1. Introduction
The Dinarides stand for a 700 km long Alpine orogen consisted of high-karst mountains with a NW–SE extension, which connect the Southern Calcareous Alps (NW) and the Albanides (SE) [Dimitrijević 1982; Pamić et al. 1998; Vlahović et al. 2005, 2012; Bortolotti et al. 2013, Figure 1a]. In the Triassic, the area of present-day Dinarides was located south of the northern margins of the subducting Paleotethyan lithosphere [Stampfli and Borel 2002, 2003; Scotese 2002]. During the Middle Triassic, the area of the Dinarides was affected by prominent tectonic processes which include wrench faulting, block tectonics and rifting [Schmid et al. 2008]. Repetitive uplifts and subsidence gave rise to the formation of deep marine environments marked by significant magmatic activity [e.g. Pamić and Balen 2005]. The magmatism had its peak during the Ladinian [e.g. Pamić 1984; Knežević et al. 1998; Trubelja et al. 2004; Pamić and Balen 2005; Smirčić et al. 2018, 2020] and is related to the formation of Tethyan Mesozoic Adriatic-Dinaridic Carbonate Platform(s) [e.g. Castellarin et al. 1988; Philip et al. 1995; Vlahović et al. 2005, 2012; Robertson 2007].
The Dinaridic mountain chain is made of thick and tectonized Carboniferous to Quaternary sedimentary successions [Pamić et al. 1998]. A plethora of Alpine tectonic events controlled the evolution of the Dinarides from the Late Permian to Oligocene; however, the most prominent event was the tectonic uplift and formation of an imbricated nappe system during the Late Eocene to Oligocene, which ultimately brought about a fold-and-thrust belt of the Dinarides [Pamić et al. 1998; Balling et al. 2021]. The Dinarides are consisted of the two parallel belts (Figure 1a): External Dinaridic platform and Internal Dinarides. The former includes the coastal mountain chains which stretch along the shores of the Adriatic, while the latter are found to the northeast extending parallelly to the former. External Dinarides are largely made of Jurassic to Cretaceous carbonate successions, which constitute the Adriatic Carbonate Platform [AdCP; Vlahović et al. 2005], while Internal Dinarides are consisted of deep-water sedimentary successions and ophiolite mélange obducted along the margins of Adria Plate [Chiari et al. 2011; Dimitrijević and Dimitrijević 1973; Pamić et al. 1998; Šegvić et al. 2014, 2019, 2020].

(a1) Geotectonic sketch map of the major tectonic units and (a2) schematic cross-section accros the Dinarides [simplified after Schmid et al. 2008, 2019]. Legend: External Dinarides: (1) Adriatic Plate, (2) Dalmatian Zone, (3) High Karst; Internal Dinarides: (4) Pre-Karst and Bosnian Flysch, (5) Drina-Ivanjica; (6) Meliata, Darnó-Sźarvaskö, Dinaric, Western Vardar, Mirdita; (7) Bükk, Jadar, Kopaonik. ZMTDZ—Zagorje-Mid-Transdanubian Zone, SKF—Split-Karlovac fault. The occurrences of magmatic rocks: (1) Fužinski Benkovac, (2) Donje Pazarište, (3) Senjska Draga, (4) vicinity of Drniš, (5) vicinity of Sinj. (b1) Simplified geological map of a wider research area and (b2) detailed cross-section [modified after Grimani et al. 1972; Kuljak 2004]. Legend: (1) Quaternary sedimentary rocks; (2) Paleogene limestone conglomerates and breccias; (3) Early Cretaceous limestones; (4) Jurassic limestones and dolomites; (5) Triassic limestones, dolomites, clastic and volcanoclastic rocks; (6) subvolcanic rocks; (7) reverse or thrust faults; (8) normal faults; (9) normal geological line; (10) discordance line, tectonic-erosion discordance; (11) location of investigated area. (c) Field occurrence of the dolerite rocks in the study area. Masquer
(a1) Geotectonic sketch map of the major tectonic units and (a2) schematic cross-section accros the Dinarides [simplified after Schmid et al. 2008, 2019]. Legend: External Dinarides: (1) Adriatic Plate, (2) Dalmatian Zone, (3) High Karst; Internal Dinarides: (4) Pre-Karst ... Lire la suite
The occurrences of magmatic rocks in External Dinarides, i.e. the area of the Adriatic Carbonate Platform, are rarely reported, and if found, are small in surface cropping out at few localities solely (Senjska Draga, Donje Pazarište, vicinity of Sinj, Drniš and Knin, Fužinski Benkovac; Figures 1a and b). These basaltic, andesitic, dacitic, doleritic and gabbroic rocks may be found on some of the remote Adriatic islands such as Jabuka, Brusnik and Vis (Figure 1a); however, their origin has heretofore not been unequivocally defined especially with regards to geotectonic movements which caused the magmatism parental to those rocks [e.g. Barić et al. 1968; Barić 1969; Golub and Vragović 1975; Lugović and Majer 1983; Pamić 1984; Trubelja et al. 2004; Belak et al. 2005; Pamić and Balen 2005; Garašićet al. 2005, 2006; De Min et al. 2009; Palinkaš et al. 2010; Smirčić 2017]. Triassic effusive and associated volcanoclastic rocks are allochthonous and encountered in the fault zones along the rims of the Adriatic Carbonate Platform in contact with Anisian to Carnian sedimentary successions while intrusive rocks may crosscut Permian to Lower Triassic strata [Pamić 1984; Pamić and Balen 2005].
This study has a focus set on the biggest Triassic igneous subvolcanic body which crops out in the heart of External Dinarides in Croatia (Figure 1a–c). The body represents a unique manifestation of hypabyssal dolerite within the Adriatic Carbonate Platform. Its origin is related to the widespread magmatism having taken place along the rims of the AdCP which is documented by numerous occurrences of gabbroic rocks below Adriatic Sea [Mancinelli et al. 2022]. Assessing complex geodynamic processes and magmatic activity along AdCP continental rims the aim of this research is to advance our understanding on the extensional magmatism issued from subduction-modified mantle domains. This study brings new petrographic data, phase and rock geochemistry, as well Nd isotope systematics of investigated dolerite. From a mineralogical perspective this research offers an extensive characterization of the rare dolerite occurrence, while from the petrological point of view defines dolerite’s geodynamic affinity, petrogenesis, as well as the intensity of plausible contamination and alteration processes. Petrogenetic inferences will be correlated with existing data collected from similar Triassic rocks cropping out throughout External Dinarides [Belak et al. 2005; Garašićet al. 2005, 2006; De Min et al. 2009; Palinkaš et al. 2010], Middle Triassic volcanic series from the Zagorje-Mid-Transdanubian Zone [Slovenec et al. 2020; Slovenec and Šegvić 2021, Figure 1a] as well as Triassic volcanic rock from the Albanide-Hellenide belt [Pe-Piper and Panagos 1989; Pe-Piper and Mavronichi 1990; Capedri et al. 1997; Pe-Piper 1998; Bortolotti et al. 2004, 2006; Monjoie et al. 2008; Chiari et al. 2012]. This will ultimately shed light on the influence of pre-Triassic subduction events on the composition of lithospheric mantle below the AdCP and add to the understanding of Mesozoic geodynamics of the central Mediterranean prior to the final disintegration of Pangea.
2. Geology of the study area
The location of the study area is in the south-western part of External Dinarides and belongs to the High Karst Unit [after Schmid et al. 2008; Figures 1a1 and a2]. The broader investigation area is geologically complex and according to Grimani et al. [1975] constitutes the Poštak-Plavno-Pađene tectonic unit, which is largely made of Triassic sedimentary series. Their lower bedding plane is made of Lower Triassic clastic and carbonate rocks represented by “Seissian beds” (shaley sandstone, oolithic/clayey limestone) and “Campilian beds” (platy limestone, marl, and dolomite). These rocks are overlain by Middle Triassic limestone and dolomite alternated by clastic and volcanoclastic rocks. The upper bedding plane of Triassic rocks is consisted of Upper Triassic dolomite transgressively overlain by Jurassic sediments (limestone and dolomite) of the Adriatic Carbonate Platform. Upper Cretaceous limestone is a next lithological member in this sequence found in a transgressive or tectonic contact with older rocks. Scarce occurrences of Paleogene carbonate-rich conglomerate and breccia are also documented transgressively overlying Upper Cretaceous rock series (Figure 1b).
Studied subvolcanic rocks crop out for about 300 m along the road at the southern entrance to the city of Knin (Northern Dalmatia, Croatia) (Figures 1a1, b1). These rocks are tectonically overlain by Late Cretaceous layered to blocky limestone and Early Triassic platy limestone (“Campilian beds”), while northwards the studied rocks are covered by Quaternary deposits (Figures 1b1, b2). The dolerite is found in the zone of the Split-Karlovac paleotransform fault [Chorowicz 1975, 1977, Figure 1a1], along which Upper Permian evaporites break through [Bahun 1985; Figures 1b1, b2]. The studied dolerite, along with eruptive rocks from the region, was researched by Barić [1969], Grimani et al. [1975], and Kuljak [2004]; however, a detailed mineralogical, petrological and geochemical characterization is lacking. The age of magmatic activity parental to this magmatic occurrence has not been unequivocally determined as well. According to Grimani et al. [1975] it could be Pre-Triassic, likely corresponding to the Late Permian based off the age of the inclusions and identical heavy mineral fraction found in Upper Permian clastic rocks of the broader region [Ivanović et al. 1978; Šušnjara et al. 1992]. Middle Triassic (Ladinian) age cannot be excluded either considering a positive correlation with similar rocks at the neighboring mountain of Svilaja (Figure 1a) which are in a direct contact with Ladinian sediments [Ščavničar et al. 1984]. Bearing in mind such geological context and lack of datable igneous phases in dolerite this contribution favors its Middle Triassic age which is inferred for similar intrusive rocks at the Croatian Middle Adriatic islands [De Min et al. 2009]. Albeit cropping out at a relatively small surface, studied dolerite represents the most important primary occurrence of Middle Triassic(?) coarse grained mafic rocks in the central part of External Dinarides.
