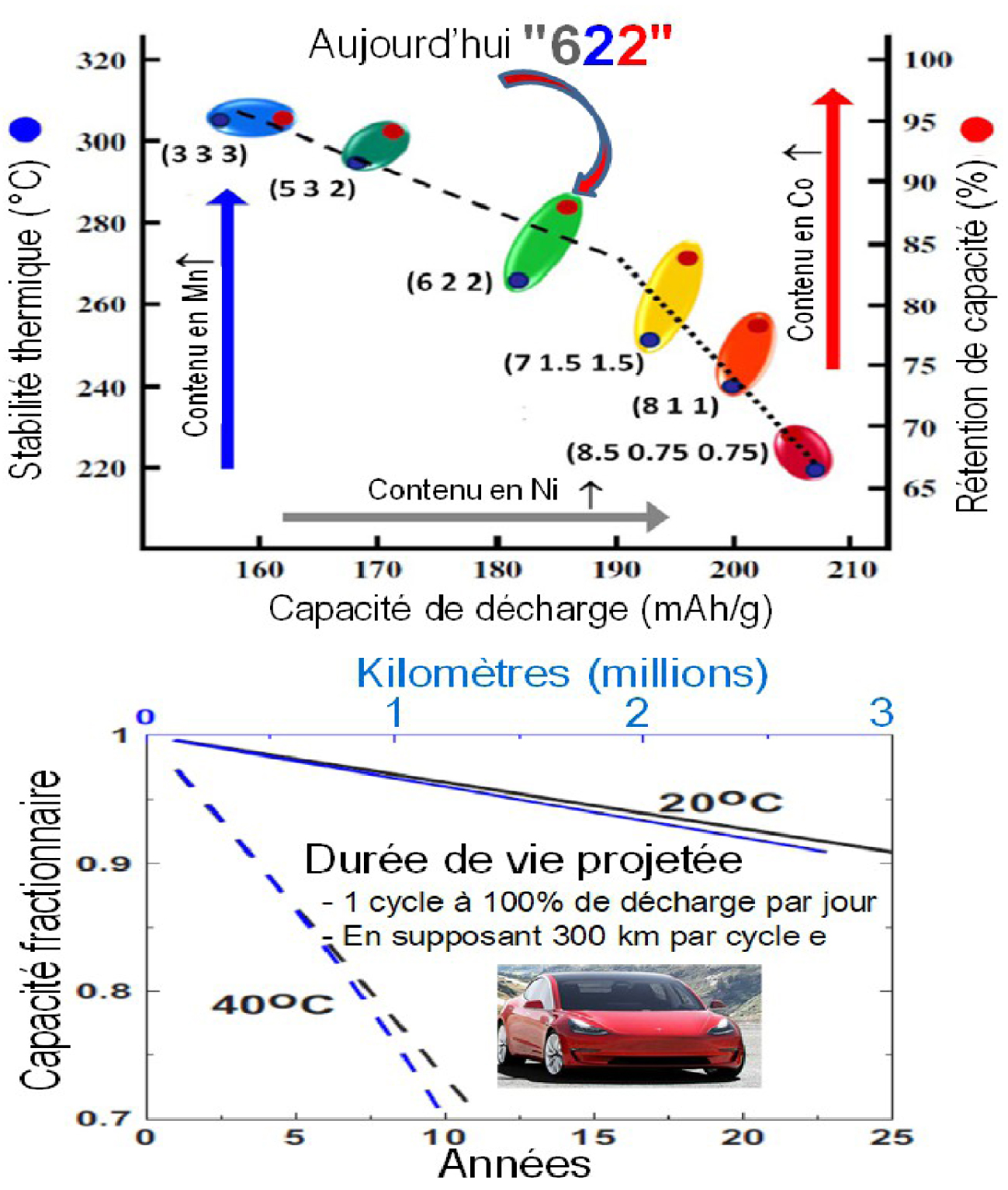
Low-cobalt lamellar oxides for the next generations of electric vehicle batteries (left). To date, LiNi0.6Mn0.2Co0.2O2 (622) is the most widely used composition. An estimate, derived from accelerated tests over 3 years (see text) on oxide-based Li-ion batteries (532), suggests a lifetime of 25 years at 20 °C and a cumulative distance of 3 million km assuming a discharge of 100% and 300 km per cycle.
