1. Introduction
The late Neoproterozoic to early Cambrian was an important time interval in the geological evolution of the Gondwana supercontinent because it was the period of assembly of this supercontinent as well as subduction initiation in the Peri-Gondwana margin [Cawood and Buchan 2007, Cawood et al. 2007, Collins and Pisarevsky 2005, Li et al. 2008a,b, Meert et al. 2003, Murphy et al. 2011]. It is also proposed that the eastern Gondwana assembly took place during 570–510 Ma, and subduction initiation in the Gondwana proto-Pacific margin happened during 580–550 Ma [Cawood and Buchan 2007, Meert et al. 2003]. Subduction of the proto-Tethyan oceanic lithosphere during Late Neoproterozoic to Early Cambrian along the northern margin of Gondwana was responsible for the arc magmatism which occurred along the northern part of the Gondwana supercontinent [Nadimi 2007, Ramezani and Tucker 2003, Ustaömer et al. 2009]. The Indian proto-Tethyan margin witnessed the formation of extensively distributed Early Paleozoic granitoids thought to be emplaced during the final assembly of Gondwana [Baig et al. 1988, Gaetani and Garzanti 1991, Meert and Van Der Voo 1997]. Cawood et al. [2007] proposed the possible existence of the “North India Orogen” in the Early Cambrian (530-490 Ma) and interpreted the granitoids as a result of an Andean-type magmatism due to the southward subduction of the proto-Tethyan lithosphere below the Indian plate. Continued southward subduction of proto-Tethyan lithosphere produced gravitational sinking which led to slab rollback resulting in asthenospheric upwelling and back-arc extension along the northern margin of Gondwana [Gürsu et al. 2015, Zhu et al. 2012]. This type of magmatism was reported from the Australian proto-Tethyan margin (Kalkarindji basalts, in Northeast Australia), south-western part of Turkey [Gürsu and Göncüoglu 2005] and northern Arabian plate [Gürsu et al. 2015]. Gaetani and Garzanti [1991] argued that the Early Paleozoic granitoids might have been associated with the formation of the Gondwana supercontinent while others (e.g. [Hughes and Jell 1999, Murphy and Nance 1991]) questioned the supercontinent breakup. Concerning the evolution of the early Paleozoic granitoids, either the late Pan-African orogeny [Islam et al. 1999, Valdiya 1995] or mantle involvement, due to the presence of dark micro granular enclaves [Le Fort et al. 1986, Miller et al. 2001] are proposed. The knowledge of the processes responsible for these intrusions is important for the evolution of the pre-collision margin of northern India, but it still remains controversial.
The Himalayan Mountain Belt has experienced several phases of mafic magmatism ranging in age from late Precambrian to Cenozoic periods [Bhat 1987]. These magmatic activities can be found as volcanic flows and mafic dykes throughout the belt. The Precambrian mafic magmatic rocks are best exposed in western Himalaya and are well studied compared to their eastern counterparts [Ahmad and Bhat 1987, Ahmad and Tarney 1991, Ahmad et al. 1999, Srivastava and Sahai 2001]. Geochemical characteristics imply that these magmas were derived from an enriched lithospheric mantle source in an extensional tectonic setting with variable degrees of partial melting [Ahmad 2008].
In the eastern Himalaya, geological and geochemical investigations have been conducted on the Abor mafic volcanic rocks of the Siang Window [Acharyya 1994, Ali et al. 2012, Bhat 1984, Bhat and Ahmad 1990, Jain and Thakur 1978, Kumar 1997, Liebke et al. 2011, Sengupta et al. 1996, Singh et al. 1993, 2006, 2007, Singh and Singh 2012, Singh et al. 2019], and the associated felsic volcanics [Singh and Singh 2012, Singh et al. 2019, Talukdar and Majumdar 1983]. However, the age and geochemical evolution of the mafic intrusives have not been studied in detail despite their importance in understanding the nature of magmatism and the formation of new crust at the eastern tip of the Indian sub-continent. Also, their petrogenetic relationship with the mafic and felsic rocks of Abor Volcanics is still unknown. One of such intrusions occurs in the western part of the Siang window where it is exposed in association with carbonates of the Buxa formation. This mafic intrusion is interpreted to have been formed in an extensional setting, and a late Neoproterozoic age has been assigned on the basis of microfossils – Rivularia haematites and Filamentous cyanobacteria – present in the dolomites of the Buxa formation [Singh and Tewari 2010]. In this paper, we present for the first time U-Pb zircon ages and new whole-rock geochemical data of the mafic intrusions from the Pangin area of the Siang window, eastern Himalaya in order to understand their petrogenesis and emplacement mechanism.
2. Geological background
The Himalayan mountain range of India is geographically classified into (i) Siwaliks which occupy the footwall of Main Boundary Thrust (MBT), (ii) Lesser Himalaya bounded by MBT in the south and Main Central Thrust (MCT) in the north and (iii) Higher Himalaya between MCT and South Tibetan Detachment (STD) (Figure 1; Hodges et al. [2000] and Yin [2006]). The eastern Himalaya is a ∼350 km long fold-thrust belt which extends from the eastern part of Bhutan up to the Dibang and Lohit valleys of Arunachal Pradesh. The Siang valley is a part of the eastern Himalaya and major litho-tectonic units show a bend in their regional strike from NE-SW in the west to NW-SE in the east throughout the Siang gorge. This unit is known as the Eastern Syntaxial Bend (Figure 1b; Wadia et al. [1931]). The folded core structure of this syntaxis is also known as the Siang antiform [Singh et al. 1993] and the Siang window [Acharyya 1998]. Acharyya [1998] suggested that the Siang window evolved during the southern exhumation of the Higher Himalayan Crystalline Complex and the southward thrusting of the Tertiary Himalayan foreland basin.
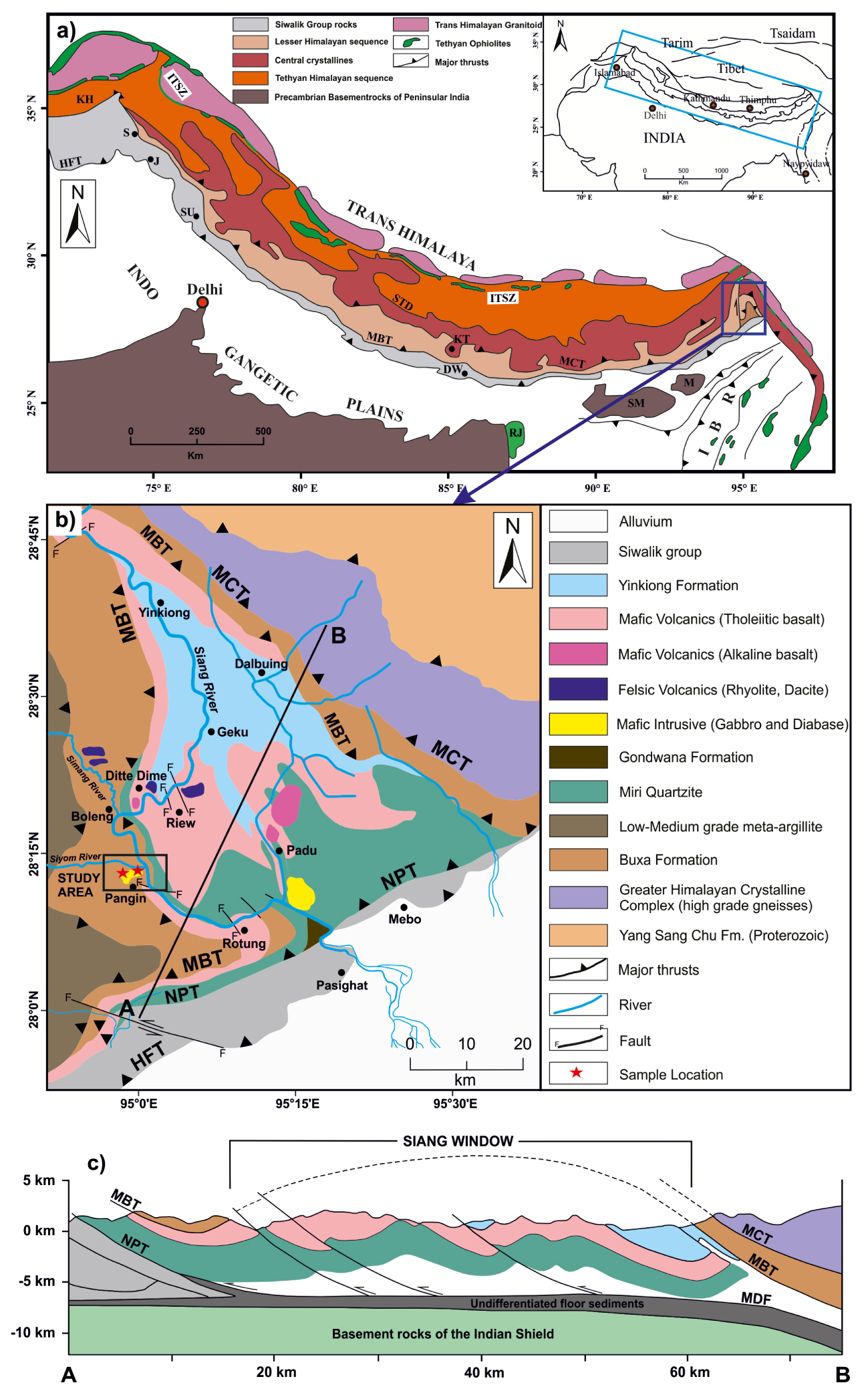
(a) Map showing a broad framework of the Himalaya bounded by major thrusts (modified after Acharyya [2007]; the upper insert shows the location map of the Himalayan mountain range. Abbreviations: KH – Kashmir-Hazara Syntaxis, S – Salal, J – Jammu, SU – Subathu, DW – Dwarkhola, KT – Kathmandu, RJ – Rajmahal Volcanics, SM – Shillong Massif, M – Mikir Massif, IBR – Indo Burma Range, HFT – Himalayan frontal thrust, MBT – Main boundary thrust, MCT – Main central thrust, STD – South Tibetan Detachment and ITSZ – Indus Tsangpo Suture Zone. (b) Geological map of Siang window (modified after Acharyya [2007], Singh and Singh [2012], Singh et al. [2019]); NPT – North Pasighat Thrust. (c) Structural cross-section along AB (modified after Acharyya [2007]); MDF – Main Detachment Fault. Masquer
(a) Map showing a broad framework of the Himalaya bounded by major thrusts (modified after Acharyya [2007]; the upper insert shows the location map of the Himalayan mountain range. Abbreviations: KH – Kashmir-Hazara Syntaxis, S – Salal, J – ... Lire la suite
The lithological association of the Siang window comprises the Miri-Buxa Groups of meta-sedimentary interbedded rocks that are folded with the Abor volcanics and Yinkiong sedimentary formations (Figure 1b). The lithological units inside the window are dissected by numerous normal faults that are oblique to both North Pasighat Thrust (NPT) in the southern part and MBT in the northern part of the window [Acharyya 2007, Kumar 1997]. These faults control the duplex geometry and the antiform nature of the Siang window [Acharyya and Sengupta 1998] (Figure 1c). Acharyya [2007] reported NPT as the southern continuity of MBT and another arm in the window’s roof. The Abor volcanics which occupy the core of the Siang window are grouped into (i) mafic volcanics which are found as lava flows and include basalt, agglomeratic basalts, sills, dykes with minor amount of tuffs, volcanic breccias, lapilli and pillow basalt; (ii) felsic volcanics such as rhyolite, dacite and welded tuff; and (iii) mafic intrusives including gabbro and diorite. The Buxa formation in the western part of the window consists of quartzite, dolomite, mafic intrusives and fossiliferous carbonates [Kumar 1997, Singh et al. 1993, Singh 1999]. This formation is surrounded by the Higher Himalaya and further succeeded by the Yang-sang-chu formation in the north [Singh et al. 1993]. The Abor Volcanics are underlain by the Miri Quartzite which is mainly white, purple and pink-coloured mature quartzite. The Miri quartzites have also been intruded by mafic rocks near its boundary with the volcanics (Figure 1b) [Acharyya 2007]. The Yinkiong Formation overlying the Abor Volcanics is composed of an alternating sequence of dark grey sandstone to siltstone and green and red shale with rare orthoquartzites and volcanic rocks [Jain and Tondon 1974]. This formation has been assigned an early to mid-Eocene age on the basis of foraminiferal assemblages [Acharyya 1994]. The Buxa and Miri formations are intruded by mafic rocks that are exposed in Pangin [Singh 2012], situated to the west of the Siang Window and the Siang Valley, respectively (Figure 1b).