3. Materials and methods
3.1. Materials
Studied dolerite may be described as massive, brownish to greyish, heavily altered rock with an irregular to platy habit and visible ferromagnesian phases up to 4 mm in size (Figure 1c). Going toward the rims of the dolerite body the size of mineral grains abates because of rapid cooling of this hypabyssal, plausibly dyke-like type of rock. A set of 14 dolerite samples was selected for mineralogical, chemical and isotopic (143Nd/144Nd) analyses. All samples were firstly investigated with a petrographic microscope to define the rock’s texture and modal mineralogy.
3.2. Analytical techniques
To determine mineral phase compositions of representative sample, we carried out the electron microprobe analyses and elemental X-ray study using a JEOL JXA 8200 Superprobe with a wavelength/energy dispersive combined microanalyzer installed at the University of Geneva, Switzerland. Operating parameters included an accelerating voltage of 20 kV, a 20 nA beam current, and a beam size of ∼1 μm (for feldspars 10 μm). Counting times of 20 s on peak and 10 s on background on both sides of the peak were used for all elements. Limits of detection (LOD) were calculated as the minimum concentration required to produce count rates three times higher than the square root of the background (3 s; 99 wt% degree of confidence at the lowest detection limit). Raw data were corrected for matrix effects using the PAP algorithm implemented by JEOL [Pouchou and Pichoir 1985]. Natural minerals, oxides (corundum, spinel, hematite, and rutile), and silicates (albite, orthoclase, anorthite, and wollastonite) were used for calibration. Mineral formulas were calculated using a software package MINPET written by Linda R. Richard (Gatineau QC, Canada).
Bulk-rock powders for chemical analyses of five samples were analyzed by ICP-OES for major elements, and ICP-MS for trace elements at Bureau Veritas Laboratories (Vancouver BC, Canada). International mafic rocks were used as standards. Major element and trace element concentrations were measured with accuracy and precision better than ±1% and ±5%, respectively. It is 3σ at 10 times detection limit.
Nd isotopic compositions of two bulk rock samples were measured at the Noble Gas Laboratory Pacific Centre for Isotopic and Geochemical Research, University of British Columbia, Vancouver, Canada, following the procedure described in Weis et al. [2006]. Collector cup bias was monitored by frequent measurement of the La Jolla Nd standard which yielded 143Nd/144Nd 0.511853 ± 11 (n = 6; fractionation corrected to 146Nd/144Nd = 0.7219). Python™ programing language (numpy package) was used to calculate the Monte Carlo propagation error through 10,000 iterations for 147Sm/144Nd, 143Nd/144Nd(t) ratios.
X-ray diffraction (XRD) was carried out on a set of four representative samples. For that purpose, the material was firstly gently crushed and powdered in an agate mortar and was thereupon placed in the sample holder. The material was analyzed with a Bruker D8-Advanced diffractometer installed at Texas Tech University (Lubbock TX, USA) and run using a step scan in the Bragg–Brentano geometry with CuKα radiation. The measurement settings were 45 kV and 40 mA with sample mounts scanned from 3 to 70 °2𝜃. Measurements were completed under air-dried conditions and at a counting time of 2.5 s per 0.02 °2𝜃. Lastly, the peaks of XRD patterns were interpreted and compared with the whole-rock geochemistry generated from ICP-MS to ensure geological fluidity. The Bruker EVA diffraction suite was used to analyze diffraction data.
4. Results
4.1. Petrography and mineral chemistry
Investigated subvolcanic rocks are medium to coarse grained and show nicely preserved igneous fabrics characterized by intergranular holocrystalline texture and homogenous structure (Figure 2) which classifies them as dolerite. The crystallization sequence includes: (a) sericitized and completely albitized subhedral to euhedral plagioclase (An3.2–8.6Ab89.8–95.7Or0.4–4.1; Figures 2a–c, 3a; up to 1.2 mm in size) and less common alkali feldspar (An0.2–1.0Ab1.9–7.6Or92.0–97.8; up to 1.0 mm in size), (b) subhedral diopside to augite (Wo44.5–46.6 En37.1–45.3Fs8.3–44.5; Figure 3b), (c) brown subhedral prismatic, weakly pleochroitic, igneous Ca-amphibole (tschermakite to pargasite; up to 4 mm in size; Figures 2b–d, 3c–d), (d) Fe–Ti oxide (magnetite?, titanite), and (e) opaque hematite/limonite. Apatite has been documented as a common accessory phase. While plagioclase has been thoroughly albitized, this process affected alkali feldspar only marginally (Figure 2e). Clinopyroxene is normally zoned and features a decrease in Mg#, AlVI/AlIV, Ca and Cr coupled with an increase in Ti towards the rims of the grains (Table 1). The Mg#is relatively high (75.6–87.2), while TiO2 and Al2O3 abundances vary considerably (0.50–2.45 wt% and 0.76–6.56 wt%, respectively). Giret et al. [1980] has successfully utilized a A(Na+K) + B(Ca+Na) vs. TSi ratio to discern between igneous and metamorphic amphibole; accordingly, analyzed amphibole is largely metamorphic with only a fraction of it considered as magmatic (Figure 3d). The latter is marked by lower Mg#(<57) and MgO concentrations (<11 wt%) on the one hand and higher Al2O3 (11.21–11.42 wt%) and FeO values (>16 wt%) on the other hand compared to the composition of the former (Table 1). Comparing Mg#values of primary pargasite and tschermakite with those of primary clinopyroxene a strong correspondence may be inferred (Table 1) which reflects their roughly coeval magmatic crystallization [Singh et al. 2016].

Microphotographs of thin sections of coarse-grained dolerite from the Konj Hill (a,b) sample KN-1, N+, (c,d) sample KN-4, N+ and back-scattered electron image of coarse-grained dolerite (e) sample KN-1. The numbers correspond to the microprobe spot presented in the Tables 1–2. Mineral abbrevations after Whitney and Evans [2010]: Ab—albite, Am—amphibole, Bt—biotite, Cal—calcite, Chl—chlorite, Cpx—clinopyroxene, Kfs—alkali feldspar, Mag—magnetite, Pl—plagioclase, Prh—prehnite, Pmp—pumpellyite.
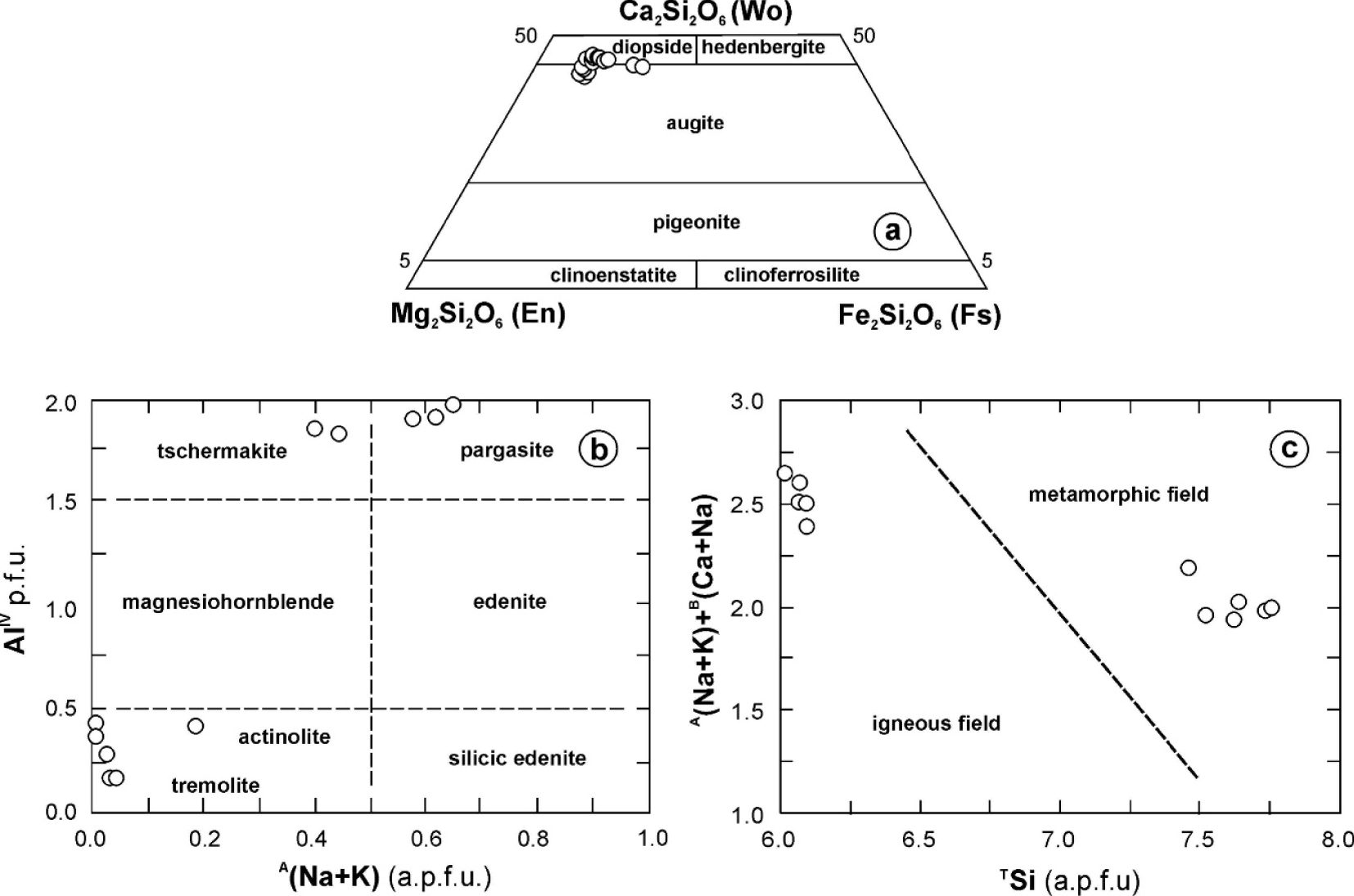
Classification diagrams for (a) pyroxene [En–Wo–Fs (Mg2Si2O6–Ca2Si2O6–Fe2Si2O6) plot Morimoto 1988], (b) calcium amphibole [AlIV–A(Na+K) plot adapted after Leake et al. 1997; Hawthorne et al. 2012] and (c) A(Na+K) + B(Ca+Na)–TSi discriminant diagram for amphibole [Giret et al. 1980].