1.1. Increasing the energy density of Li-ion technology
Many families of Li-ion batteries are currently being developed. They are all based on the use of graphite as a negative electrode, but differ in the nature of the positive electrode. The compounds most used for electrical mobility are oxides with lamellar structure Li(Ni1/3Mn1/3Co1/3)O2 and Li(NixCoyAl1−x−y)O2, denoted NMC and NCA respectively [Padhi et al. 1997]. These batteries, due to a high gravimetric energy density, dethrone those using phosphate-based positives (LiFePO4) that are more attractive in terms of safety and environmental impacts. However, a concern with the NMC and NCA electrodes is based on their high levels of Co, a very sparse chemical element whose mining raises ethical concerns. Electrode materials with low Co levels are becoming increasingly popular. The actors in the domain are developing lamellar phases [Rozier and Tarascon 2015] of type 811, (these figures indicating the proportions of Ni/Mn/Co in the material: LiNi0.8Co0.1Ni0.1O2) which is more reduced in Co than the phases 622 (LiNi0.6Co0.2Ni0.2O2) currently marketed (Figure 3a), as well as new lithium-rich phases of type Li(Li1.2Ni0.3Co0.13Mn0.54O2 [Luand Dahn 2002; Thackeray et al. 2007] which, in addition to low Co levels, have heightened capacities. These last phases whose capacity is based on a new concept of anionic redox, updated for the first time in the group that I lead at the Collège de France [Assat and Tarascon 2018; Sathiya et al. 2013], suggest a 20% increase in the gravimetric density of the cell. However, technological barriers related to potential drops during cycling, as well as hysteresis problems negatively influencing the performance in terms of power and energy efficiency of these materials, remain to be addressed in order to concretely commercialise these new materials that are rich in Li [Assat and Tarascon 2018].
The electrode-electrolyte interfaces that govern performance in power, durability, and calendar life are also the subject of intense studies. The challenge is significant because of the dynamic changes in these interfaces during cycling and the aging of the battery. However, thanks to refined research on the stabilisation of electrolytes through the addition of additives combined with the development of operando characterisation techniques with excellent resolution in time and space, we are now mastering these interfaces as never before. As a result, battery life has been improved. Indeed, a recent study [Harlow et al. 2019] of three years of tests on Li-ion cells of type NMC(532)/C, at various temperatures, shows that the lifetime of the batteries of electric vehicles could allow them to reach 3 million kilometers travelled at 20 °C, i.e. a lifetime of 25 years assuming a discharge of 100% per day (Figure 3b). Furthermore, on the basis of the progress made in terms of lamellar oxides with the addition of Li-rich compounds as well as on the C electrode via the addition of Li, obtaining an energy density of around 700–800 Wh/l and 350–400 Wh/kg is envisaged for 2025.

Advantages of all solid with Li metal anode versus liquid and solid Li-ion (top), and (middle and bottom) Japan's long-term forecasts for all-solid technology as well as (bottom) an estimate of overall electric and hybrid vehicle sales.