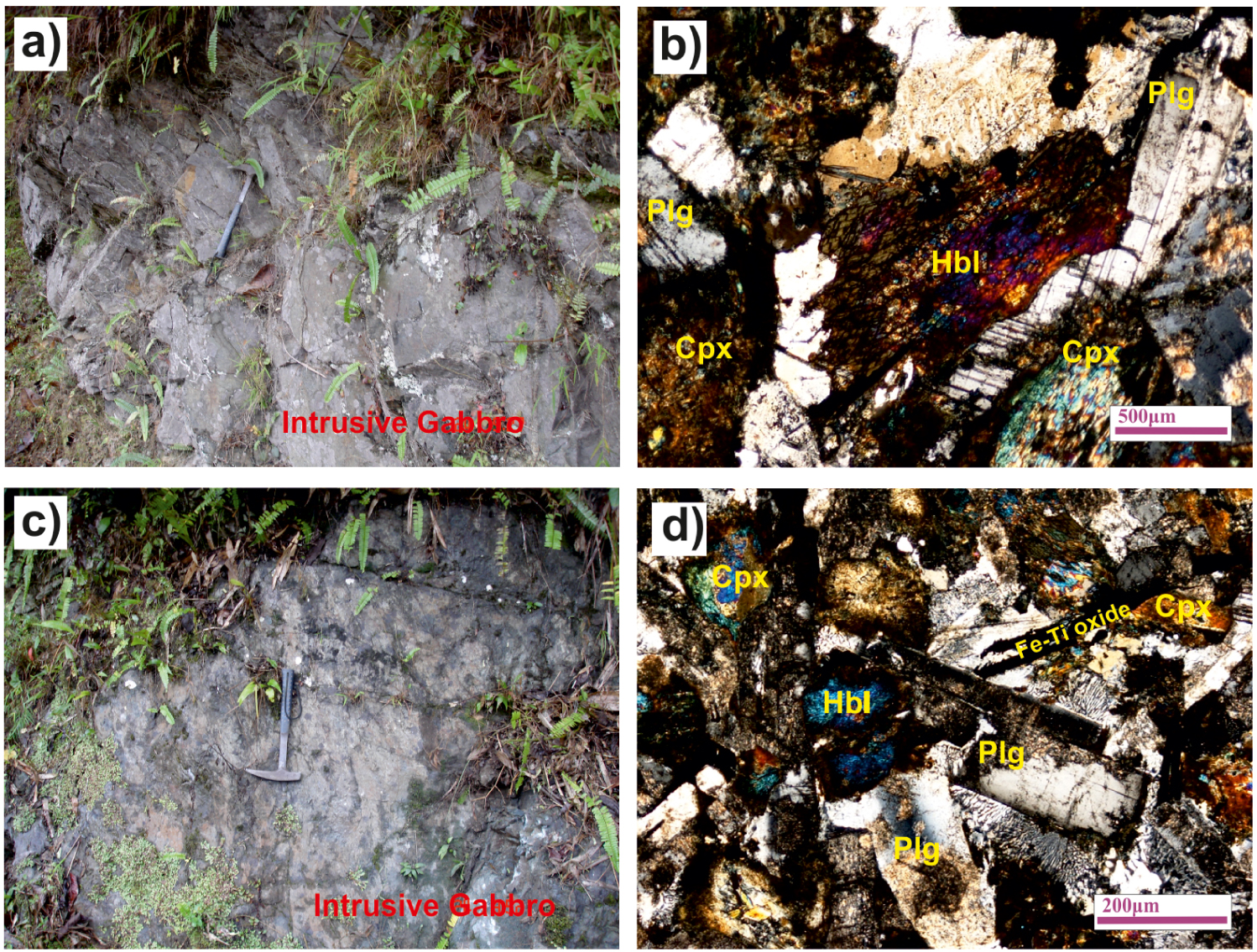
Representative field photographs and photomicrographs of the mafic intrusive rocks from Pangin area of the Siang Window, Eastern Himalaya, Northeast India – (a, c) intrusive medium-grained gabbro in the Buxa formation of Pangin; (b, d) granular and interlocking textures observed in intrusive gabbros with subhedral laths of plagioclase and stumpy clinopyroxene. Abbreviations for minerals are: Cpx – clinopyroxene, Hbl – hornblende, Plg – plagioclase and Fe–Ti oxide – Iron and titanium oxide.
3. Sampling and petrography
We collected the mafic rocks that intruded in the Buxa Formation of the Pangin area in the Siang window, eastern Himalaya (Figure 1b). Outcrops are 1–2 km long and 500–1000 m wide and show discordant contacts with country rocks. Sampling was done along the road sections of the Rotung–Pangin–Geku section and relatively fresh and unweathered samples were collected (Figure 2a, c) and the coordinates are 28° 13′ 54.4′′ N, 94° 59′ 33.5′′ E. Deformation features and primary flow structures are not observed. There is an increase in grain size from the contact margin to the core of the mafic intrusive bodies. No xenoliths of country rocks were observed in the outcrops, suggesting the intrusive nature of their emplacement. The mafic intrusives are characterized by medium to coarse-grained minerals comprising clinopyroxene (∼40–50%) and plagioclase (∼50–60%) as the major phases with minor amounts of hornblende, sericite, chlorite and Fe–Ti oxides showing granular textures. Interlocking textures between plagioclase and clinopyroxene were also observed (Figure 2b, d). Clinopyroxene is euhedral to subhedral in shape, has high relief and imperfect cleavages. Plagioclase grains are subhedral to anhedral and short columnar to granular in shape. In some places, the appearance of granophyric textures indicates that the plagioclase has been subject to some intergrowth (Figure 2d). All these petrographical observations clearly indicate that the intrusives are primarily gabbroic in nature and have undergone few alterations and low-grade metamorphism which might have caused the formation of hornblende and sericite.
4. Analytical techniques
4.1. XRF-ICPMS – major and trace element analysis
A pressed pellet samples preparation technique was adopted at the Wadia Institute of Himalayan Geology (WIHG), Dehradun, India, to determine the major element and few trace element concentrations for the selected samples using an X-ray Fluorescence Spectrometer (XRF; Bruker, Tiger S8). The analytical methods and data acquisition techniques of Saini et al. [2003] was adopted. Approximately 0.5 kg samples were cut into small chips and then crushed using a steel jaw crusher. In an agate mill, the crushed samples were pulverized up to 200 mesh following which pellets were prepared with approximately 7 g of each powdered sample and then analysed in the XRF. The loss-on-ignition (LOI) was measured after a 5 g sample powder was heated at 1000 °C in a Muffle furnace. The analytical precision is found to be better than ±2%–3% for major oxides and ±5%–6% for trace elements.
Rare earth elements (REE) and selected trace element concentrations were analysed using a Perkin-Elmer SCIEX ELAN DRC-e Inductively Coupled Plasma Mass Spectrometer (ICP-MS) at WIHG, Dehradun, India. The analytical procedure of Khanna et al. [2009] was adopted. The open-system digestion method was used for the analysis. Approximately 0.1 g of powdered sample was mixed with 20 ml of HF+HNO3 (2:1 ratio) and ∼2 ml of HClO4 in Teflon crucibles. Then the crucibles were heated over a hot plate until the samples were fully digested and dried to form a paste. This was followed by the addition of 20 ml of 10% HNO3 to each sample which was left on a hot plate for 10–15 min until a clear solution was obtained. The clear solution was made up to 100 ml final volume with milli-Q water. BHVO-1 and JB-1a are used as reference standards; their accuracy of trace element ranges from 2% to 12% and precision varies from 1% to 8%. Whole-rock geochemical data of the studied samples are given in Table 1.
4.2. LA-MC-ICPMS – zircon U–Pb geochronology
Zircon grains were separated from two gabbroic samples (GKS-40 and 4X3-A) for the U–Pb geochronology studies. For separation of zircon, the sample was first crushed using a jaw crusher and a disk mill and then sieved up to 60 mesh size. This was followed by gravity separation using the Holman–Wilfley water table, isodynamic magnetic and heavy liquids separation method. Finally, the zircon grains were hand-picked under a binocular microscope at WIHG, Dehradun, India. About 50 zircon grains were mounted in per-fluoro-alkoxy alkane (PFA®) Teflon followed by sequential polishing with 8, 5, 3, 1 and 0.25 μm diamond paste to expose the zircon mid-section. Cathodoluminescence (CL) images of zircon grains were taken on a Zeiss EVO 40 extended pressure (EP) Scanning Electron Microscope (SEM) with a 2 minutes scan time and varying probe current of 10–20 nA.
In situ U–Pb zircon dating was performed by 193 nm excimer laser (UV Laser, Model Analyte G2, Cetec-Photon machine, Inc.), equipped with a high-performance HelEx–II sample chamber and a Multi-Collector Inductively Coupled Plasma Mass Spectrometer (MC-ICP-MS; Neptune Plus, Thermo Fisher Scientific, Inc.) at WIHG, Dehradun, India. The analyses were carried out with a spot diameter of 20 μm (the spots were positioned relative to the CL images), a repetition rate of 5 Hz, an energy density of 4 J/cm2 and 75% laser intensity. Instrumental conditions, analysis methods and data acquisition were similar to those described by Mukherjee et al. [2017]. For fractionation correction and calculation of results, we used the Z 91500 zircon standard (Thermal Ionization Mass Spectrometry [TIMS] normalization data 206Pb∕238U = 1062.32 ± 2.22 [2 sigma]; Wiedenbeck et al. [1995]) and the accuracy was later checked using the Plesovice zircon standard (Isotope Dilution [ID] TIMS 206Pb/238U age = 337.13 ± 0.37 Ma; Slama et al. [2008]) as the external standard. Standards were analysed four times, at the beginning and end of the session, another two analysed after every 10 analyses. Common Pb was corrected following the method of Stacey–Kramers [Stacey and Kramers 1975]. The isotopic data reductions were processed using Iolite software [Paton et al. 2011]. The Concordia diagrams and U–Pb age calculations were made using Isoplot R [Vermeesch 2018]. The uncertainties of isotopic ratios and ages for a single analytical spot are quoted at 2σ whereas the errors for the weighted average age are given at 2σ (95% confidence). The U–Pb zircon data are given in Table 2.
5. Results
5.1. Whole-rock geochemistry
Geochemical data for the studied gabbros are presented in Table 1. On a nominally anhydrous basis, SiO2 contents range from 49.39 to 54.95 wt%, Al2O3 from 14 to 15.39 wt% and MgO from 5.46 to 7.4 wt%. The samples are characterized by variable Fe2O3 (7.09–12.7 wt%) and TiO2 (0.97–1.93 wt%) contents and are generally sodium-rich (Na2O > K2O). They have low and variable Ni (36–63 ppm), Co (29–65 ppm) and Cr (91–169 ppm) values. In the classification diagram of (Na2O+K2O) vs. SiO2 [Cox et al. 1979], the samples plot in the field of gabbro (Figure 3a). All the samples plot within the sub-alkaline basalt field on Nb/Y vs. Zr/Ti diagram (Figure 3b) (after Winchester and Floyd [1977]). On an AFM diagram (Na2O+K2O–FeOt–MgO, after Irvine and Baragar [1971]), they exhibit a typical tholeiitic trend (Figure 3c). The samples show uniform chondrite-normalized rare earth elements (REE) patterns with varying light rare earth elements (LREE) enrichment (La/Yb)cn = 2.65–3.99 and slight Eu anomalies (Eu∕Eu∗ = 1–1.31) (Figure 4a). In the primitive mantle (PM) normalized spider-diagram for incompatible elements, the mafic intrusive rocks exhibit variable abundances. They are enriched in large ion lithophile elements (LILEs) but depleted in high field strength elements (HFSE) (e.g. Hf, Ti, Zr) and are characterized by depleted Nb relative to La (Figure 4b), with Nb/La ratios ranging from 1 to 1.5. They follow a trend similar to E-MORB (Figure 4a, b).