Representative chemical compositions and calculated mineral formulae of clinopyroxene and amhibole from the mafic subvolcanic rocks (dolerites) from the Konj Hill
Mineral | Clinopyroxene | Amphibole | ||||||||||||||
---|---|---|---|---|---|---|---|---|---|---|---|---|---|---|---|---|
Sample | KN-1 | KN-1 | KN-1 | KN-1 | KN-1 | KN-1 | KN-3 | KN-3 | KN-1 | KN-1 | KN-1 | KN-1 | KN-1 | KN-1 | KN-1 | |
Anal. No. | 2;c | 3;r | 5;r | 15;c | 16;r | 40 | 49;r | 53;c | 38 | 41 | 42 | 43 | 44 | 45 | 54 | |
Min. type | di | di | di | di | di | aug | di | aug | act/tr | prg | ts | act/tr | act/tr | act/tr | prg | |
SiO2 | 51.28 | 50.41 | 50.58 | 50.85 | 49.94 | 46.53 | 51.20 | 52.67 | 53.20 | 40.56 | 40.56 | 54.97 | 54.91 | 54.50 | 39.22 | |
TiO2 | 0.68 | 1.01 | 0.99 | 1.04 | 1.13 | 2.45 | 0.72 | 0.50 | 0.15 | 3.89 | 2.57 | 0.36 | 0.26 | 0.25 | 3.87 | |
Al2O3 | 3.36 | 4.24 | 3.88 | 4.32 | 4.67 | 6.56 | 3.31 | 1.76 | 2.42 | 11.42 | 11.21 | 1.15 | 1.06 | 1.05 | 11.40 | |
Cr2O3 | 0.41 | 0.32 | 0.60 | 0.44 | 0.19 | 0.05 | 0.56 | 0.33 | 0.00 | 0.00 | 0.00 | 0.02 | 0.00 | 0.03 | 0.04 | |
FeO | 5.67 | 6.44 | 5.61 | 5.82 | 7.04 | 10.81 | 5.44 | 5.90 | 11.72 | 16.45 | 20.78 | 11.59 | 12.01 | 13.43 | 18.20 | |
MnO | 0.15 | 0.15 | 0.11 | 0.13 | 0.17 | 0.27 | 0.15 | 0.18 | 0.26 | 0.21 | 0.36 | 0.16 | 0.16 | 0.27 | 0.19 | |
MgO | 15.73 | 15.08 | 15.10 | 15.65 | 14.68 | 12.50 | 15.82 | 16.75 | 16.43 | 10.64 | 8.39 | 17.20 | 17.09 | 16.20 | 9.57 | |
CaO | 22.85 | 22.19 | 22.25 | 21.97 | 22.02 | 20.84 | 22.21 | 21.71 | 11.25 | 11.19 | 10.03 | 10.80 | 10.38 | 9.97 | 10.98 | |
Na2O | 0.18 | 0.22 | 0.21 | 0.20 | 0.18 | 0.29 | 0.20 | 0.13 | 0.73 | 2.07 | 1.89 | 1.38 | 1.43 | 1.57 | 2.12 | |
K2O | 0.01 | 0.00 | 0.00 | 0.00 | 0.00 | 0.02 | 0.01 | 0.01 | 0.05 | 1.17 | 1.13 | 0.15 | 0.20 | 0.18 | 1.17 | |
H2O | - | - | - | - | - | - | - | - | 2.06 | 1.98 | 1.93 | 2.09 | 2.09 | 2.07 | 1.93 | |
Total | 100.32 | 100.04 | 99.32 | 100.51 | 99.93 | 100.33 | 99.61 | 99.94 | 98.27 | 99.56 | 98.85 | 99.87 | 99.59 | 99.51 | 98.68 | |
Si | 1.878 | 1.857 | 1.875 | 1.861 | 1.854 | 1.740 | 1.887 | 1.934 | 7.627 | 6.079 | 6.139 | 7.761 | 7.758 | 7.738 | 5.981 | |
Ti | 0.019 | 0.028 | 0.028 | 0.029 | 0.031 | 0.069 | 0.020 | 0.014 | 0.016 | 0.438 | 0.293 | 0.038 | 0.028 | 0.027 | 0.444 | |
Altot | 0.145 | 0.183 | 0.169 | 0.186 | 0.203 | 0.289 | 0.144 | 0.076 | 0.409 | 2.017 | 1.999 | 1.191 | 0.176 | 0.176 | 2.049 | |
AlIV | 0.122 | 0.143 | 0.125 | 0.139 | 0.155 | 0.260 | 0.113 | 0.066 | 0.373 | 1.921 | 1.861 | 0.190 | 0.176 | 0.176 | 2.019 | |
AlVI | 0.023 | 0.040 | 0.044 | 0.047 | 0.048 | 0.029 | 0.031 | 0.010 | 0.036 | 0.096 | 0.138 | 1.001 | 0.000 | 0.000 | 0.030 | |
Cr | 0.012 | 0.009 | 0.018 | 0.013 | 0.006 | 0.001 | 0.016 | 0.010 | 0.000 | 0.000 | 0.000 | 0.020 | 0.000 | 0.003 | 0.005 | |
Fe3+ | 0.063 | 0.053 | 0.023 | 0.036 | 0.052 | 0.113 | 0.041 | 0.029 | 0.637 | 0.530 | 1.120 | 0.537 | 0.682 | 0.793 | 0.654 | |
Fe2+ | 0.111 | 0.145 | 0.152 | 0.142 | 0.165 | 0.225 | 0.127 | 0.152 | 0.768 | 1.532 | 1.518 | 0.831 | 0.737 | 0.801 | 1.667 | |
Mn | 0.005 | 0.005 | 0.003 | 0.004 | 0.005 | 0.009 | 0.005 | 0.006 | 0.032 | 0.027 | 0.046 | 0.019 | 0.019 | 0.032 | 0.025 | |
Mg | 0.859 | 0.828 | 0.834 | 0.854 | 0.808 | 0.697 | 0.869 | 0.917 | 3.512 | 2.377 | 1.893 | 3.620 | 3.600 | 3.429 | 2.176 | |
Ca | 0.896 | 0.86 | 0.884 | 0.861 | 0.871 | 0.835 | 0.877 | 0.854 | 1.728 | 1.797 | 1.626 | 1.634 | 1.571 | 1.517 | 1.794 | |
Na | 0.013 | 0.016 | 0.015 | 0.014 | 0.013 | 0.021 | 0.014 | 0.009 | 0.203 | 0.601 | 0.555 | 0.378 | 0.392 | 0.432 | 0.627 | |
K | 0.000 | 0.000 | 0.000 | 0.000 | 0.000 | 0.001 | 0.000 | 0.000 | 0.009 | 0.224 | 0.218 | 0.027 | 0.036 | 0.033 | 0.228 | |
Total | 4.000 | 4.000 | 4.000 | 4.000 | 4.000 | 4.000 | 4.000 | 4.000 | 14.940 | 15.622 | 15.399 | 15.038 | 14.999 | 14.981 | 15.648 | |
Mg# | 80.56 | 85.09 | 84.58 | 85.74 | 83.04 | 75.59 | 87.24 | 85.78 | 82.06 | 60.81 | 55.49 | 81.33 | 83.01 | 81.06 | 56.62 | |
AlVI/AlIV | 0.19 | 0.28 | 0.35 | 0.34 | 0.31 | 0.11 | 0.27 | 0.15 | - | - | - | - | - | - | - |
Chemical compositions in wt%, mineral formulae in atoms per formula unit (apfu). act = actinolite; aug = augite; di = diopside; prg = pargasite; ts = tschermakite; tr = tremolite. Formulae calculated on the basis of 4 cations and 6 oxygens for clinopyroxene; 23 oxygens and 13 cats excluding Ca, Na and K for amphibole. Estimated H2O corresponds 2 (OH) per formular unit for 1 amphibole. Mg# = 100 ∗ (Mg/(Mg+Fe2+)).