Nevertheless, the question remains whether we will be able to go beyond 800Wh/l. Much hope rests on the so-called "all-solid" batteries, which are popular in the world of electric car manufacturers. For example, Toyota already announced in 2017 that it planned to compete with Tesla cars in 2022 with batteries of this type featuring fast charge. This is a revival for this technology which was originally developed by J. Akridge at Eveready@ in 1986 [Jones and Akridge 1992] in a thin-film version (TiS2/LiPON-LiI/Li.) The discovery by Kanno in 2011 [Kanno and Murayama 2001] of Li10GeP2S12 thiophosphate with an exceptionally high ionic conductivity of 10−2 S/cm2, comparable to or even greater than that of a liquid electrolyte, rekindled interest in all-solid batteries. It was a true trigger, to the point that it is difficult to find a laboratory, a battery manufacturer, or a car manufacturer who does not currently work on solid batteries. These batteries are on the roadmap of all Asian institutions and companies, including Japan, which anticipates a significant penetration of the automotive market by 2030 (Figure 4b). Europe is also mobilising with the launch of many projects under the H-2020 programmes. These batteries would be potentially safer because of the absence of liquid electrolyte, but also more attractive in terms of volumetric energy because of the use of metallic Li instead of the graphite carbon negative electrode (Figure 4a). They are also of interest because of the possibility of assembling them in a bipolar configuration. However, this euphoria is beginning to fall back due to the growing awareness of the recurrent difficulties related to interface instability and in particular due to the potential window limited by the thermodynamic stability of the ionic conductors, whether those of the family of thiophosphates (Li3PS4, Li10GeP2S12) or garnets (Li7La3Zr2O12 [Thangadurai et al. 2014]. The problem of dendritic growth at the Li electrode, which led to the abandonment of Li-metal liquid electrolyte technology in the 1980s, has not yet been fully resolved. The diffusion of Li, which is slower in the metallic Li than in the ionic conductor, causes the appearance of porosity at the interface that is conducive to the formation of these dendrites. To date, this problem cannot be completely solved despite the use of surface treatments and maintaining the battery under mechanical pressure. Equally complex is the control of the lamellar oxide-ionic conductor interface at the positive electrode, both in terms of the chemical compatibility of the solid electrolyte with the active electrode material and in terms of its elasticity to absorb the repeated volume changes associated with the insertion/extraction of Li+. Removing these scientific and technological barriers remains a major challenge that concentrates the efforts of many research groups around the world. The research directions pursued are focused on various coating methods, including the deposition of oxide buffer layers to minimise decomposition reactions, alloys to ensure better wettability of the Li metal / electrolyte interface, and finally thin layers of hybrid (inorganic-polymer) ionic conductors to ensure elasticity of the interface without affecting ionic transport [Famprikis et al. 2019]. Although some studies are promising, the problem of interfaces within all-solid batteries is far from being mastered. This gives a somewhat unrealistic appearance to some announcements announcing the all-solid battery for 2022 (a date which has already been postponed). In summary, all-solid batteries are promising, but the road will be long, both at the fundamental and applied levels, before they can be commercialised. Let us remain humble and not repeat the history of Li-air technology which was largely oversold only to be totally forgotten 5 years later.
1.2. Design of more eco-compatible batteries
In addition to battery life, its eco-compatibility is of paramount importance for societal and global reasons. We would be very naïve to think that a battery perfectly follows the principles of sustainable development. This fear is reinforced by analyses of the batteries' energy life cycle, which reveal that 400 kWh of energy is needed to manufacture a Li-ion battery storing 1 kWh per cycle. This is accompanied by the emission of about 75 kg of CO2 [Larcher and Tarascon 2015]. Such an observation has led to a reorientation of our research themes which are currently focused on exploring new concepts of electrode material design based on abundant elements and designed using less energy-intensive synthesis processes. Research is also interested in the development of new chemical compositions, beyond Li, to minimise the environmental footprint of battery production. The challenge is also to answer legitimate questions about the available lithium reserves in the world, given the growing demand for this element by the automotive industry and the deployment of renewable energies. Moreover, these reserves are located in a limited number of countries, some of which are geopolitically at risk, with the fear that the price of Li will fluctuate like that of black gold [Tarascon 2010]. These concerns have led to the development of new metal-air technologies (Li-O2, Zn–O2), Li-S, Na-ion, Mg(Ca)-ion, Zn–MnO2 and "Redox-Flow" which, although described as "new", are all based on already known chemistries, but neglected for various reasons.