Major (wt%) elements and trace elements including rare earth elements (ppm) for the mafic intrusive rocks from Pangin area of Siang Window, Eastern Himalaya, Northeast India
Samples | GK5 | GK9 | GK13 | 4X3A | GK8 | GK14 | GK12 | GKS40 | 4X3B | GKS4 | GKS6 |
---|---|---|---|---|---|---|---|---|---|---|---|
SiO2 | 50.81 | 53.96 | 54.95 | 52.28 | 51.04 | 52.85 | 52.85 | 52.54 | 50.94 | 50.50 | 49.39 |
TiO2 | 1.60 | 1.58 | 0.97 | 1.51 | 1.58 | 1.50 | 1.53 | 1.60 | 1.93 | 1.93 | 1.66 |
Al2O3 | 14.60 | 14.97 | 14.04 | 14.76 | 14.85 | 14.71 | 14.77 | 15.39 | 14.00 | 14.28 | 14.92 |
MgO | 5.69 | 6.26 | 6.50 | 6.85 | 5.53 | 6.55 | 6.29 | 7.40 | 5.46 | 6.02 | 6.54 |
Fe2O3 | 11.19 | 10.47 | 7.09 | 10.76 | 11.01 | 10.79 | 10.66 | 10.39 | 12.38 | 12.70 | 12.27 |
CaO | 9.64 | 6.45 | 9.00 | 6.23 | 9.23 | 6.55 | 6.46 | 5.83 | 8.45 | 8.90 | 8.75 |
K2O | 0.38 | 0.89 | 0.28 | 0.93 | 0.48 | 1.01 | 0.88 | 0.84 | 0.73 | 0.75 | 0.71 |
Na2O | 3.17 | 3.93 | 4.48 | 3.56 | 3.13 | 3.53 | 3.60 | 3.67 | 3.18 | 3.15 | 3.09 |
P2O5 | 0.18 | 0.17 | 0.11 | 0.17 | 0.20 | 0.17 | 0.19 | 0.17 | 0.26 | 0.25 | 0.22 |
MnO | 0.14 | 0.14 | 0.07 | 0.12 | 0.14 | 0.11 | 0.13 | 0.14 | 0.16 | 0.16 | 0.15 |
LOI | 1.21 | 2.10 | 0.88 | 2.16 | 0.90 | 2.08 | 1.83 | 2.94 | 1.04 | 1.37 | 2.40 |
∑ | 98.61 | 100.92 | 98.37 | 99.33 | 98.09 | 99.85 | 99.19 | 100.91 | 98.53 | 100.01 | 100.10 |
FeOt | 10.07 | 9.42 | 6.38 | 9.68 | 9.91 | 9.71 | 9.59 | 9.35 | 11.14 | 11.43 | 11.04 |
Mg# | 50.18 | 54.22 | 64.49 | 55.77 | 49.87 | 54.60 | 53.89 | 58.52 | 46.63 | 48.43 | 51.36 |
Trace element (ppm) | |||||||||||
Sc | 27 | 18 | 45 | 20 | 27 | 21 | 22 | 18 | 28 | 27 | 32 |
V | 253 | 164 | 356 | 161 | 244 | 164 | 166 | 168 | 272 | 260 | 239 |
Cr | 93 | 78 | 24 | 91 | 169 | 138 | 98 | 97 | 154 | 150 | 109 |
Co | 35 | 29 | 18 | 35 | 38 | 35 | 29 | 65 | 39 | 42 | 62 |
Ni | 41 | 51 | 38 | 56 | 41 | 56 | 54 | 63 | 38 | 36 | 38 |
Ga | 21.26 | 23.34 | 17.42 | 22.77 | 20.84 | 22.93 | 22.79 | 24.64 | 21.94 | 23.20 | 22.20 |
Rb | 6.00 | 25 | 4.00 | 30 | 9.00 | 32 | 26 | 27 | 14 | 14 | 13 |
Sr | 316 | 311 | 153 | 335 | 318 | 359 | 308 | 312 | 292 | 293 | 362 |
Y | 22 | 22 | 21 | 24 | 21 | 25 | 23 | 25 | 27 | 30 | 27 |
Zr | 86 | 67 | 113 | 63 | 83 | 63 | 64 | 64 | 105 | 109 | 92 |
Nb | 8.10 | 6.60 | 7.00 | 7.10 | 7.70 | 6.90 | 6.90 | 6.90 | 9.30 | 9.50 | 8.30 |
Ba | 108 | 167 | 75 | 194 | 115 | 193 | 156 | 143 | 153 | 147 | 152 |
La | 6.53 | 4.54 | 13.54 | 5.05 | 6.65 | 5.16 | 4.90 | 5.57 | 7.80 | 9.20 | 7.84 |
Ce | 14.99 | 10.18 | 27.02 | 11.28 | 14.97 | 11.43 | 11.06 | 12.76 | 18.24 | 21.86 | 18.85 |
Pr | 2.33 | 1.53 | 3.62 | 1.69 | 2.34 | 1.72 | 1.70 | 1.86 | 2.78 | 3.27 | 2.81 |
Nd | 10.69 | 7.05 | 14.36 | 7.88 | 10.75 | 8.03 | 7.75 | 8.82 | 12.92 | 15.40 | 13.27 |
Sm | 3.23 | 2.34 | 3.51 | 2.68 | 3.22 | 2.70 | 2.58 | 2.93 | 3.87 | 4.72 | 4.03 |
Eu | 1.29 | 1.05 | 1.65 | 1.21 | 1.30 | 1.25 | 1.15 | 1.32 | 1.50 | 1.68 | 1.54 |
Gd | 3.86 | 2.96 | 4.24 | 3.38 | 3.87 | 3.46 | 3.27 | 3.78 | 4.63 | 5.63 | 4.95 |
Tb | 0.63 | 0.50 | 0.69 | 0.56 | 0.63 | 0.58 | 0.55 | 0.64 | 0.75 | 0.92 | 0.81 |
Dy | 3.68 | 2.97 | 4.27 | 3.36 | 3.71 | 3.42 | 3.29 | 3.86 | 4.36 | 5.45 | 4.82 |
Ho | 0.73 | 0.57 | 0.92 | 0.65 | 0.72 | 0.67 | 0.63 | 0.77 | 0.87 | 1.10 | 0.97 |
Er | 1.80 | 1.39 | 2.48 | 1.57 | 1.84 | 1.61 | 1.51 | 1.84 | 2.13 | 2.74 | 2.42 |
Tm | 0.25 | 0.18 | 0.36 | 0.21 | 0.25 | 0.21 | 0.20 | 0.25 | 0.29 | 0.39 | 0.34 |
Yb | 1.58 | 1.13 | 2.43 | 1.31 | 1.58 | 1.34 | 1.22 | 1.49 | 1.83 | 2.37 | 2.12 |
Lu | 0.22 | 0.15 | 0.36 | 0.18 | 0.23 | 0.18 | 0.17 | 0.20 | 0.27 | 0.35 | 0.31 |
Hf | 2.30 | 1.26 | 0.62 | 1.49 | 2.34 | 1.56 | 1.32 | 1.34 | 2.82 | 3.01 | 2.52 |
Ta | 0.46 | 0.35 | 0.42 | 0.40 | 0.46 | 0.24 | 0.36 | 0.44 | 0.59 | 0.82 | 0.43 |
Pb | 2.20 | 17.10 | 5.50 | 14.40 | 2.20 | 15.40 | 14.50 | 22.40 | 1.10 | 2.60 | 1.90 |
Th | 0.51 | 0.43 | 3.47 | 0.44 | 0.52 | 0.42 | 0.41 | 0.40 | 0.60 | 0.69 | 0.61 |
U | 0.13 | 0.09 | 0.47 | 0.12 | 0.13 | 0.10 | 0.09 | 0.08 | 0.14 | 0.16 | 0.16 |
∑ REE | 51.81 | 36.54 | 79.45 | 41.01 | 52.06 | 41.76 | 39.98 | 46.09 | 62.24 | 75.08 | 65.08 |
LREE | 39.06 | 26.69 | 63.70 | 29.79 | 39.23 | 30.29 | 29.14 | 33.26 | 47.11 | 56.13 | 48.34 |
HREE | 12.75 | 9.85 | 15.75 | 11.22 | 12.83 | 11.47 | 10.84 | 12.83 | 15.13 | 18.95 | 16.74 |
(Nb/La)N | 1.20 | 1.40 | 0.50 | 1.35 | 1.12 | 1.29 | 1.36 | 1.19 | 1.15 | 0.99 | 1.02 |
(Ta/La)N | 1.18 | 1.29 | 0.52 | 1.33 | 1.16 | 0.78 | 1.23 | 1.32 | 1.27 | 1.49 | 0.92 |
(Hf/Sm)N | 1.02 | 0.77 | 0.25 | 0.80 | 1.04 | 0.83 | 0.73 | 0.66 | 1.05 | 0.91 | 0.90 |
(La/Yb)cn | 2.96 | 2.88 | 4 | 2.77 | 3.02 | 2.76 | 2.88 | 2.68 | 3.06 | 2.78 | 2.65 |
(Gd/Yb)cn | 2.02 | 2.17 | 1.44 | 2.13 | 2.03 | 2.14 | 2.22 | 2.10 | 2.10 | 1.97 | 1.93 |
(Sm/Yb)cn | 2.27 | 2.30 | 1.60 | 2.27 | 2.26 | 2.24 | 2.35 | 2.18 | 2.35 | 2.21 | 2.11 |
Eu∕Eu∗ | 1.12 | 1.22 | 1.31 | 1.22 | 1.12 | 1.25 | 1.21 | 1.21 | 1.08 | 1.00 | 1.05 |
Mg# = 100 × (MgO/40.304/(MgO/40.304+FeO/71.839); Eu∕Eu∗ = (Eu)cn∕((Gdcn + Smcn)∕2); cn-chondrite; N-primitive mantle normalized. The FeOt values are recalculated based on the analysed
5.2. Zircon U–Pb geochronology
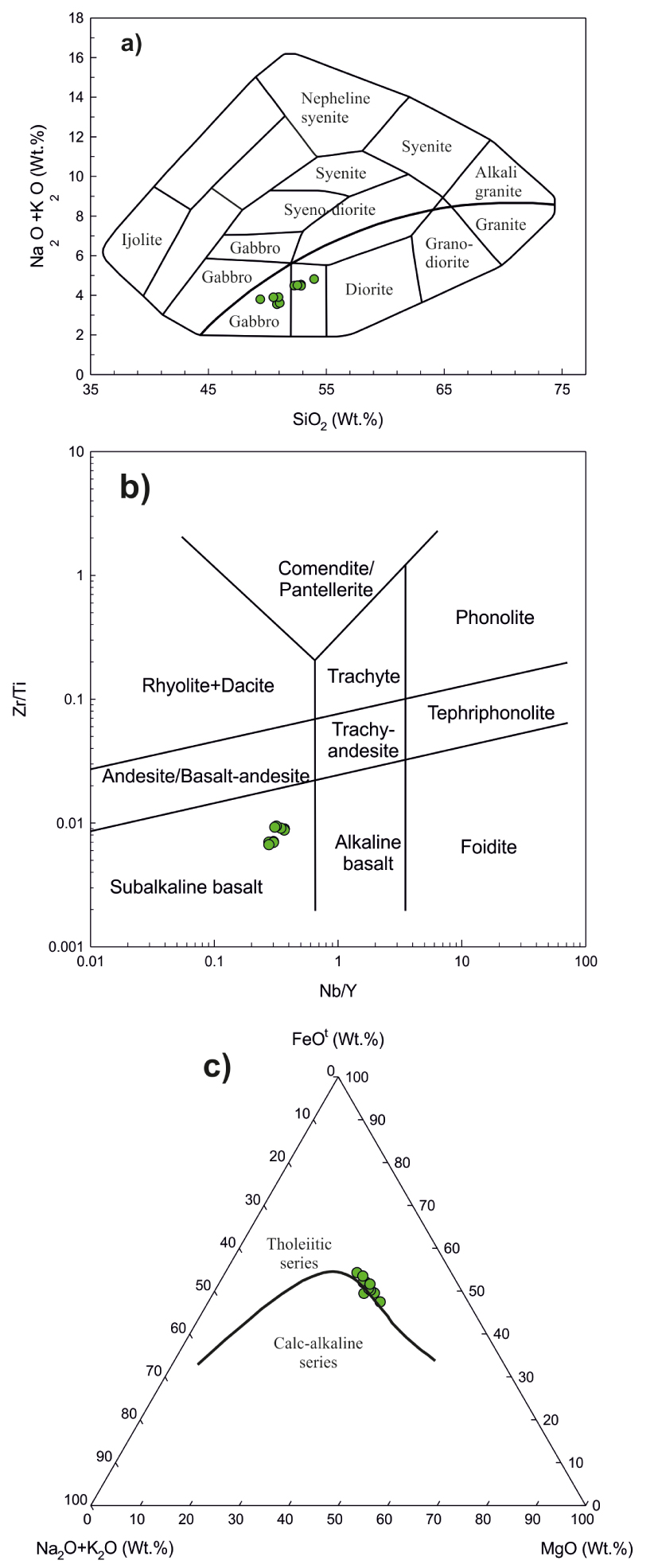
(a) SiO2 vs. Na2O + K2O (after Cox et al. [1979]); (b) Nb/Y vs. Zr/Ti (after Winchester and Floyd [1977]); (c) AFM diagram indicating tholeiitic signature (after Irvine and Baragar [1971]) for the mafic intrusive rocks from Pangin area of Siang Window.