Studied dolerite is moderately altered with documented hydrothermal parageneses indicating pumpellyite–prehnite facies conditions [Figure 4a; Šegvić et al. 2022]. Four distinct alteration processes have been observed: (a) prehnitization and pumpellyitization of plagioclase giving rise to the formation of acicular aggregates of prehnite (Figures 2c, d; Table 2) and associated, but less common, microcrystalline acicular Al-pumpellyite (Figure 4a; Table 2), (b) chloritization of clinopyroxene and plagioclase leading to the formation of minute flakey trioctahedral Mg-chlorite of penninite composition (Figures 4b1, b2; Table 2), (c) alteration of clinopyroxene to form a secondary actinolite and tremolite (Figure 3c; Table 1) and (d) transformation of primary amphibole to Fe–Mg rich mica (Figure 2b–d). The pseudomorphosis of amphibole to biotite required an excess of K entering mineral structure while Ca, Na, and/or Mg vacated it at temperatures between 300 and 500 °C [Brimhall et al. 1985].

Classification diagrams for (a) pumpellyite [Fetot–Mg–Al plot [adapted after Passglia and Gottardi 1973; Coombs et al. 1976]], the compositional fields of pumpellyite from the East Taiwan Ophiolite (zeolite facies), the Olympic Peninsula (prehnite–pumpellyite facies) and the Taveyannaz Formation (upper prehnite–pumpellyite and pumpellyite–actinolite facies) were taken from Mével [1981], Coombs et al. [1976] and Rahn et al. [1994]; chlorite (b1) Mg–Fe–VI(Al+Cr+Fe3+) diagram [Zane and Weiss 1998]. (b2) Fe/(Fe+Mg)–Si diagram [after Hey 1954 adapted to Sun et al. 2019] from subvolcanic rocks (dolerite) from the Konj Hill. Masquer
Classification diagrams for (a) pumpellyite [Fetot–Mg–Al plot [adapted after Passglia and Gottardi 1973; Coombs et al. 1976]], the compositional fields of pumpellyite from the East Taiwan Ophiolite (zeolite facies), the Olympic Peninsula (prehnite–pumpellyite facies) and the Taveyannaz Formation (upper ... Lire la suite
Representative chemical compositions and calculated mineral formulae of plagioclase, alkali-feldspar, prehnite, pumpellyite and chlorite from the mafic subvolcanic rocks (dolerites) from the Konj Hill
Mineral | Plagioclase | Alkali-feldspar | Prehnite | Pumpellyite | Chlorite | |||||||||||||
---|---|---|---|---|---|---|---|---|---|---|---|---|---|---|---|---|---|---|
Sample | KN-1 | KN-1 | KN-1 | KN-1 | KN-1 | KN-1 | KN-1 | KN-1 | KN-1 | KN-1 | KN-1 | KN-1 | KN-1 | KN-1 | ||||
Anal. No. | 27 | 32 | 47 | 28 | 30 | 46 | 8 | 20 | 21 | 35 | 56 | 23 | 37 | 17 | ||||
Min. type | ab | ab | ab | sa | sa | sa | - | - | - | Al-pmp | Al-pmp | pen | pen | pen | ||||
SiO2 | 67.23 | 65.78 | 66.28 | 63.76 | 66.59 | 63.54 | 43.31 | 43.66 | 45.81 | 37.38 | 37.31 | 34.59 | 36.64 | 32.10 | ||||
TiO2 | 0.00 | 0.00 | 0.00 | 0.04 | 0.00 | 0.00 | 0.03 | 0.02 | 0.04 | 0.03 | 0.04 | 0.05 | 0.00 | 0.03 | ||||
Al2O3 | 20.71 | 20.94 | 20.84 | 18.98 | 19.13 | 18.60 | 23.74 | 24.22 | 23.79 | 24.92 | 24.84 | 14.56 | 15.44 | 19.08 | ||||
Cr2O3 | 0.00 | 0.00 | 0.00 | 0.00 | 0.00 | 0.00 | 0.00 | 0.00 | 0.02 | 0.00 | 0.00 | 0.06 | 0.03 | 0.00 | ||||
FeO | 0.12 | 0.41 | 0.45 | 0.08 | 0.12 | 0.28 | 0.37 | 0.21 | 0.37 | 5.16 | 5.25 | 12.53 | 10.22 | 12.98 | ||||
MnO | 0.00 | 0.00 | 0.00 | 0.03 | 0.00 | 0.00 | 0.03 | 0.11 | 0.00 | 0.19 | 0.17 | 0.08 | 0.03 | 0.19 | ||||
MgO | 0.00 | 0.00 | 0.00 | 0.02 | 0.02 | 0.00 | 0.00 | 0.00 | 0.00 | 2.35 | 2.31 | 21.07 | 20.37 | 21.56 | ||||
CaO | 0.82 | 1.47 | 1.86 | 0.20 | 0.04 | 0.07 | 26.57 | 26.57 | 24.16 | 22.43 | 22.51 | 1.23 | 1.48 | 0.48 | ||||
Na2O | 11.73 | 10.91 | 10.77 | 0.43 | 0.21 | 0.83 | 0.00 | 0.01 | 1.03 | 0.03 | 0.02 | 0.05 | 0.19 | 0.03 | ||||
K2O | 0.11 | 0.54 | 0.07 | 15.79 | 15.30 | 15.15 | 0.02 | 0.00 | 0.05 | 0.06 | 0.05 | 0.25 | 0.40 | 0.06 | ||||
H2O | - | - | - | - | - | - | 4.72 | 4.76 | 4.83 | 6.47 | 6.46 | 11.87 | 12.13 | 12.14 | ||||
Total | 100.72 | 100.14 | 10.27 | 99.32 | 101.41 | 98.46 | 98.79 | 98.81 | 98.95 | 99.02 | 98.96 | 96.80 | 97.57 | 99.02 | ||||
Si | 2.931 | 2.900 | 2.909 | 2.966 | 3.007 | 2.977 | 3.026 | 3.022 | 3.130 | 3.034 | 3.032 | 3.447 | 3.551 | 3.138 | ||||
Ti | 0.000 | 0.000 | 0.000 | 0.000 | 0.000 | 0.000 | 0.002 | 0.001 | 0.002 | 0.002 | 0.002 | 0.004 | 0.000 | 0.002 | ||||
Altot | 1.063 | 1.087 | 1.077 | 1.040 | 1.017 | 1.026 | 1.955 | 1.976 | 1.916 | 2.384 | 2.379 | 1.734 | 1.800 | 2.221 | ||||
AlIV | - | - | - | - | - | - | - | - | - | - | - | 0.553 | 0.449 | 0.861 | ||||
AlVI | - | - | - | - | - | - | - | - | - | - | - | 1.181 | 1.351 | 1.360 | ||||
Cr | 0.000 | 0.000 | 0.000 | 0.000 | 0.000 | 0.000 | 0.000 | 0.000 | 0.001 | 0.000 | 0.000 | 0.004 | 0.003 | 0.000 | ||||
Fe2+ | 0.004 | 0.015 | 0.017 | 0.003 | 0.005 | 0.011 | 0.022 | 0.012 | 0.021 | 0.350 | 0.357 | 0.701 | 0.357 | 0.786 | ||||
Fe3+ | 0.000 | 0.000 | 0.000 | 0.000 | 0.000 | 0.000 | 0.000 | 0.000 | 0.000 | 0.000 | 0.000 | 0.342 | 0.471 | 0.274 | ||||
Mn | 0.000 | 0.000 | 0.000 | 0.001 | 0.000 | 0.000 | 0.002 | 0.006 | 0.000 | 0.013 | 0.012 | 0.007 | 0.003 | 0.016 | ||||
Mg | 0.000 | 0.000 | 0.000 | 0.001 | 0.001 | 0.000 | 0.000 | 0.000 | 0.000 | 0.284 | 0.280 | 3.130 | 2.943 | 3.142 | ||||
Ca | 0.038 | 0.069 | 0.087 | 0.010 | 0.02 | 0.004 | 1.989 | 1.971 | 1.769 | 1.950 | 1.960 | 0.131 | 0.153 | 0.051 | ||||
Na | 0.992 | 0.932 | 0.917 | 0.039 | 0.018 | 0.075 | 0.000 | 0.001 | 0.136 | 0.005 | 0.003 | 0.019 | 0.072 | 0.012 | ||||
K | 0.006 | 0.036 | 0.004 | 0.937 | 0.881 | 0.906 | 0.002 | 0.000 | 0.004 | 0.006 | 0.005 | 0.063 | 0.099 | 0.015 | ||||
Total | 5.034 | 5.039 | 5.011 | 4.998 | 4.931 | 4.999 | 6.996 | 6.996 | 6.980 | 8.028 | 8.030 | 9.582 | 9.452 | 9.657 | ||||
An | 3.67 | 6.65 | 8.63 | 1.01 | 0.22 | 0.41 | - | - | - | - | - | - | - | - | ||||
Ab | 95.75 | 89.88 | 90.97 | 3.95 | 1.99 | 7.61 | - | - | - | - | - | - | - | - | ||||
Or | 0.60 | 3.50 | 0.40 | 95.00 | 97.80 | 92.00 | - | - | - | - | - | - | - | - | ||||
Mg# | - | - | - | - | - | - | - | - | - | - | - | 81.7 | 89.2 | 79.9 |
Chemical compositions in wt%, mineral formulae in atoms per formula unit (apfu). Mineral formulae calculated on the basis of 8 oxygens and all Fe as Fe2+ for plagioclase and alkali-feldspar; 11 oxygens and all Fe as Fe2+ for prehnite; 12.25 oxygens and all Fe as Fe2+ for pumpellyite (H2O is calculated and corresponds to 2 (OH) and 1.5 (OH) per formular unit in prehnite and pumpellyite, respectively); 14 oxygens and Fe as Fe2+ and Fe3+ for chlorite (H2O is calculated and corresponds to 8 (OH) per formular unit). An = 100 ∗ Ca/(Ca+Na+K); Mg# = 100 ∗ (Mg/(Mg+Fe2+)). ab = albite; sa = sanidine; Al-pmp = aluminium pumpellyite, pen = penninite.