Cycling and self-discharge performance of Na-ion 18650 prototypes based on Na3V2(PO4)2F3 electrochemistry assembled by the company TIAMAT.
Of the chemistries mentioned above, the most mature and interesting in the context of sustainable development is undoubtedly sodium. Indeed, Na resources are unlimited, accessible anywhere in the world, and exploitable at a very low cost. Moreover, although some criteria may appear to be disadvantageous, in particular mass criteria (Na+ being three times heavier than Li+), these disadvantages are counterbalanced by the possibility of replacing the current collector of the Cu negative electrode in Li-ion technology with aluminum, which is lighter, cheaper, and does not form an alloy with sodium. Two Na-ion technologies are currently being developed. They share the same negative electrode made from carbon but differ in that one uses polyanionic compounds Na3V2(PO4)2F3 [Ponrouch et al. 2013] and the other uses lamellar compounds Na2/3Mn0.5Fe0.5O2 [Yabuuchi et al. 2012] as a positive electrode. The Na3V2(PO4)2F3/C Na-ionsystem, currently developed within the French Research Network on Electrochemical Energy Storage (RS2E), is the most attractive. Thanks to efforts in the chemistry and morphology of the Na3V2(PO4)2F3 material coupled with the production of electrolytes based on specific additives [Cometto et al. 2019], we were able to obtain cycling performance in button cells over a wide temperature range (−15 °CC +55°CC +55°CC) that is unimaginable with Li-ion technology (Figure 5).
This Na3V2(PO4)2F3/C chemistry has been successfully integrated into 18650 cells within the recently created French Na-ion sector and supported by a start-up named TIAMAT. Cells of 120 Wh/kg with a long lifetime (>3000 cycles) and attractive power performance (5–10 W/kg) have been produced to date. Although Na-ion technology derives from Li-ion technology as it uses similar types of electrodes and electrolytes, it is not competitive with Li-ion in terms of energy (120 vs. 240 Wh/kg) due to the weight of Na, which is greater than that of Li, and its lower reducing character. Its role in high-energy-density applications, such as electric vehicles, will be limited. However, this low energy density is not a handicap for network applications where volume is not a constraint. A major advantage of the Na3V2(PO4)2F3/C technology remains its power performance. This is due to the three-dimensional structure of Na3V2(PO4)2F3 which has channels that allow for a rapid diffusion of Na + and thus complete discharges and charges in less than 5 minutes. It outperforms its Li-ion counterparts and even competes favorably with TOSHIBA's ultra-fast-charging Li-ion batteries (SCiB) — the benchmark in the field — at a lower cost per kWh [Tarascon 2020]. Thus, the Na3V2(PO4)2F3/C battery certainly has a key role to play in the automotive industry for functions with high power needs (48 V hybrid vehicle, recovery, and start/stop functions), as well as in the sector of network applications for frequency smoothing.
Another attractive aspect of Na-ion batteries is that, unlike Li-ion batteries, they can be discharged or kept at zero volts without any risk of impairing its subsequent performance. The reason lies in the fact that it is possible to use aluminum current collectors, which do not form an alloy with Na, unlike Li, with which they form an alloy. To mitigate this formation of alloys, we use a Cu-based current collector for Li-ion cells. However, it comes with the risk that it may oxidise when the cells are at zero volts, leading to the problem of explosions from the formation of Cu dendrites. As a result, Li-ion cells cannot be transported while being discharged, unlike Na-ion batteries: the latter are therefore exempt from any transport regulations.
To conclude, Na-ion technology is becoming a reality. However, this idea is not revolutionary. The proof of this is that in 1869, a French visionary writer, Jules Verne, had already identified the advantages of this battery technology in his novel "20,000 leagues under the sea". As is written in his book, "Sir, sodium alone is consumed and it is the sea that provides me with it. I will also tell you that sodium batteries must be considered the most energetic ". Although imagined for a long time, Na-ion technology is only emerging now and presents itself as an attractive option for greener and more sustainable batteries.