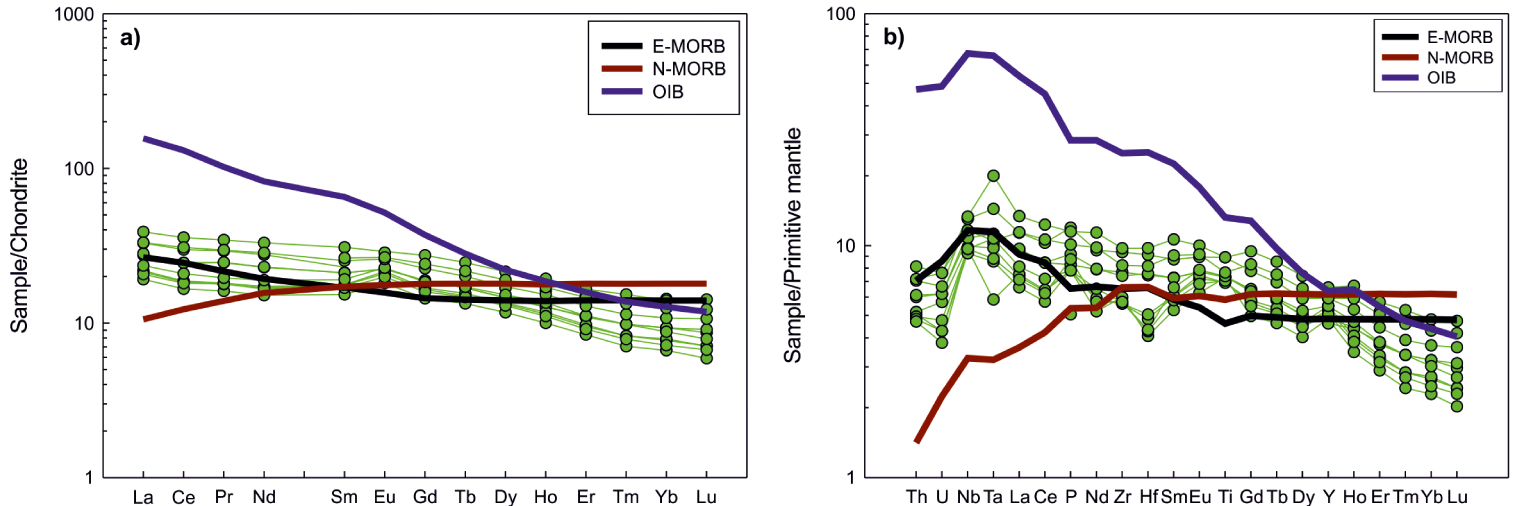
Chondrite-normalized REE pattern (a) and primitive mantle-normalized trace element pattern (b) for the mafic intrusive rocks from the Pangin area of the Siang Window. The normalizing values for chondrite and primitive mantle are from Sun and McDonough [1989]. Data for OIB, E-MORB and N-MORB are also from Sun and McDonough [1989].
Zircons in samples GKS-40 are colourless to translucent, euhedral to subhedral in shape, ∼80–200 μm long with length to width ratios varying from 2:1 to 3:1. In CL images, most zircon grains show clear oscillatory zoning and few grains show thin bright overgrowth rims (Figure 9a). A total number of sixteen spots were analysed on 15 zircon grains from this sample. The analytical data show a wide range of Pb (15.5–100.9 ppm), U (40.5–696 ppm) and Th (63.1–517 ppm) with Th/U ratios of 0.12–3.30 (Table 2). The CL images of zircon grains together with their high Th/U ratios imply a magmatic origin [Hoskin and Black 2000]. Out of the 16 zircon spots analysed, ten spots give 206Pb/238U concordia age of 568 ± 2 Ma (Figure 10a; MSWD = 0.0071), which can be considered to represent the magmatic event during the Late Proterozoic (Ediacaran).
LA-MC-ICPMS zircon U–Pb ages of the gabbro samples (GKS-40 and 4X3-A) from the Siang Window, Northeast India
Spots | Element content (ppm) | Th/U | Isotope ratio (±2σ) | Age (Ma ± 2σ) | ||||||||||||
---|---|---|---|---|---|---|---|---|---|---|---|---|---|---|---|---|
Th ppm | U ppm | Pb ppm | 207Pb/206Pb | 207Pb/235U | 206Pb/238U | 207Pb/206Pb | 207Pb/235U | 206Pb/238U | ||||||||
Ratio | ±2σ | Ratio | ±2σ | Ratio | ±2σ | Age (Ma) | ±2σ | Age (Ma) | ±2σ | Age (Ma) | ±2σ | |||||
Sample GKS-40: Gabbro, Lat. 28° 13′ 54.4′′ N, Lon. 94° 59′ 33.5′′ E, Concordia age of 10 spots = 567.66 ± 2.09 Ma (MSWD = 0.0071) | ||||||||||||||||
GKS-40-1 | 317 | 376 | 15.5 | 0.84 | 0.0481 | 0.0004 | 0.140 | 0.005 | 0.0211 | 0.0007 | 101 | 18 | 133 | 4 | 134 | 4 |
GKS-40-2 | 127 | 669 | 33.5 | 0.19 | 0.0580 | 0.0003 | 0.663 | 0.008 | 0.0826 | 0.0010 | 528 | 9 | 516 | 4 | 511 | 5 |
GKS-40-3 | 138 | 194 | 28.1 | 0.71 | 0.0578 | 0.0003 | 0.670 | 0.017 | 0.0837 | 0.0019 | 522 | 12 | 520 | 10 | 518 | 11 |
GKS-40-4 | 517 | 342 | 95 | 1.51 | 0.0575 | 0.0005 | 0.669 | 0.024 | 0.0867 | 0.0028 | 508 | 17 | 522 | 14 | 535 | 16 |
GKS-40-5 | 349 | 651 | 68.3 | 0.54 | 0.0579 | 0.0003 | 0.714 | 0.013 | 0.0889 | 0.0016 | 526 | 11 | 547 | 7 | 548 | 9 |
GKS-40-6 | 322 | 416 | 57.7 | 0.77 | 0.0579 | 0.0005 | 0.734 | 0.016 | 0.0920 | 0.0017 | 527 | 19 | 560 | 9 | 567 | 9 |
GKS-40-7 | 130 | 241 | 28.7 | 0.54 | 0.0567 | 0.0002 | 0.722 | 0.010 | 0.0922 | 0.0013 | 478 | 6 | 552 | 6 | 568 | 7 |
GKS-40-8 | 63.1 | 279 | 17.9 | 0.23 | 0.0617 | 0.0022 | 0.753 | 0.022 | 0.0886 | 0.0030 | 650 | 70 | 570 | 12 | 547 | 18 |
GKS-40-9 | 81.7 | 696 | 17.08 | 0.12 | 0.0593 | 0.0003 | 0.760 | 0.010 | 0.0927 | 0.0012 | 579 | 10 | 574 | 5 | 571 | 6 |
GKS-40-10 | 132.9 | 260 | 29.4 | 0.51 | 0.0581 | 0.0006 | 0.746 | 0.021 | 0.0926 | 0.0018 | 532 | 21 | 565 | 12 | 570 | 11 |
GKS-40-11 | 238 | 146 | 49.3 | 1.63 | 0.0574 | 0.0002 | 0.741 | 0.011 | 0.0933 | 0.0013 | 506 | 7 | 562 | 6 | 574 | 7 |
GKS-40-12 | 133.8 | 40.5 | 28.79 | 3.30 | 0.0649 | 0.0031 | 0.843 | 0.051 | 0.0939 | 0.0011 | 740 | 86 | 617 | 26 | 578 | 6 |
GKS-40-13 | 169 | 535 | 38.6 | 0.32 | 0.0648 | 0.0010 | 0.875 | 0.055 | 0.0949 | 0.0059 | 765 | 30 | 633 | 31 | 583 | 35 |
GKS-40-14 | 201 | 512 | 55.1 | 0.39 | 0.0632 | 0.0011 | 0.926 | 0.032 | 0.1103 | 0.0039 | 701 | 31 | 664 | 17 | 674 | 23 |
GKS-40-15 | 236 | 217 | 100.9 | 1.09 | 0.0714 | 0.0004 | 1.675 | 0.029 | 0.1725 | 0.0022 | 967 | 11 | 998 | 11 | 1026 | 12 |
GKS-40-16 | 138.1 | 244.1 | 65.8 | 0.57 | 0.0751 | 0.0002 | 2.177 | 0.020 | 0.2091 | 0.0020 | 1072 | 4 | 1173 | 6 | 1224 | 11 |
Sample 4X3-A: Gabbro, Lat. 28° 13′ 55.7′′ N, Lon. 94° 59′ 39.2′′ E, Concordia age of 08 spots = 521.50 ± 2.53 Ma (MSWD = 0.0011) | ||||||||||||||||
4X3-A-1 | 321 | 1250 | 50.2 | 0.26 | 0.0651 | 0.0007 | 0.482 | 0.014 | 0.0532 | 0.0015 | 775 | 21 | 400 | 9 | 334 | 9 |
4X3-A-2 | 178 | 339.7 | 35.4 | 0.52 | 0.0565 | 0.0002 | 0.599 | 0.016 | 0.0770 | 0.0023 | 473 | 8 | 476 | 10 | 478 | 14 |
4X3-A-3 | 301 | 567 | 61 | 0.53 | 0.0595 | 0.0011 | 0.653 | 0.022 | 0.0808 | 0.0020 | 579 | 38 | 509 | 13 | 501 | 12 |
4X3-A-4 | 163 | 717 | 32.6 | 0.23 | 0.0590 | 0.0003 | 0.679 | 0.013 | 0.0829 | 0.0017 | 566 | 12 | 525 | 8 | 513 | 10 |
4X3-A-5 | 86.8 | 574 | 18.2 | 0.15 | 0.0570 | 0.0003 | 0.661 | 0.010 | 0.0839 | 0.0015 | 489 | 13 | 515 | 6 | 519 | 8 |
4X3-A-6 | 220 | 185 | 45 | 1.19 | 0.0564 | 0.0003 | 0.652 | 0.009 | 0.0839 | 0.0010 | 468 | 11 | 509 | 5 | 519 | 6 |
4X3-A-7 | 135 | 626 | 28.5 | 0.22 | 0.0582 | 0.0003 | 0.689 | 0.012 | 0.0853 | 0.0014 | 535 | 11 | 531 | 7 | 527 | 8 |
4X3-A-8 | 281 | 416 | 56.6 | 0.68 | 0.0586 | 0.0005 | 0.691 | 0.015 | 0.0852 | 0.0015 | 549 | 17 | 532 | 8 | 526 | 9 |
4X3-A-9 | 232 | 462 | 53.5 | 0.50 | 0.0626 | 0.0026 | 0.704 | 0.023 | 0.0867 | 0.0018 | 649 | 69 | 540 | 14 | 536 | 11 |
4X3-A-10 | 38 | 145 | 10 | 0.26 | 0.0734 | 0.0085 | 0.885 | 0.097 | 0.0876 | 0.0046 | 860 | 170 | 632 | 51 | 541 | 27 |
4X3-A-11 | 297 | 1040 | 75 | 0.29 | 0.0615 | 0.0010 | 0.816 | 0.039 | 0.0955 | 0.0052 | 651 | 34 | 603 | 21 | 587 | 30 |
4X3-A-12 | 46.2 | 360 | 13.47 | 0.13 | 0.0629 | 0.0007 | 0.915 | 0.047 | 0.1053 | 0.0043 | 702 | 25 | 655 | 25 | 645 | 25 |
4X3-A-13 | 108 | 178 | 52.7 | 0.61 | 0.0750 | 0.0005 | 2.132 | 0.029 | 0.2074 | 0.0022 | 1068 | 13 | 1158 | 9 | 1215 | 12 |
4X3-A-14 | 124 | 87.4 | 88.8 | 1.42 | 0.1009 | 0.0005 | 4.245 | 0.041 | 0.3064 | 0.0027 | 1641 | 9 | 1685 | 6 | 1723 | 13 |
Note: Indicated by bold is used for Concordia diagram.