X-ray trace analyses showed dominance of amphibole, prehnite, albite and quartz. Pyrophyllite, chlorite and Fe–Mg mica (phlogopite and biotite) are less abundant. Traces of clinopyroxene were also documented. Chlorite, and to a lesser extent mica and phlogopite, show indications of post-peak hydrothermal activity or weathering in the form of smectite component mixed layering, which gave rise to the formation of chlorite–smectite.
4.2. Geothermobarometric estimations
Amphibole and clinopyroxene phase chemistry sheds light on their crystallization conditions; multiple inosilicate based geothermobarometers were thus employed to unveil at which pressure and temperature the studied dolerite formed. The geothermobarometer based on clinopyroxene composition [Putirka 2008] yielded the maximal crystallization temperatures between 1205 and 1244 °C and pressures of 0.91–1.13 GPa, while the geobarometer of Nimis [1999] and Nimis and Ulmer [1998] returned similar equilibration pressures of 0.98 to 1.37 (±0.2) GPa. This corresponds to crystallization depths of Konj Hill dolerite of ∼29 to 41 km.
Oxygen fugacity gives important insights in liquidus temperature and melt/crystal composition as well as magmatic processes in general [e.g. Kress and Carmichael 1991; Botcharnikov et al. 2005; France et al. 2010]. The ratio of AlIV+Na and AlVI+2Ti+Cr of pyroxene may be used to draw illation on oxygen fugacity [Schweitzer et al. 1979]. The composition of analyzed pyroxene indicate high oxygen fugacity (Figure 5). This is likely an inheritance of the oxidized mantle source modified by fluids and/or melt derived from previously subducted Paleotethyan plate. The P–T conditions of the alteration processes were estimated based on the geochemistry of amphibole (formed by alteration of clinopyroxene) and chlorite. Based on the criteria of Schmidt [1992] amphibole crystallization pressures were set to the range from 0.20 to 0.25 GPa (±0.006 GPa). This corresponds to crystallization depths of ∼6–8 km. Finally, the temperatures of chlorite formation were between 77.6 and 144.7 °C [Cathelineau 1988; Jowett 1991] and 81.9 to 99.7 °C [Kranidiotis and Maclean 1987], which corresponds to very-low grade hydrothermal alteration processes.
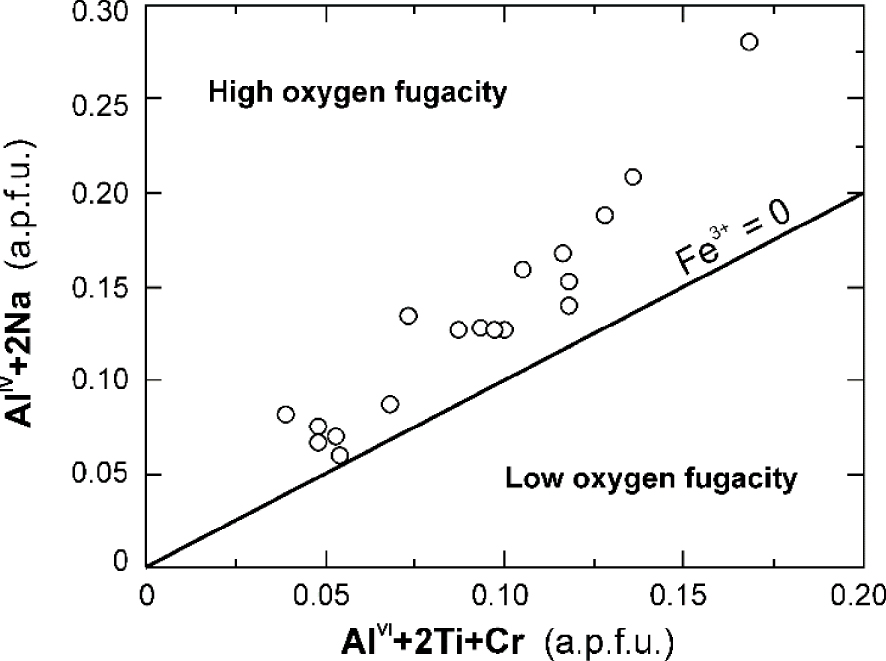
Oxygen fugacity condition of magma during clinopyroxene crystallization [Schweitzer et al. 1979].
4.3. Whole-rock geochemistry
Dolerite representative samples outline a comparable whole-rock chemical composition (Table 3). The concentrations of all measured elements vary in a very narrow span. The content of the most of macroelements is characteristic for mafic subvolcanic rocks [Cox et al. 1979, Chapter 2; Wilson 1989, Chapters 5 and 7]; however, high MgO (9.28–10.31 wt%), CaO (up to 12.05 wt%) and LOI (8.70–11.57 wt%) coupled with low K2O (<0.99 wt%) are typical characteristics of the investigated dolerite. Elevated LOI values reflect medium to high degrees of sea-floor alteration [Polat et al. 2002; Polat and Hofmann 2003]. Dolerite samples were found to be SiO2-undersaturated with the following CIPW normative abundances: anorthite (21–26%), diopside (16–32%), hypersthene (8–21%) and olivine (2–4%). The analyzed rocks are sub-alkaline calc-alkaline basalts (Figures 6a, b). They are characterized by high contents of Ni (202–239 ppm), Cr (592–768 ppm), Co (37–45 ppm) and Mg#(>70.3). Other compatible elements (e.g. Sc, Sr, V; Table 3) do not deviate from the spans commonly reported in mafic volcanic/subvolcanic rocks [e.g. Wilson 1989].
Chemical analyses of the mafic subvolcanic rocks (dolerites) from the Konj Hill
Sample | KN-1 | KN-2 | KN-3 | KN-4 | KN-5 |
---|---|---|---|---|---|
SiO2 | 42.85 | 42.36 | 43.18 | 44.51 | 44.62 |
TiO2 | 1.08 | 1.11 | 0.99 | 0.98 | 0.86 |
Al2O3 | 11.57 | 11.43 | 11.72 | 12.17 | 12.24 |
Fe2O3total | 8.27 | 8.01 | 8.16 | 8.04 | 8.32 |
MnO | 0.16 | 0.15 | 0.15 | 0.16 | 0.16 |
MgO | 10.24 | 10.31 | 9.86 | 9.34 | 9.28 |
CaO | 12.16 | 12.18 | 12.10 | 12.10 | 12.05 |
Na2O | 1.24 | 1.31 | 1.42 | 1.55 | 1.59 |
K2O | 0.96 | 0.81 | 0.87 | 0.84 | 0.99 |
P2O5 | 0.23 | 0.24 | 0.21 | 0.20 | 0.19 |
LOI | 10.70 | 11.57 | 10.86 | 8.70 | 9.13 |
Total | 99.61 | 99.48 | 99.52 | 99.65 | 99.43 |
Mg# | 72.27 | 72.41 | 71.51 | 70.39 | 70.26 |
Cs | 2.4 | 2.5 | 2.4 | 2.3 | 2.2 |
Rb | 32 | 34 | 29 | 28 | 27 |
Ba | 248 | 251 | 232 | 227 | 221 |
Th | 3.3 | 3.2 | 3.3 | 3.4 | 3.5 |
Ta | 0.5 | 0.5 | 0.5 | 0.5 | 0.6 |
Nb | 7.4 | 7.3 | 7.7 | 7.9 | 8.9 |
Sr | 352 | 360 | 342 | 331 | 330 |
Zr | 88 | 88 | 88 | 94 | 95 |
Hf | 2.3 | 2.2 | 2.3 | 2.4 | 2.5 |
Y | 18.3 | 18.1 | 19.2 | 21.3 | 21.8 |
Sc | 26 | 25 | 26 | 26 | 27 |
V | 205 | 207 | 203 | 201 | 200 |
Cr | 760 | 768 | 721 | 596 | 592 |
Ni | 237 | 239 | 212 | 209 | 202 |
Co | 44 | 45 | 42 | 39 | 37 |
Pb | 3.5 | 3.7 | 2.9 | 2.4 | 2.4 |
La | 19.4 | 19.1 | 19.9 | 21.3 | 21.9 |
Ce | 43.1 | 43.0 | 44.6 | 48.4 | 49.3 |
Pr | 5.41 | 5.39 | 5.82 | 6.15 | 6.22 |
Nd | 22.6 | 22.3 | 24.2 | 26.1 | 26.8 |
Sm | 4.94 | 4.89 | 5.08 | 5.49 | 5.59 |
Eu | 1.27 | 1.24 | 1.32 | 1.44 | 1.48 |
Gd | 4.40 | 4.35 | 4.65 | 5.02 | 5.09 |
Tb | 0.63 | 0.60 | 0.68 | 0.71 | 0.74 |
Dy | 3.64 | 3.61 | 3.76 | 4.06 | 4.14 |
Ho | 0.69 | 0.65 | 0.73 | 0.77 | 0.82 |
Er | 1.97 | 1.92 | 2.21 | 2.27 | 2.33 |
Tm | 0.26 | 0.25 | 0.27 | 0.29 | 0.30 |
Yb | 1.58 | 1.56 | 1.68 | 1.71 | 1.74 |
Lu | 0.23 | 0.22 | 0.24 | 0.26 | 0.27 |
Major elements in wt%, trace elements in ppm. LOI = loss on ignition at 1100 °C. Mg# = 100 ∗ molar (MgO/(MgO+FeOtotal)).