1.3. Towards smart batteries
In order to lower the environmental footprint of batteries but also the cost of stored energy, much hope relies on studies aimed at offering them a second life. The idea is that when they reach their end of life in an electric vehicle (with an end of life criterion corresponding to a 20% reduction in nominal capacity), they could, depending on their degree of wear, be brought back into compliance for network applications. This change in functionality is today only a utopian ideal (i) when we see the diversity of pack models, all different from each other, and the sophistication of battery management systems (BMS) for conservative but safe use and (ii) in the absence of traceability or the possibility of intervening in the health status of the batteries in a non-invasive way. Non-invasive detection methods aimed at providing a better knowledge of the processes taking place in batteries — their state of health in a way — are therefore necessary to give a second life to the battery. Our approaches are strongly inspired by the medical field. As such, we note the introduction of optical fibers equipped with Bragg gratings into our accumulators in order to obtain a temperature mapping of the interior of the battery via optical interrogation (wavelength shift of the signal emitted), as well as an evolution of its pressure during its formation stage, its cycling, or its use under high power conditions. Thanks to our current ability to decipher the chemical information contained in these optical signals in terms of side reactions, interface growth, and reaction kinetics [Huang et al. 2020], we can refine their chemistry through the addition of specific additives. The collection of this data over time will allow us to know the state of health of the batteries. This will establish a "medical monitoring" for each battery that will allow us to ascertain its degree of wear before assigning it an appropriate second life application.

Conceptual diagram of a battery of the future integrating diagnostic and self-healing functions.
It is quite obvious that as in the medical field, such a diagnosis only makes sense if it goes hand in hand with self-healing. We thus see the birth of a new science at the crossroads of several disciplines, dedicated to the self-healing of batteries by acting at the level of electrodes and separators via innovative grafting chemistry. A European program has just started with the aim of developing a system for delivering molecules on demand via physical stimuli. This will allow for solubilising a resistive deposit or injecting self-repairing polymers operating via supramolecular interactions in order to restore a defective electrode/electrolyte interface in the battery. For an illustrative example, inspired by cell membranes featuring ion channels that provide membrane transport in our cells and act as ion regulators, we used a protein channel inserted into a lipid membrane as a biological nanopore for the detection and selective regulation of transport of undesirable chemical species (Sx)n− within a battery, via the formation of inclusion complexes with cyclodextrin [Bétermier et al. 2020]. Moreover, the specific complexation properties of cyclodextrin give it the possibility of capturing or releasing chemical species over a narrow temperature range. Thus, the design of CD-functionalised membranes using temperature as a physical stimulus to regulate chemical species becomes possible.
The coupled diagnostic/self-healing approach is disruptive, so much that future batteries could have an additional analytical output besides the positive and negative poles (Figure 6) to communicate with a BMS, which, when coupled with an actuator, can trigger the desired stimulus to deliver the required treatment that addresses the identified problem. This advanced detection/self-repair will not only facilitate the second life of the batteries but will also be of crucial value for increasing their durability and reliability: an indirect way to significantly reduce their environmental footprint.

From collection (urban mine) to "direct recycling" via a short circuit (top) and a redesigned assembly introducing the concept of the lego-type battery of the future (bottom).