Zircons in sample 4X3-A are colourless or pale brown and transparent. The anhedral to subhedral grains show a near-prismatic or irregular morphology with a length of 50–200 μm and length to width ratios varying from 1:1 to 3:1. In CL images, most zircon grains show clear oscillatory zoning, and few grains show thin bright overgrowth rims (Figure 9b), which indicate magmatic origin. A total of 14 spots were analysed on 13 zircon grains from this rock. The analytical data show a high range of Pb (10–88.8 ppm), U (87.4–1250 ppm) and Th (25.8–321 ppm) with Th/U ratios of 0.13–1.42 (Table 2). Eight analysed zircon spots define concordia 206Pb/238U age of 521.50 ± 3 Ma (Figure 10b; MSWD = 0.0011), which can be further considered to either represent an early Cambrian magmatic event or might be a part of the same magmatic event as that determined from GKS-40, which might have lasted for 40 Ma. Th/U vs. U plots (Figure 9c, d) indicate that the zircon grains from both samples have a magmatic origin.
6. Discussion
6.1. Fractional crystallization and crustal contamination
Before considering any petrogenetic interpretation, it is important to evaluate the effects of metamorphism and alteration on the geochemistry of any mafic magmatic rocks. The studied samples have not undergone significant secondary alteration as inferred from their low LOI values (LOI = 0.88–2.94 wt%), absence of Ce anomalies, and lack of carbonization or silicification. In mafic rocks, LILE such as Sr, Ba and Rb are considered as mobile elements, while transition elements such as Ni, Cr and V and HFSE such as REE, Nb, Ti and Th as immobile during alteration and low-grade metamorphism [Pearce and Cann 1973, Staudigel et al. 1996]. Zr is regarded as the most immobile element under the lower grade of metamorphism [Pearce and Peate 1995, Polat et al. 2002] and is used as an alteration index during geochemical variations [Liu et al. 2012, Pearce et al. 1992]. In this study, there is a positive correlation between Zr and REE, Hf, Ta, Nb and U which indicates that they are not mobilized during alteration and metamorphism (see Table 1). Therefore, only HFSE and immobile elements are used for the studies of the petrogenesis and tectonic environment of the mafic intrusive rocks.
The low MgO (<8 wt%), Ni (36–63 ppm) and high Mg#(46.63–64.49) contents in these rocks point towards an evolved nature of the parent magma that underwent varying degrees of fractional crystallization and/or crystal accumulation. Positive correlations between Mg#and Ni and Cr indicate crystal fractionation of olivine and pyroxene, whereas negative correlations between V, TiO2 and Fe2O3 vs. Mg#suggest a minimum accumulation of amphibole and late-stage crystallization of Fe–Ti oxides in the magma (see Table 1; figure not shown). In addition, positive trends between Mg#and Al2O3 and weak Eu anomalies (Eu∕Eu∗ = 1–1.31) in the samples indicate that they record negligible plagioclase fractionation.
Crustal contamination is a common feature for mantle-derived magmas inside the magma chamber or during ascent which leads to changes in the major oxide and trace element concentrations of mafic rocks [DePaolo 1981]. Such contaminated rocks show enrichment of LREEs, LILEs, negative Nb, Ta, Ti anomalies and positive Zr-Hf anomalies in their primitive mantle normalized patterns (e.g. Rudnick and Gao [2003], Zhao and Zhou [2007]). In this study, we did not observe a strong depletion of Zr–Hf, Nb–Ta and Ti (Figure 4b), which indicates minimum crustal contamination during the evolution of the studied rocks. This observation is also supported by the lack of correlation between SiO2 and (Th/Nb)PM (Figure 5a). (La/Nb)PM vs. (Th/Nb)PM diagrams (Figure 5b) also suggests insufficient input of continental crust. Contamination of Th and U in the middle and upper crust causes their amounts to increase rapidly in the ascending magma [Taylor and McLennan 1985], but these features are not observed in the studied samples. To summarise, crustal contamination is considered to be negligible for the parental magmas of the studied samples. The slight enrichment of LREE reflects the characteristics of the source mantle prior to magma extraction. Therefore, the whole rock geochemical composition of the studied gabbros are of pure magmatic origin with little signs of contamination and can be used to determine the mantle source of the magmas as well as the processes that occurred during their emplacement.
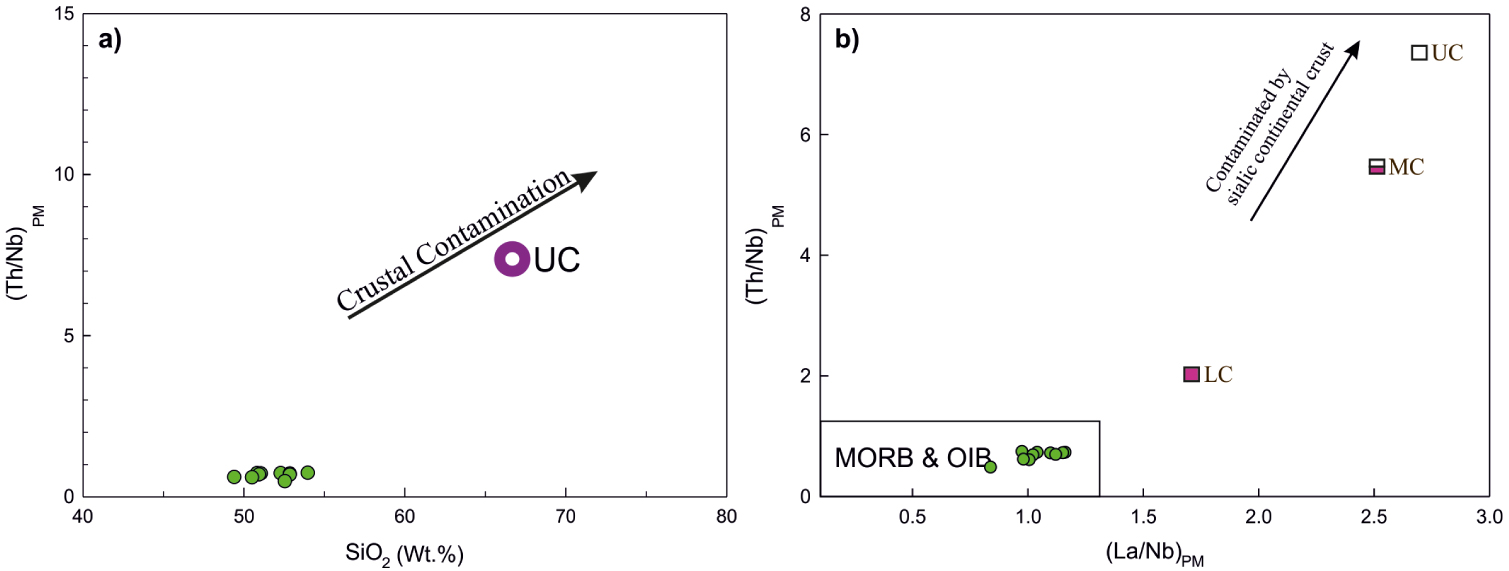
(a) SiO2 vs. (Th/Nb)PM; (b) (La/Nb)PM vs. (Th/Nb)PM (after Frey et al. [2002]) plots for testing crustal contamination of mafic intrusive rocks from the Pangin area of the Siang Window. Subscripts PM indicate ratios normalized to primitive mantle values of Sun and McDonough [1989]. UC-upper crust, MC-middle crust, and LC-lower crust values after Rudnick and Gao [2003].
6.2. Mantle source characteristics
The HFSE and LREE ratios (Nb/Ta, Zr/Hf and Nb/Yb) are important proxies for identifying different sources of the mantle for mafic magmatic rocks [Pearce and Peate 1995, Weyer et al. 2003]. In our study, the gabbros show higher LREE and HFSE enrichment and Nb/Ta (15.68–28.75) and Zr/Hf (35.47–53.17) ratios higher than the chondritic values (17.6, 36.3, respectively) suggesting their generation from an enriched mantle source [Weyer et al. 2003]. Th/Nb vs. Nb/Yb, and TiO2/Yb vs. Nb/Yb plots (Figure 6a, b) confirm the enriched mantle source signature of the studied samples. The absence of strong negative Nb–Ta and Zr–Hf anomalies in the primitive mantle normalized diagram (Figure 4b) combined with their plotting in the MORB-OIB array (Figure 6a) indicates that these rocks do not record any interaction with slab-derived material [Pearce 2008]. The samples have high La/Yb and Nb/La ratios (3.70–5.57 and 0.52–1.45) and fall in the asthenospheric mantle source field (Figure 7) suggesting that they are not contaminated by crustal rocks. The studied rocks have higher Th/Yb (0.27–1.43), Th/Ta (0.84–1.75), Ta/Yb (0.17–0.35) and Zr/Y (2.53–5.38) ratios and lower Zr/Nb (8.87–16.14) and La/Nb (0.69–0.97) ratios as compared to normal-MORB (0.04, 0.75, 0.052, 2.64, 31.75 and 1.07, respectively; after Sun and McDonough [1989]). These geochemical ratios are clear indications towards an E-MORB source.