(a) Classification diagram Zr/TiO2 ∗ 0.0001–Nb/Y after Winchester and Floyd [1977], (b) ThN–NbN discrimination diagram simplified after Saccani [2015] for the mafic subvolcanic rocks (dolerite) from the Konj Hill. Abbreviations: AB = alkali basalts; CAB = calc-alkaline basalts; E-MORB = enriched mid-ocean ridge basalts; IAT = island arc tholeiite; N-MORB = normal mid-ocean ridge basalts; PM = primitive mantle.
Zirconium on the one hand and high field strength elements (HFSE) and rare-earth elements (REE) (not shown) on the other hand outline well defined linear correlation which means that secondary remobilization of these elements was minimal. Conversely, large ion lithophile elements (LILE) show pronounced selective mobilization and will be therefore excluded from further petrogenetic considerations.
The N-MORB normalized element abundances feature uniform normalization patterns with significant enrichment in LILE (Cs, Rb, Ba and K) and Th compared to HFSE and mid- and heavy-rare-earth elements (MREE and HREE) at levels of 11 to 320 times relative to N-MORB (Figure 7a). Studied dolerite shows significantly pronounced negative anomalies of the Nb–Ta pair relative to La [(Nb/La)N-MORB = 0.39–0.43], as well as of Zr–Hf and Ti which is typical of subduction-related magmas [e.g. Pearce et al. 1984; Hofmann 1997; Wang et al. 2016; Figure 7a]. A weak to moderate Pb anomaly relative to Ce [(Pb/Ce)N-MORB = 1.21–2.15] is another typical feature of studied rocks. The chondrite normalized REE patterns show moderate enrichment in light-rare-earth elements (LREE) over HREE [(La/Lu)CN = 8.09–8.53] at 31–60 times chondrite relative concentrations and nearly flat HREE profiles [(Tb/Lu)CN = 1.70–1.77] at 7–12 times relative to chondrite (Figure 7b). A low intensity of Eu anomaly (Eu/Eu* = 0.81–0.83) in dolerite samples marks an early fractionation of plagioclase.

(a) N-MORB-normalized multielement and (b) REE patterns for the mafic subvolcanic rocks (dolerite) from the Konj Hill. Normalization values are from Sun and McDonough [1989]. Fields for active continental margin-continental arc calc-alkaline basaltic andesites in the Andes [Wilson 1989, Chapter 7] are plotted for correlation constraints.
The values of 143Nd/144Nd ratios of two representative dolerite samples fit a narrow span between 0.512352 and 0.512359 (Table 4). The initial εNd are calculated for 242 Ma, which is the time of intensive volcanic activity in the larger area [Ščavničar et al. 1984]. The initial εNd varies between −3.29 (±0.51) to −3.59 (±0.51). Values of 147Sm/144Nd and εNd(t) (Figure 8) are characteristic for the subduction of crustal derived material [SCM; Swinden et al. 1990].

147Sm/144Nd—εNd(240 Ma) diagram for the mafic subvolcanic rocks (dolerite) from the Konj Hill. Hypothetical mantle sources: DM—depleted mantle (not refractory), VDM—very depleted mantle (refractory), SJM—subducted juvenile material (subducted oceanic crust; slab with little pelagic sediment) and SCM—subducted continental material. The observed compositions and hypothetical end members sources calculated for the Middle Triassic following Swinden et al. [1990].
Nd isotope data of the mafic subvolcanic rocks (dolerites) from the Konj Hill
Sample | Sm/Nd | 147Sm/144Nd | 143Nd/144Nd | 143Nd/144Nd(t) | 𝜀Nd(t)a | Time (t)b |
---|---|---|---|---|---|---|
KN-1 | 0.21858 | 0.132135 (7 × 10−3) | 0.512352 (6 × 10−6) | 0.512143 (3 × 10−5) | −3.59 (0.51) | 242 ± 12.1 Ma |
KN-3 | 0.20991 | 0.126901 (6 × 10−3) | 0.512359 (6 × 10−6) | 0.512158 (2 × 10−5) | −3.29 (0.51) | 242 ± 12.1 Ma |
Errors in brackets for Nd isotopic ratios are given at the 2𝜎-level. The method of calculating the errors is presented in the analytical techniques chapter. 147Sm/144Nd calculated from the ICP-MS concentrations of Sm and Nd following equation: 147Sm/144Nd = (Sm/Nd) ∗ [0.53151 + 0.14252 ∗ 143Nd/144Nd]. aInitial 𝜀Nd(t) calculated assuming I
5. Discussion
Middle Triassic(?) dolerite documented in the vicinity of the city of Knin in northern Dalmatia represents the biggest occurrence of mafic sub-volcanic rocks in the central part of External Dinarides. Its primary stratigraphic position with respect to the neighboring rocks (Figures 1b1, b2) cannot be unequivocally defined [Grimani et al. 1975]. A Middle Triassic affiliation to the volcano-sedimentary succession is, however, advised based on the correlation with coeval igneous rocks from the broader Dinaridic region [Ščavničar et al. 1984; De Min et al. 2009].
5.1. Dolerite petrogenesis
The origin of studied dolerite may be reconstructed based off the ratios of trace elements and Nd isotopic data. Generally, low values of Ba/Th and their steady increase coupled with the low (La/Sm)N (Figure 9) are in favor of a pervasive fluid metasomatism. The fluids likely originated from a previously subducted slab [Pearce 1983; Plank 2005]. Basaltic magmas affected by crustal contamination have La/Nb > 1.5, La/Ta > 22, and K/P > 7 [Hart and Zindler 1989]. Studied rocks however feature higher values of these ratios (La∕Nb = 2.5–2.7; La/Ta = 36.5–42.6; K∕P = 5.8–9.0) which suggests that their parental magmas experienced weak to moderate crustal contamination. A comparable degree of crustal contamination is inferred from elevated values of Th/Yb (1.82–1.88) and Th/Ta (5.8–6.8) as well as positive Pb spikes (up to 2.2; Figure 7a) [Taylor and McLennan 1985]. Negative values of initial εNd (−3.29 to −3.59) accompanied by the very low values of 147Sm/144Nd ratio (⩽0.126901; Figure 8) are in line with inferences on significant crustal interference. Advocated crustal contamination might have stemmed from a slow and prolonged magma uplift through the continental crust [e.g., Huppert and Stephen 1985; Pomonis et al. 2004] and/or subducted/recycled sediments.

Ba/Th–(La/Sm) −N diagram [after Elliott 2003] for the the mafic subvolcanic rocks (dolerite) from the Konj Hill. Abbreviations: [N-MORB = normal mid-ocean ridge basalts; E-MORB = enriched MORB; OIB = ocean island basalts; PM = primitive mantle Sun and McDonough 1989]; [UCC = upper continental crust; LCC = lower continental crust Taylor and McLennan 1985]; GLOSS = global subduction sediment [Plank and Langmiur 1998]; DMM = depleted MORB mantle [Workman and Hart 2005]. Masquer
Ba/Th–(La/Sm) −N diagram [after Elliott 2003] for the the mafic subvolcanic rocks (dolerite) from the Konj Hill. Abbreviations: [N-MORB = normal mid-ocean ridge basalts; E-MORB = enriched MORB; OIB = ocean island basalts; PM = ... Lire la suite
Balanced and high La/YbPM slopes (∼15–17) as well as negative Zr–Hf anomalies (Figure 7a) may call for presence of residual garnet [Xie et al. 1993], which suggests that magmas that gave rise to the studied dolerite originated in the garnet-facies mantle. Using the Dy/Yb vs. Yb diagram (Thirwall et al., 1994; Figure 10) we therefore modelled the non-modal partial melting of a spinel peridotite, a garnet peridotite and a spinel–garnet peridotite mantle source. Accordingly, the studied rocks show a consistently narrow span along the evolution line for a spinel–garnet bearing peridotite mantle source (60sp:40grt) where partial melting likely ranged from 11 to 13%. This is corroborated by moderately elevated values of Sm/YbPM (3.1–3.3), which is indicative for melting in the spinel–garnet stability field. The above suggests the formation of melt in the garnet-facies, which was later mixed with the magmas generated at shallower mantle levels where residual spinel prevailed. This points out that studied rocks formed from a unique mantle source compared to similar Permian to Triassic igneous rocks cropping out elsewhere in External Dinarides and the Zagorje-Mid-Transdanubian Zone further to the north-west (Figures 1a and 10). To get more insights into the characteristics of studied rocks’ mantle characteristics we utilized the Th/Yb vs. Ta/Yb discrimination diagram (Figures 11a, b). Enrichment in Th with regards to Ta and Yb indicates an origin from the sources modified by subduction-related metasomatism [Pearce 1982].