1.4. Recycling
The advent of Li-ion technology and the applications that have accompanied it - in terms of communication, electric mobility, renewable energies, drones, and medicine - is a revolution in the service of a better planet. However, it is essential not to repeat the mistakes of the past. One can recall that polymers, including plastics with the thousands of applications that followed its discovery in the mid-nineteenth century, initially delighted our societies before becoming a major problem today due to a complex recycling process that is not fully carried out to date. Although less covered by the press and the media, the chemistry of recycling, a term that is not very sophisticated and attractive, is inevitable and becomes an imperative. Billions of batteries will have to be recovered and to date we do not have a clear idea of how this will be done efficiently. Recycling sounds more like an old rather than a new science among scientists. This science is currently based on multi-stage pyro- or hydro-metallurgical processes [Harper et al. 2019] whose energy and solvent demand will have to be reduced. We need to rethink these recycling processes and encourage short circuits in a single stage. However, innovation is still possible in this area. Several teams are working on a process called "Direct Recycling" [Chen et al. 2016] involving a chemistry that preserves the structural elements of the electrode so as to allow for a simple annealing step (Figure 7a). Recycling will only make sense with efficient collection and recovery systems based on urban mines that are easy and less expensive to exploit. It is thus more economical to recycle the Co of the batteries than to extract it from the mines. In the same spirit, we note that only 28 tonnes of Li-ion batteries need to be treated to recover one tonne of Li, whereas 250 tonnes of spodumene (ore containing Li) or 750 tonnes of brine need to be handled in order to obtain the same quantity [Tetgar 2011]. The European standard that 60% of the weight of the battery must be recovered is not sufficient. It will need to be enhanced with the joint awareness of manufacturers and users that recycling should be seen as a value of the battery supply chain.
In addition to recycling processes [Ducts 2018], it is also necessary to rethink the configuration of the batteries, which has hardly changed in decades. It may be the time to design “LEGO” batteries where each battery component can be recycled independently (Figure 7b). This is not a fantasy; in the past, the mechanical recharging of Zn-air batteries by replacing the Zn electrode was put into practice. New processes and new configurations would allow recycling to make its own evolution and become attractive to future generations, as the cause is so noble.
1.5. Conclusions/perspectives
Because of its societal and planetary impact, Li-ion technology is now seen as a great discovery of the 20th century, and rightly so. I will answer the question from the beginning of this examination in the affirmative. Batteries are a good option for sustainable development, but only if they meet certain criteria. They include the need to (i) use primary energy from renewable energies to recharge them; (ii) develop higher-capacity, metal-abundant electrode materials through less energy-intensive processes; (iii) develop more eco-compatible battery technologies; (iv) inject intelligence into batteries through sensors and self-healing functionalities to extend the age of recycling and allow a second life for the battery; and finally (v) launch an aggressive recycling policy including short-circuit recovery processes and the establishment of strict standards. These are fundamental scientific challenges resulting from the existence of concrete technological barriers which will require, in addition to a multidisciplinary approach, a profound synergy between fundamental and applied research, thus strengthening the interest of this field of research.
Although there is a well-performing research happening in Europe, 95% of the battery market is in Asia. This is an uncomfortable situation for the automotive Europe, which in the race for electric mobility will be dependent on Asia for its supply. To respond to this, an industrial policy for the manufacturing of batteries is being implemented in Europe through projects to construct giant "gigafactories". Although this brings some sovereignty to the storage, it is still a defensive position that will always give it a spectator role. Ultimately, in order to obtain a substantial share of this lucrative electric mobility and renewable energy market, Europe will have to be more offensive. The aim will be to put in place disruptive and innovative research strategies over time, aimed at adding value to batteries. This can be achieved through new paradigms mobilising systemic approaches that involve new research sectors and industrial actors. Current industrial investments in gigafactories must go hand in hand with investments in the academic sector if we are not to repeat history and see Asia's supremacy once again assert itself in the years to come. We cannot insist enough on the importance of synergy between scientific and technological knowledge to securing a total European value chain.
The recent launch of the European flagship "Battery 2030+", whose three founding pillars are (i) the use of artificial intelligence for the accelerated discovery of new materials and interface control, (ii) the development of new diagnostic/self-repair strategies for the design of smart batteries, and (iii) the development of new recycling concepts and battery configurations, is proof of Europe's awareness of the efforts needed to conquer leadership in the field of batteries. We can only rejoice at this and work enthusiastically to succeed in this endeavour, the challenge of which for France and Europe will be to regain true energy independence.
Acknowledgements
I would like to thank Benjamin Campech for his advice and feedback given to this article.