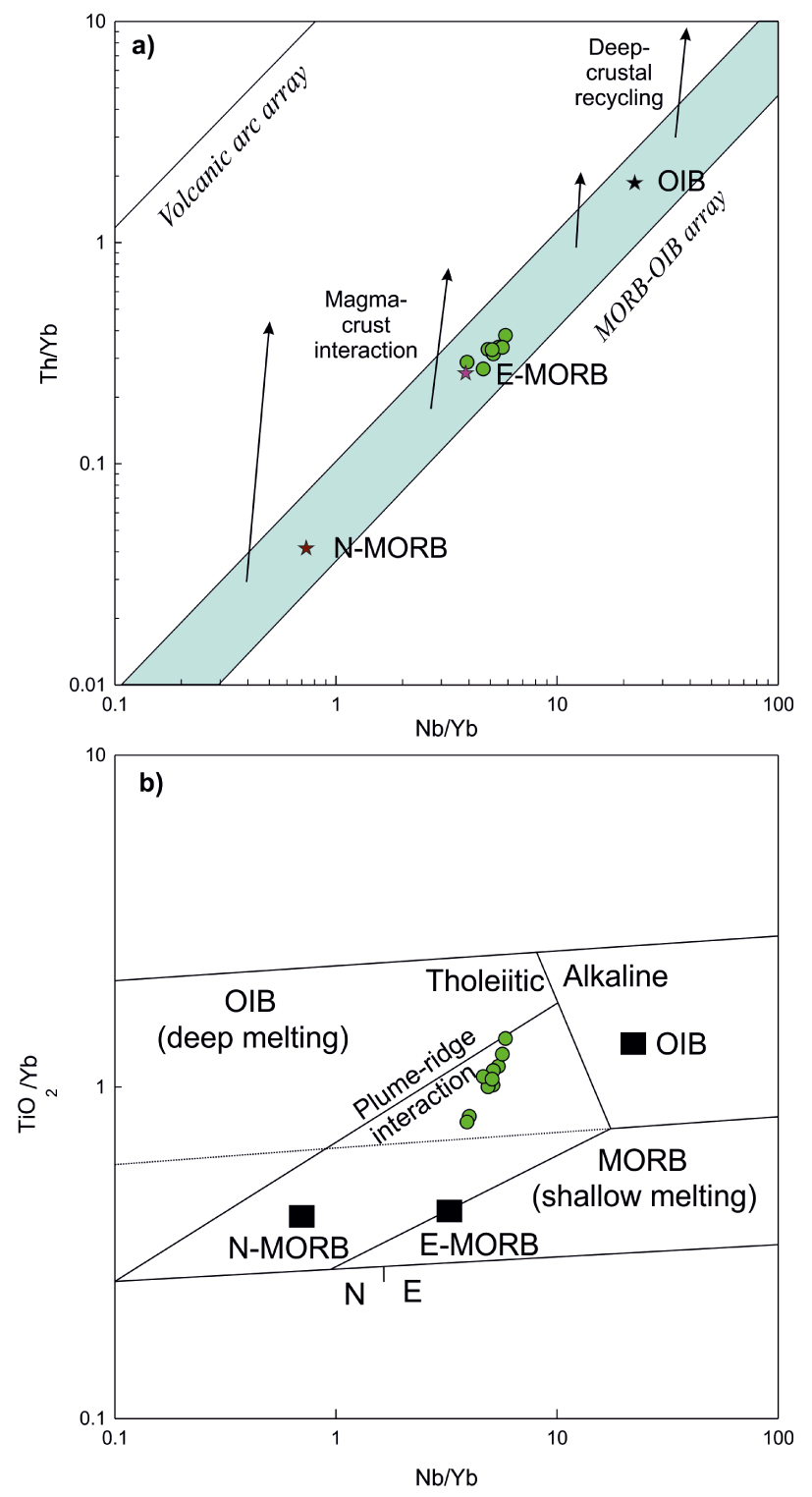
(a) Nb/Yb vs. Th/Yb plot (after Pearce [2008]); (b) Nb/Yb vs. TiO2/Yb diagram (after Pearce [2014]) for mafic intrusive rocks from the Pangin area of the Siang Window. Data for E-MORB, N-MORB, and OIB are from Sun and McDonough [1989].
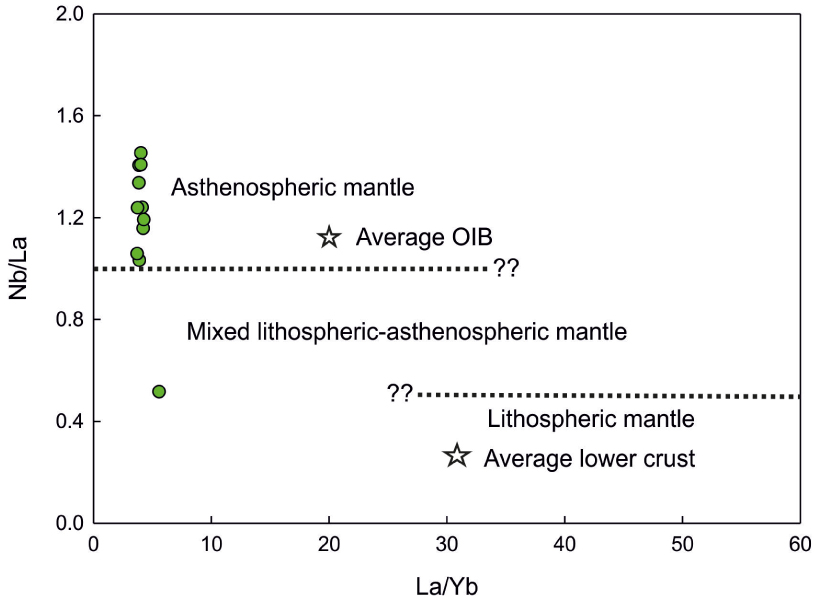
La/Yb vs. Nb/La plot to distinguish for the lithospheric and asthenospheric mantle (after Abdel-Rahman and Nassar [2004]) for mafic intrusive rocks from the Pangin area of the Siang Window.
Primitive normalized spider diagrams and chondrite normalized REE patterns of the studied samples show a pattern similar to E-MORB (Figure 4a, b). There is a slight depletion of Ti and Nb anomalies in comparison to La and Ce (Figure 4b). Their insignificant Eu anomalies indicates that plagioclases were not fractionated (Figure 4a). Zr/Ba ratio is regarded as a geochemical signature for differentiating between the asthenospheric source (Zr/Ba > 0.5) and lithospheric source (0.3–0.5) Kürkcüoglu [2010], Menzies et al. [1991]. In this study, the Zr/Ba ratio ranges from 0.32 to 1.51 and the Zr/Hf ratios vary between 35.47 and 53.17 indicating an asthenospheric source. This observation is also supported by Nb/La vs. La/Yb (Figure 7), where the studied samples fall in the asthenospheric mantle.
To understand the melting conditions of the mantle, REE compositions are treated as a geochemical signature because the REE ratios can indicate the degree of partial melting [He et al. 2010, Lassiter et al. 1995, Reichow et al. 2005]. In the spinel stability field, Sm/Yb is unfractionated while La/Yb is somewhat fractionated; for the garnet peridotite field, both Sm/Yb and La/Yb are strongly fractionated [Lai et al. 2012, Xu et al. 2005, Yaxley 2000]. The studied gabbros are marked by (La∕Y b)CN = 2.65–3.99, (Sm∕Y b)CN = 1.60–2.35 and (Gd∕Y b)CN = 1.44–2.22 (see Table 1) indicating moderate fractionation of HREE which suggest that the source magma might have both garnet and spinel peridotite components [Buslov et al. 2010, Safonova et al. 2008]. In Figure 8a (La/Sm vs. Sm/Yb plot), the studied samples fall in the garnet peridotite trend. In Sm/Yb vs. Sm plot (Figure 8b), the samples fall between the field of garnet and spinel-garnet lherzolite. Mafic lavas are considered to be derived from mantle melting at depths less than 100 km. Generally, the mantle source of this field is considered to be at a depth between 60 and 80 km but in case of the presence of a mantle plume, their depth might be 80–100 km [McKenzie and O’Nions 1991, White and McKenzie 1995]. Therefore, it is suggested that the studied gabbros of the Siang window, eastern Himalaya, were produced by 12–28% partial melting of a mantle source extending from spinel+garnet lherzolite to garnet lherzolite facies at a depth of 60–100 km.
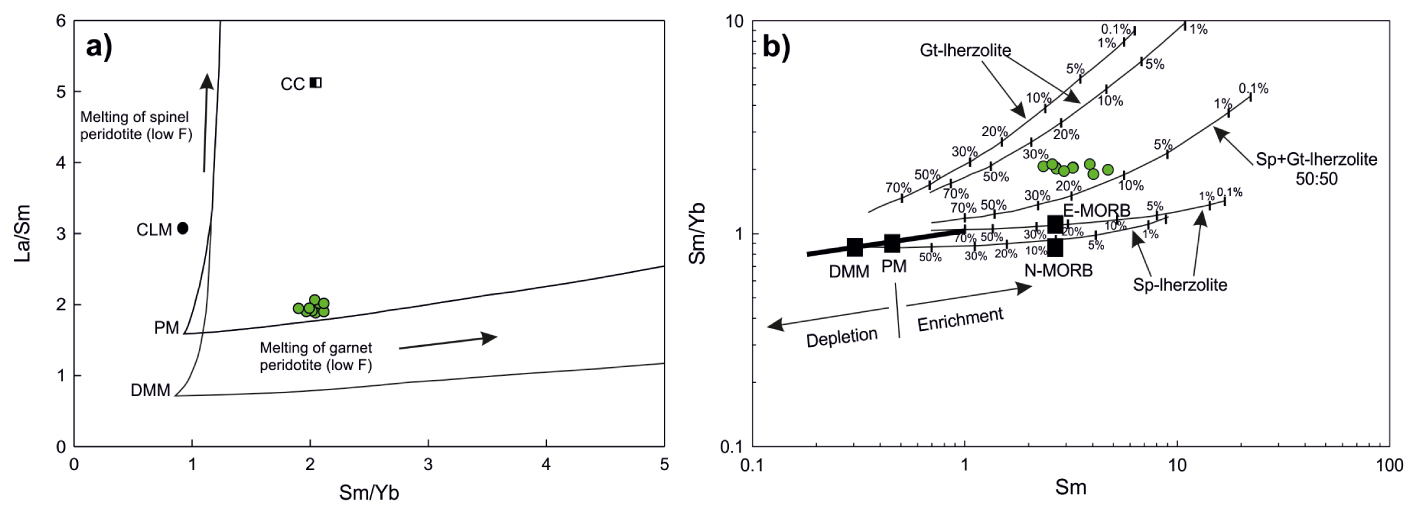
(a) Sm/Yb vs. La/Sm plot for the mafic intrusive rocks from the Pangin area of the Siang Window. Batch melting trends for garnet and spinel peridotite are after Lassiter and DePaolo [1997]. Arrows denote the effect of decreasing melt fraction (F). (b) Plots of Sm/Yb vs. Sm for the mafic intrusive rocks from the Pangin area of the Siang Window, Eastern Himalaya, Northeast India. The heavy line represents the mantle array defined using DMM [McKenzie and O’Nions 1991] and PM compositions [Sun and McDonough 1989]. N-MORB and E-MORB compositions are from Sun and McDonough [1989]. Solid curves (or lines) are the melting trends from DMM. Melting curves for spinel lherzolite (Ol53 + Opx27 + Cpx17 + Sp11) and garnet peridotite (Ol60 + Opx20 + Cpx10 + Gt10) with both DMM and PM compositions are after Aldanmaz et al. [2000]. Number marks on each curve (or line) correspond to degrees of partial melting for a given mantle source. Masquer
(a) Sm/Yb vs. La/Sm plot for the mafic intrusive rocks from the Pangin area of the Siang Window. Batch melting trends for garnet and spinel peridotite are after Lassiter and DePaolo [1997]. Arrows denote the effect of decreasing melt fraction ... Lire la suite
The origins of mafic magmatic rocks are mainly ascribed to: (1) within-plate oceanic or continental environments, (2) fast and slow-rifting MORs, (3) island arcs and (4) back-arc basins [Pearce 2008, Pearce and Cann 1973, Pearce and Norry 1979]. The studied samples consist of low to medium Ti contents (TiO2 = 0.97–1.93 wt%), Al2O3/TiO2 ranging from 7.25–14.47 and are compared to island arc basalts (15–25) and MORB (10–15), thus suggesting their formation within a plate oceanic or continental tectonic environment [Manikyamba et al. 2004, Regelous et al. 2003]. To classify basaltic magmas generated in different tectonic environments, Saccani et al. [2015] has used the Th and Nb systematics (Figure 11a). On ThN vs. NbN plot (Figure 11a), the studied samples fall near the back-arc B field which indicates that subduction-derived fluids or any other crustal components have not played any role in the formation of our rocks. This type of tectonic setting is usually found in mature intra-oceanic or intra-continental back-arcs. In the tectonic discrimination diagram of V vs. Ti (Figure 11b), the mafic intrusives are plotted in MORB and continental flood basalts (CFB) field and possess high Ti/V ratios. The Zr vs. Zr/Y diagram of Pearce and Norry [1979] helps in effectively discriminating between basalts from different tectonic settings like oceanic-island arc, mid-oceanic ridges, within-plate and back-arc, and our samples plot in the zone of within-plate basalts (WPB) and MORB (Figure 11c). In the plot of Zr vs. Ti (Figure 11d), the studied samples fall in the field of within plate basalts (WPB) and MORB, further proving their intra-continental geodynamic setting. WPBs which have higher Ti/Y and Nb/Y ratios signify an enriched mantle source relative to N-MORB and volcanic arc basalts (VABs) [Rollinson 1993]. The absence of primary hornblende in thin sections, enriched LREEs and low to moderate concentration of Ti in the samples also suggest an E-MORB source for the studied rocks. Keeping all these arguments in mind, it can be suggested that the mafic intrusives (gabbros) of the Siang window originated in a continental extension environment.