Yb–Dy/Yb diagram for the mafic subvolcanic rocks (dolerite) from the Konj Hill. Partial melting curves are shown for the non-modal batch melting of spinel and garnet lherzolite sources, starting from a Primitive Mantle [PM; McDonough and Frey 1989] material. Mineral and melt modes for spinel and garnet-lherzolite source: Ol0.58(0.10) + Opx0.27(0.27) + Cpx0.12(0.50) + Sp0.03(0.13) [Kinzler 1997] and Oll0.60(0.05) + Opx0.21(0.20) + Cpx0.08(0.30) + Gt0.12(0.45) [Walter 1998], respectively. Italic numbers in parentheses indicate the percentages of each mineral entering the liquid. Partition coefficients are from McKenzie and O’Nions [1991]. Masquer
Yb–Dy/Yb diagram for the mafic subvolcanic rocks (dolerite) from the Konj Hill. Partial melting curves are shown for the non-modal batch melting of spinel and garnet lherzolite sources, starting from a Primitive Mantle [PM; McDonough and Frey 1989] material. ... Lire la suite
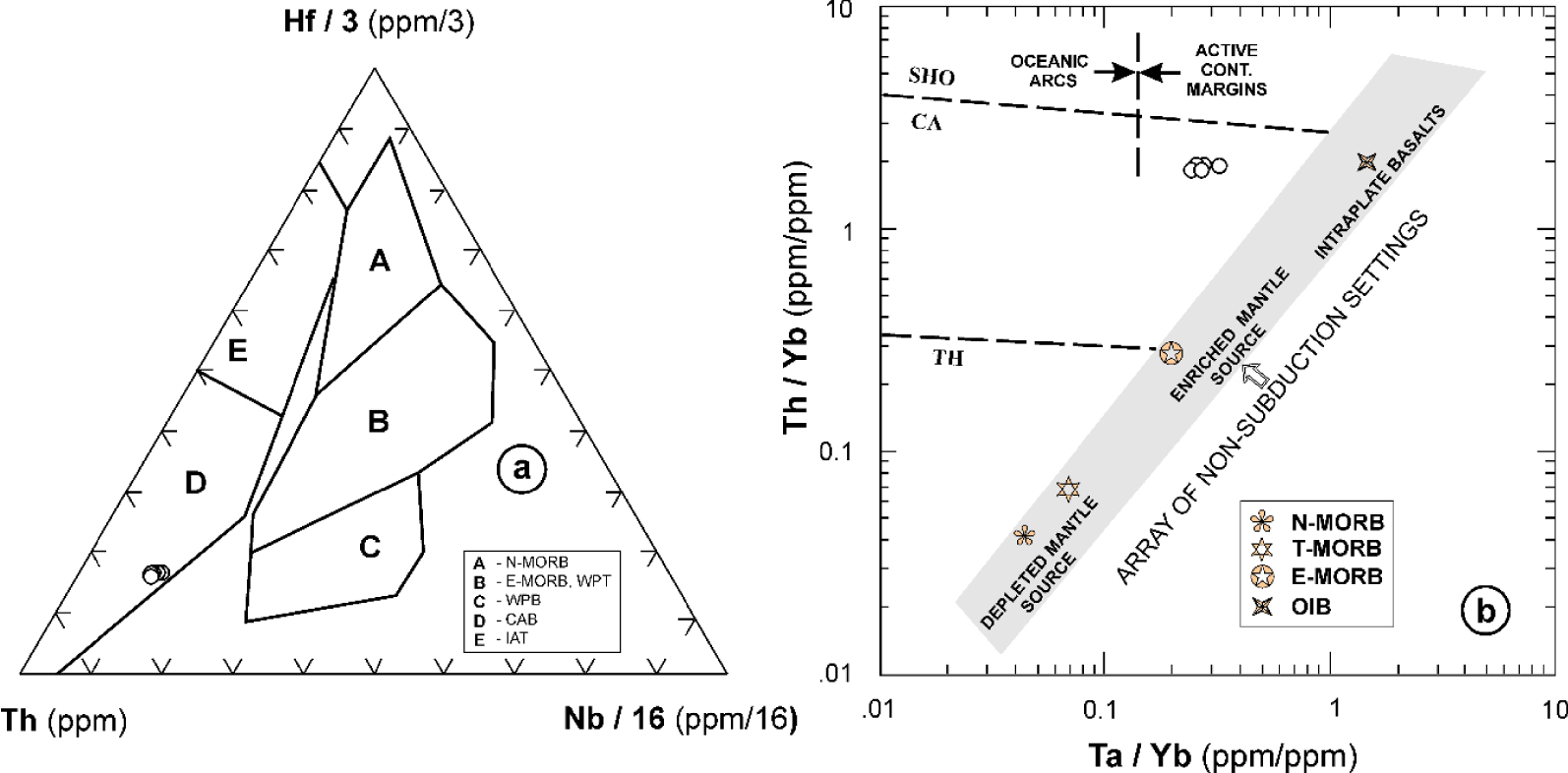
Discrimination diagrams for the mafic subvolcanic rocks (dolerite) from the Konj Hill. (a) Th—Nb/16–Hf/3 diagram [Wood 1980]. A—normal mid-ocean ridge basalts (N-MORB); B—enriched MORB (E-MORB) and within-plate tholeiites (WPT); C—alkaline within-plate basalts (AWPB); D—calc-alkali basalts (CAB); E—island-arc tholeiites (IAT). (b) Ta/Yb—Th/Yb diagram [Pearce 1983]. N-MORB, E-MORB and OIB are from Sun and McDonough [1989] and T-MORB are from Klein [2003].
Considering high values of Mg#(>70), Ni (>200 ppm) and Cr (>500 ppm) measured in the investigated dolerite one may hypothesize its mantle origin; these values furthermore indicate the composition which corresponds to near-primary melt. In order to estimate melting conditions in the source the empirical model of Wood [2004] has been utilized. It relies on pressure, temperature, MgO and total alkalis in equilibrium with olivine, orthopyroxene and clinopyroxene. The estimations of melting pressures and temperatures thus vary from 0.86 and 1.21 (±0.33) GPa and 1265 and 1298 (±30) °C, respectively. This correlates well with the obtained P–T clinopyroxene conditions of 0.91 to 1.37 GPa and 1205 to 1244 °C, respectively. Mantle source(s) of such complex melts existed at depths between 26 and 41 km which corresponds to middle and upper segments of lithospheric mantle. A low Nb/La (0.37–0.41) corroborates their lithospheric origin [Smith et al. 1999].
In brief, one may hypothesize that analyzed dolerite was formed through complex processes of low-grade partial melting of the (a) subduction-modified oceanic lithosphere and/or subducted/recycled sediments beneath a metasomatically altered active mantle wedge and (b) lithospheric mantle which has been modified by the crustal component possibly during the melting of lower crustal material, (Figures 7a, 8 and 13a2). Alternatively, the investigated calc-alkaline rocks could have been generated by partial melting of subduction-modified heterogeneous hydrated lithospheric (subcontinental) mantle (Figure 13b2). This mantle portion had likely been contaminated and/or metasomatized during earlier Hercynian subduction events in the Late Paleozoic when local geotherms were raised due to passive upwelling of asthenospheric mantle along rifted margins (Figure 13b1).
5.2. Tectonomagmatic significance
To unveil tectonomagmatic provenance in which the studied rocks formed, the discrimination diagrams based on immobile trace elements and REE were utilized (ThN–NbN, Hf/3–Th–Nb/16 and Th/Yb vs. Ta/Yb; Figures 6b, 11a, b). The studied dolerite was clearly formed in a subduction-related environment defined by a volcanic arc setting which evolved in an active, Andean-type, continental margin environment (Figure 12a). Further lines of evidence suggesting arc-related parental magmas are: (a) negative anomalies of Nb–Ta, Zr–Hf and Ti (Figure 7a) and retention of these elements in the slab [Pearce 1982, 1983; Arculus and Powell 1986; Hawkesworth et al. 1993, 1997; Wang et al. 2016], (b) normalization curves trends in chondrite-normalized REE diagrams (Figure 7b), and (c) clinopyroxene geochemistry (Figure 12b). Investigated dolerite rocks may therefore be classified as orogenic and are marked with typical calc-alkaline trends, which is a convergent geodynamic feature widely documented in the Dinarides, the Hellenides, and Southern Alps [Castellarin et al. 1988].