6.3. Geodynamic implications
Earlier, it has been proposed that the Abor volcanism was contemporaneous to the Indian and Eurasian plates collision event which occurred during the Early Eocene due to either adiabatic decompression following thickening of the crust [Sengupta et al. 1996] or a thermal anomaly caused by slab break-off following the collision [Acharyya 2007]. Later, K/Ar dating for the Abor volcanics on different basalt samples yielded varied ages of 319 ± 15 Ma (Late Carboniferous), 87.2 ±1.3 (Late Cretaceous) and 24.9 ± 0.4 Ma (Early Tertiary) [Liebke et al. 2011]. Ali et al. [2012] have interpreted the steeply dipping magnetization in the basalts to be primary in nature and have given them a Permian age. They have deduced that the rocks formed due to eruptions associated with rifting of blocks of the Cimmerian continent situated in the northern margin of the Gondwana supercontinent. More recently, zircon U–Pb geochronological studies of the Abor volcanics suggest that they were emplaced due to the outburst of the Kerguelen plume at the early stages (∼132 Ma) of the eastern Gondwana breakup [Singh et al. 2019].
In light of the recent findings, our zircon U–Pb data for the mafic intrusive rocks from the Pangin area of the Siang window yield an age ranging from 521 to 567 Ma, i.e. Late Neoproterozoic to Early Cambrian which is the oldest magmatic event reported so far from the Siang window. This age coincides with the age of the closing of the Mozambique Ocean during the Kuunga orogeny which is the eastern part of the Pan-African orogenic belt [Meert et al. 2003, Meert and Lieberman 2008]. Our obtained ages are totally different from the previous studies; therefore, their source might also be different from the Abor Volcanics.
It has been reported that the Himalayan litho-tectonic units were part of the north Indian passive margin from the Precambrian to the Cretaceous period [Brookfield 1993]. This observation is inconsistent with the presence of many early Paleozoic granites in the Himalaya [Nadimi 2007, Ramezani and Tucker 2003]. It has been also reported that from 500 to 475 Ma, the Kathmandu region of the Higher Himalayan crystalline underwent deformation and that the Indian proto-Tethyan margin was strongly affected during the Cenozoic Himalayan formation [Cawood et al. 2007, Gehrels et al. 2006]. During the assembly of the Gondwana supercontinent (570–510 Ma), the initiation of subduction along the Gondwana proto-Tethyan and Gondwana proto-Pacific margins occurred in the last stage of subduction [Cawood and Buchan 2007, Cawood et al. 2007]. From this observation, Cawood et al. [2007] interpreted the early Paleozoic magmatism found in the Himalaya as the result of southward subduction of the proto-Tethyan oceanic lithosphere beneath the northern Indian continent. Similar Late Neoproterozoic to Early Cambrian magmatisms are also reported from the northern Gondwana margin of Australia, Iran, Arabia and Turkey, which is shown in Figure 12 [Gürsu and Göncüoglu 2005, Gürsu et al. 2015, Hassanzadeh et al. 2008, Horton et al. 2008, Mahmoud et al. 2011, Saki et al. 2010, Ramezani and Tucker 2003].
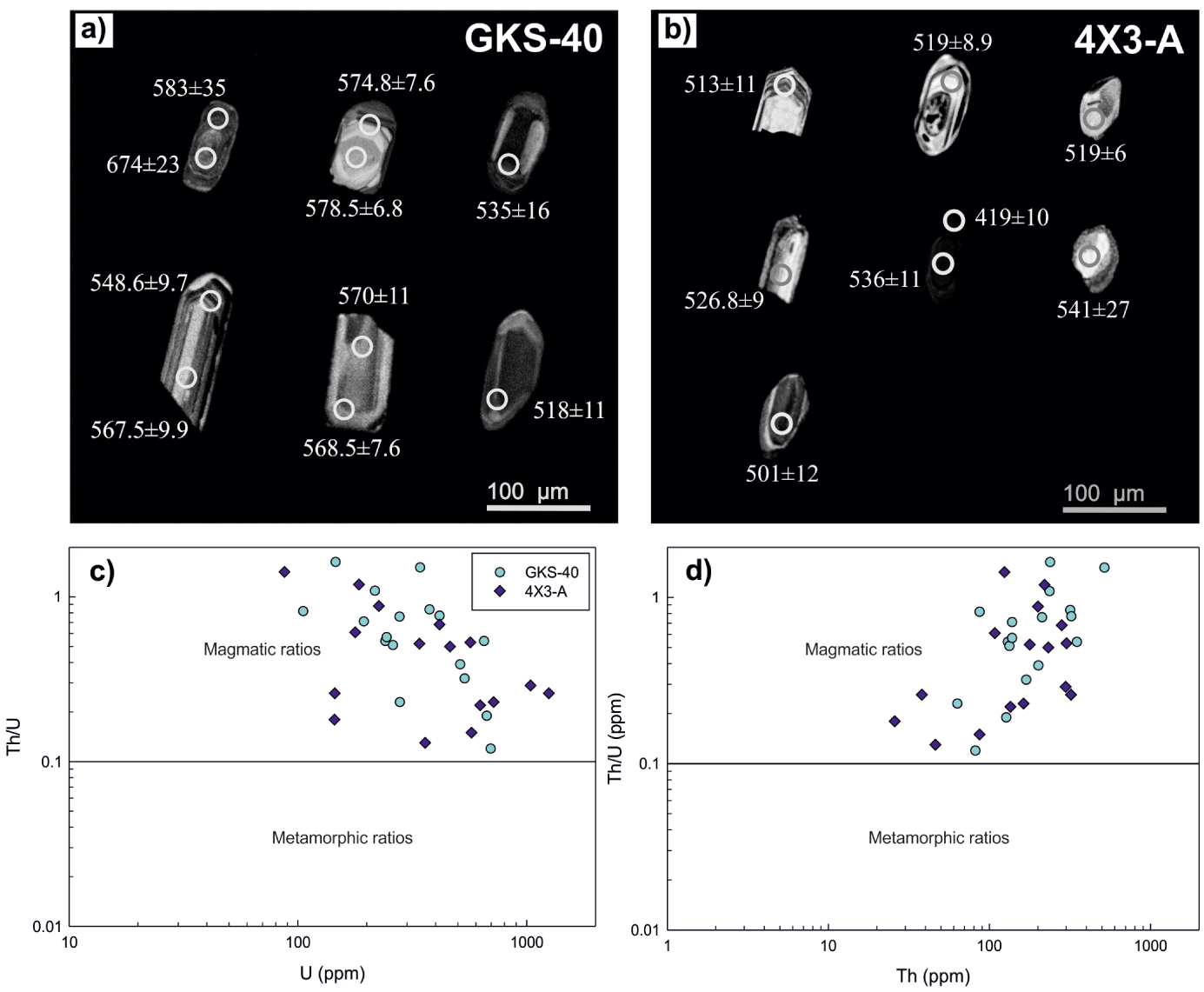
Cathodoluminescence (CL) images (a, b) of zircon U–Pb dating for the mafic intrusive rocks from the Pangin area of Siang window. U vs. Th/U and Th vs. Th/U plots (c, d) (after Hoskin and Black [2000]).
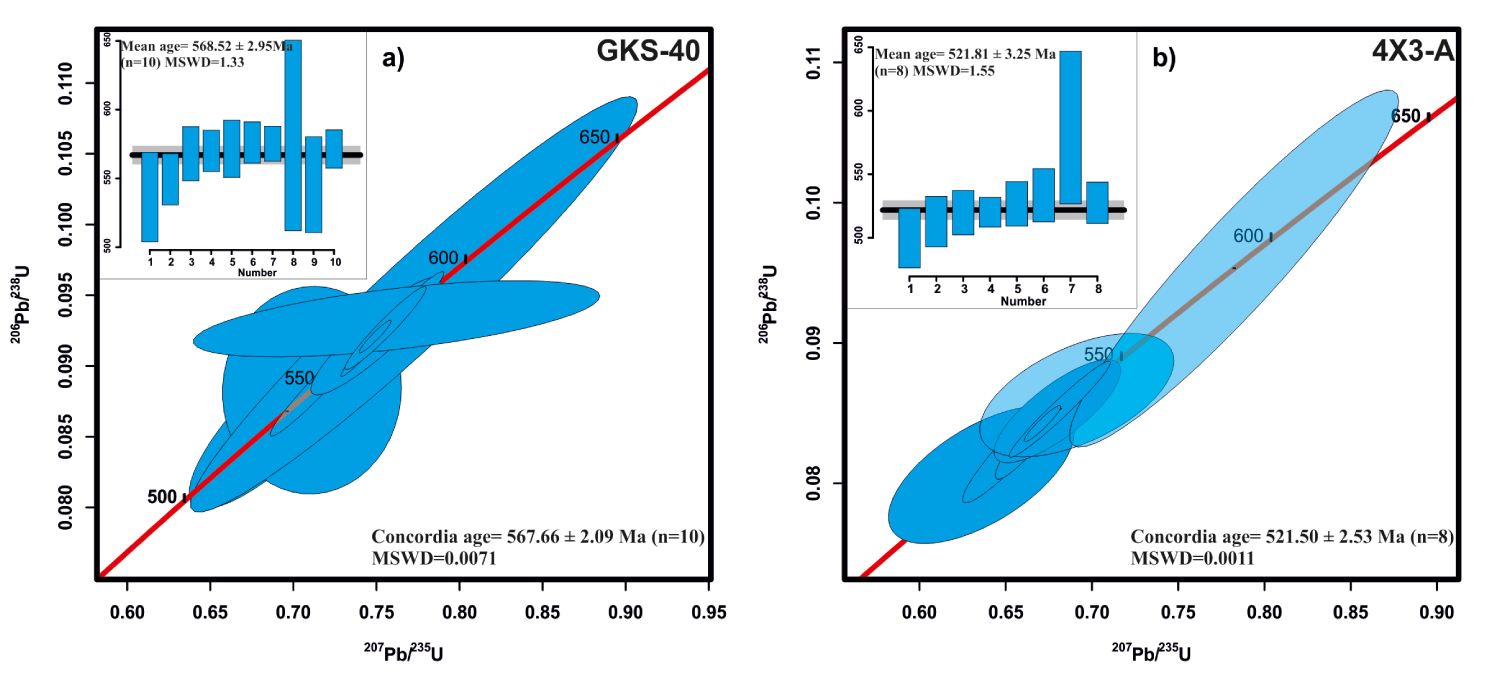
Concordia plots of zircon U–Pb dating for the mafic intrusive rocks: (a) Sample GKS 40, (b) Sample 4X3-A from the Pangin area of the Siang Window; the upper insert shows the weighted mean age.
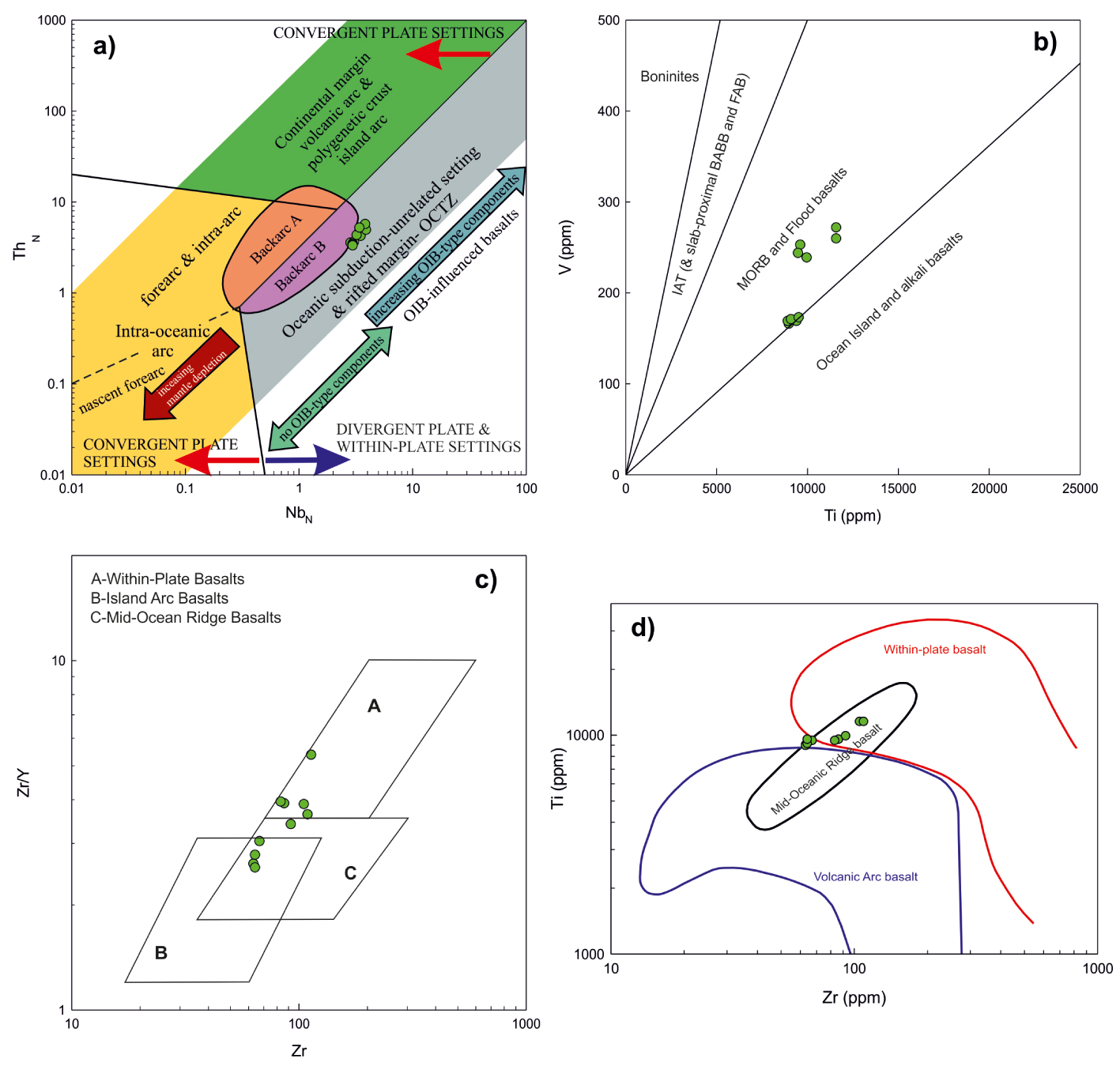
Tectonic discrimination diagrams for mafic intrusive rocks from the Pangin area of the Siang window (a) NbN vs. ThN (after Saccani et al. [2015]); N – normalizing values are the N-MORB composition of Sun and McDonough [1989]; (b) V vs. Ti plot (after Shervais et al. [1982]) showing that the studied samples fall in the field of MORB and flood basalts; (c) Zr/Y vs. Zr diagram (after Pearce and Norry [1979]); (d) Ti vs. Zr (after Pearce et al. [1981]).
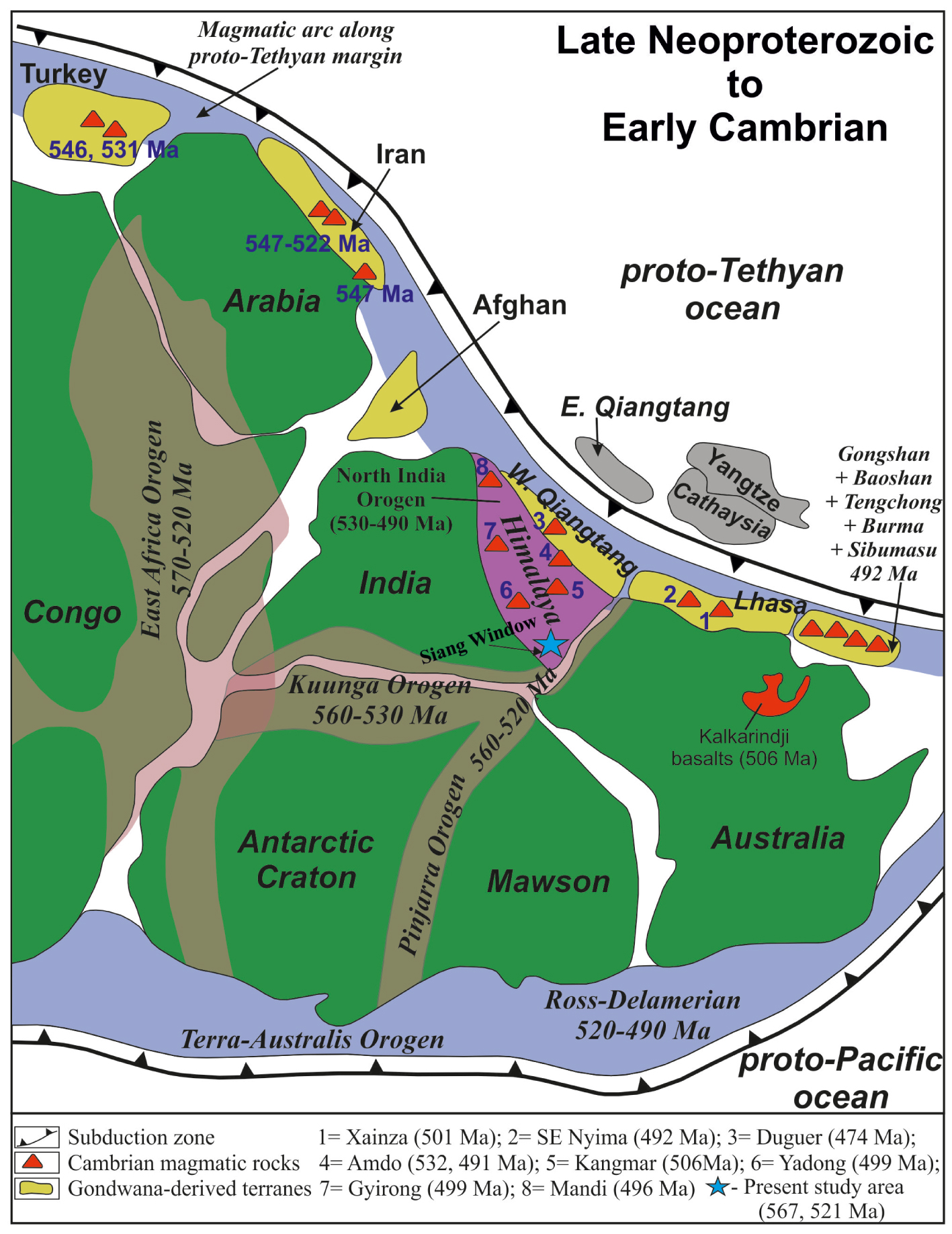
Reconstruction of the northern margin of Gondwana (modified from Zhu et al. [2012]) showing the location of the proposed late Neoproterozoic to early Cambrian extensional tectonic environment and early Paleozoic Andean-type magmatic arc from the Indian proto-Tethyan margin to the Australian proto-Tethyan margin.
A late Pan-African granitic activity (500 ± 50 Ma) and a transgressive Ordovician sedimentary succession characterize a large area of the Indian part of the Gondwana supercontinent [Le Fort et al. 1994]. Gaetani and Garzanti [1991] proposed that the early Paleozoic granitoids might have been associated with the formation of the Gondwana supercontinent. Based on geochemical characteristics, and ages (absence of Sr and Ba enrichment; the short-lasting magmatism from 550 to 470 Ma; in the absence of Cambro-Ordovician metamorphic and deformation records) of the Mandi basalts in northwest Himalaya, it has been proposed that the Mandi magmatism was emplaced in an extensional tectonic setting rather than a subduction to collision-related setting [Debon et al. 1986, Le Fort et al. 1986, Miller et al. 2001]. Further, Miller et al. [2001] have also proposed that the Mandi basalts were formed due to the asthenospheric upwelling and passive crustal extension possibly in a rift setting that separated the proto-Indian margin and the cratonic Asian fragments such as the South China block [Dalziel et al. 1994]. They also correlate the early Paleozoic magmatic activities from the northwest Himalaya with the late extensional stage of the long-lasting Pan-African orogenic events which ended with the formation of the Gondwana supercontinent [Girard and Bussy 1999]. The early Palaeozoic granites in the Northwest Himalaya post-date the collision and other major thermal events during the Pan-African orogeny. Palaeogeographic reconstructions suggest that only the southern parts of the Indian plate were affected by the main Pan-African event (e.g. Stern et al. [1994]). Therefore, the faults bounding the vast infra-Cambrian to mid-Cambrian evaporite basins of central Iran could have been related to a late Pan-African Basin-and-Range type rift cluster that may have affected much of the Gondwana margin between Arabia and northern India [Sengör and Natal’in 1996]. Since the magmatic arc is absent in the present study area, we have ruled out the formation of the studied mafic intrusive rocks in the BABB setting as this is geologically invalid, but we have compared them to the late extensional stage of the long-lasting Pan-African orogenic cycle in the northern Indian Gondwana margin, which ended with the assembly of the Gondwana supercontinent. Compared with the previously reported emplacement of numerous Late Neoproterozoic to Early Cambrian granitoids and Early Paleozoic mafic rocks of northwest Himalaya, our data also represent an extensional environment of the northern Gondwana margin related to the late extensional stage of the Pan-African orogenic cycle with the formation of the Gondwana supercontinent. A Late Neoproterozoic-Early Cambrian reconstruction depicting the location of peri-Gondwana terranes with the present investigated mafic intrusive rocks along the northern margin of the Gondwana supercontinent is shown in Figure 12 (modified after Zhu et al. [2012]).
7. Conclusions
Our new U–Pb zircon ages and whole-rock geochemistry presented in this study support the following conclusions:
- (1) Mafic intrusive rocks from the Pangin area of the Siang window, Eastern Himalaya, northeast India are gabbroic in composition with a sub-alkaline tholeiitic affinity. Their geochemical signatures are similar to E-MORB, and the parental magma might have been generated by medium to high degrees of partial melting (∼12–28%) of an enriched mantle spinel+garnet peridotite source.
- (2) The investigated two gabbro samples yield U–Pb zircon ages of 568 ± 2 Ma and 521.5 ± 2.5 Ma, respectively, indicating Late Neoproterozoic and Early Cambrian magmatic activities in the Siang window.
- (3) These mafic intrusive rocks are geochemically distinct from the earlier reported mafic and felsic volcanics of the Siang window; rather, they may be derived from the melts generated in an extensional tectonic setting.
- (4) Thus, our new geochemical and geochronological data suggest that the studied mafic intrusive rocks of the Siang window were generated during the Late Neoproterozoic to Early Cambrian extensional tectonic environment, similar to those reported from Northwest Himalaya due to the long-lasting Pan-African orogenic cycle which ended with the assembly of the Gondwana supercontinent.
Acknowledgements
The authors are grateful to the Director, Wadia Institute of Himalayan Geology (WIHG), Dehradun for encouragement and permission to publish the present manuscript. We are also thankful to the Lab-in-Charges of XRF, ICPMS, SEM and LA-MC-ICPMS facilities, WIHG for analytical support. Special thanks to Saurabh Singhal, WIHG, for helping with LA-MC-ICPMS U–Pb isotopic analyses. The manuscript has benefited with insightful suggestions from S. Khogenkumar, NCPOR, Goa. This work is part of the ongoing Ph.D. thesis of the first author at BHU, Varanasi and WIHG, Dehradun. We thank Editors-in-Chief, Emeritus Professor Ghislain de Marsily and Professor François Chabaux for their constructive comments. Professor Michel Faure and an anonymous reviewer are thanked for their fruitful suggestions and thorough reviews which helped to improve this study.