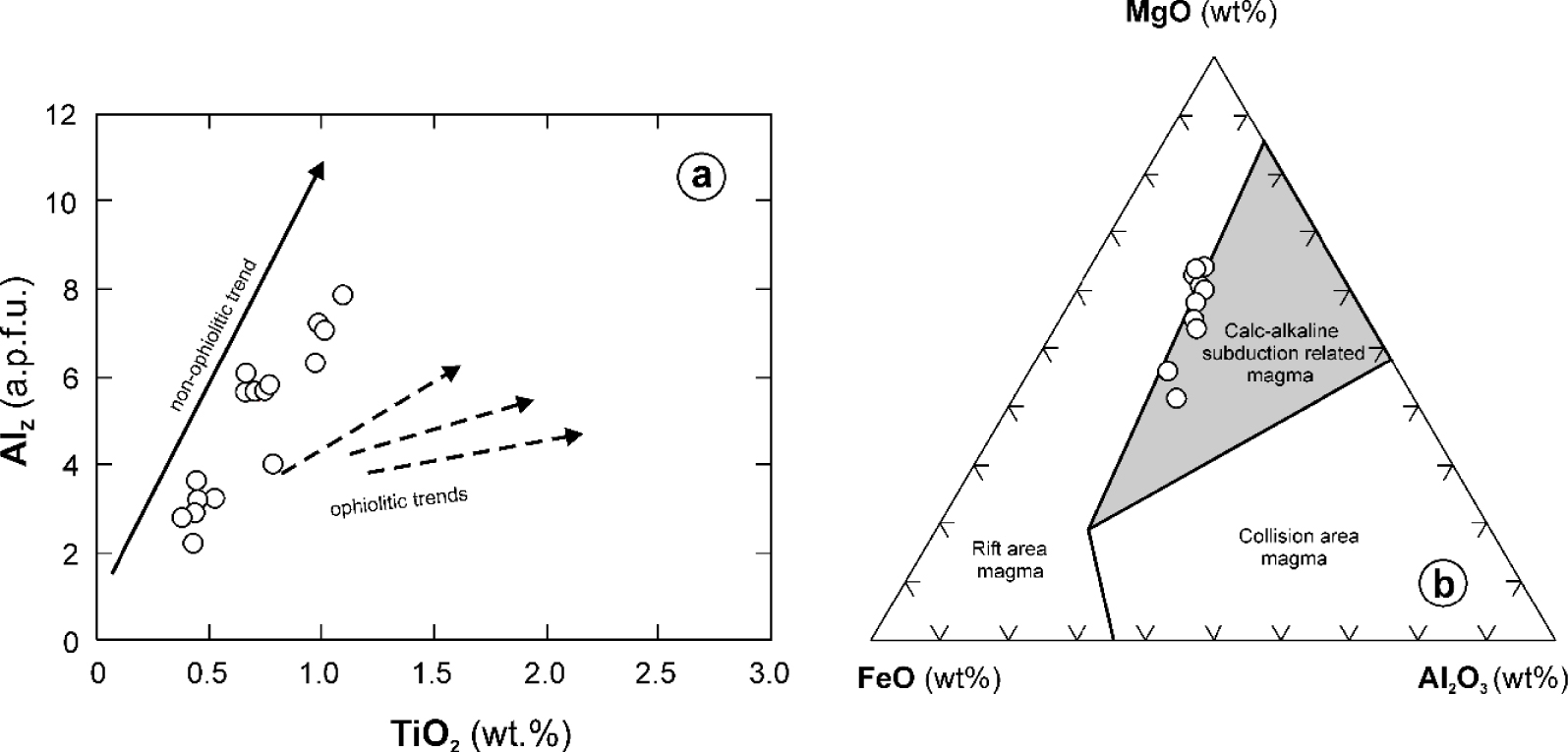
Discrimination diagrams (a) AlZ (AlIV*100/2)–TiO2 [Loucks 1990], (b) FeO–Al2O3–MgO [Le Bas 1962] for pyroxene from the mafic subvolcanic rocks (dolerite) from the Konj Hill.
5.3. Geodynamic significance

Middle to LateTriassic palaeogeographic reconstruction of the Mediterranean region: (a1) Anisian–Ladinian (∼240 Ma) and (a3) Rhaetian (∼200 Ma) simplified after Stampfli and Borel [2004] [Ad = Adria Plate s. str., Ap = Apulia s. str., Bü = Bükk, Cn = Carnic-Julian, Hy = Hydra, Pl = Pelagonian, sK = south-Karawanken fore arc, Sl = Slavonia, SM = Serbo-Macedonian, TD = Trans-Danubian, Tz = Tisza, Vo = Vourinos (Pindos)] and (b1) Anisian–Ladinian (∼240 Ma) and (b3) Rhaetian (∼200 Ma) simplified and slightly modified after van Hinsbergen et al. [2020] [Da = Dalmatian Nappe, Di = Drina-Ivanjica Nappe, Eb = East Bosnian-Durmitor Nappe, Jk = Jadar-Kopaonik Nappe, Pk = Pre-Karst Nappe] with location of investigation area of Konj Hill (marked with black arrow and red dot symbol). Schematic geodynamic model (scale is approximate) for: (a2) interaction of active continental margin magmatic ativity (subduction-related ensialic volcanic arc magmatism) and roughly contemporaneous proto back-arc rifting and (a4) drifting to spreading of oceanic domain in Meliata-Maliak-Pindos ocean system [slightly modified after Slovenec et al. 2020] according to Stampfli and Borel [2004] palaeogeographic reconstruction shown in a1. (b2) Ensialic infant arc–back-arc rifting and (b4) spreading of oceanic domain in the central part of the Mediterranean region according to van Hinsbergen et al. [2020] palaeogeographic reconstruction shown in b1. CAB = calc-alkaline and shoshonitic basalts/volcaniclastites, AB = alkaline basalts, OIB = ocean island basalts, N-MORB = normal mid-ocean ridge basalts; E-MORB = enriched MORB; P-MORB = plume MORB; 1 = mantle diapires, 2 = oceanic crust topped by radiolarian cherts, 3 = partially melted previous subducted oceanic lithosphere, 4 = zone of partial melting and contamination by continental crust, 5 = fluids from previous subducted slab. Masquer
Middle to LateTriassic palaeogeographic reconstruction of the Mediterranean region: (a1) Anisian–Ladinian (∼240 Ma) and (a3) Rhaetian (∼200 Ma) simplified after Stampfli and Borel [2004] [Ad = Adria Plate s. str., Ap = Apulia s. str., Bü ... Lire la suite
Present paleogeographic reconstructions and geodynamic concepts of Triassic geology of the broader Mediterranean region suggested by Stampfli and Borel [2002, 2004] and Stampfli and Hochard [2009] on the one hand and van Hinsbergen et al. [2020] and van Hinsbergen and Schouten [2021] on the other hand show disagreements related to geotectonic interpretations of geological events. Both concepts, however, include an active Middle to Late Triassic subduction of the Paleotethys to drive the Neotethyan ocean spreading (Figure 13). With reference to those two paleogeographic concepts (Figures 13a1, a3 and b1, b2) two geodynamic models are here proposed (Figures 13a2, a4 and b2, b4). They are based on different hypotheses of geo-tectonomagmatic interpretation:
(1) The geodynamic concept introduced by Stampfli and Borel [2002, 2004] and Stampfli and Hochard [2009] advocates Middle Permian to Middle Triassic north-directed subduction of the Paleotethys beneath the Laurasian (south European) active margin followed by the final closure of this oceanic realm in the Late Triassic (Figure 13a1–a4). As a result, further to the north, several smaller rift belts and then back-arc basins [Meliata, Maliak, Pindos; e.g. Stampfli et al. 2013] came to being accompanied by the magmatism linked to an active subduction or rifting-related processes [Pe-Piper 1998; Bortolotti et al. 2004, 2006; Neubauer et al. 2019; Lustrino et al. 2019; De Min et al. 2020]. These processes set path to Triassic magmatism and its intrusive, effusive and pyroclastic manifestations in the Dinarides, Albanides, Hellenides, Southern Alps and the Trans-Danubian region [Bébien et al. 1978; Castellarin et al. 1980, 1988; Pe-Piper and Panagos 1989; Pe-Piper and Mavronichi 1990; Capedri et al. 1997; Bortolotti et al. 2006; Monjoie et al. 2008; Obenholzner 1991; Harangi 1996; Trubelja et al. 2004; Smirčić et al. 2018, 2020; Lustrino et al. 2019; Slovenec et al. 2020; Slovenec and Šegvić 2021]. One of such active Middle Triassic rifts within the Adria Plate stretched along the present day External Dinarides [Stampfli and Borel 2003; Figure 13a1]. This extensional area therefore represents a back-arc rift basin of the Paleotethys which was subducted during the Triassic. This process was however halted already in the Ladinian because of regional geotectonic events which gave rise to the concept of the Dinaridic aborted rift [Smirčić 2017]. Geochemical and isotopic data as well as mineralogy and petrogenesis of investigated dolerite are all in line with the geodynamic model connected to Middle Triassic northwards active(?) subduction of Paleotethyan lithosphere beneath the Laurussia (Figures 7, 11, 13a1–a2). Geochemical signatures of dolerite were likely inherited from the Paleotethyan subduction slab. Presented geochemistry is furthermore characteristic for Andean-type arc-related magmas of active continental margins [Wilson 1989, Chapter 7]. This is in line with the inferences on the origin of Middle to Upper Triassic volcanic rocks in the Hellenides and Albanides [Pe-Piper and Panagos 1989; Pe-Piper and Mavronichi 1990; Capedri et al. 1997; Pe-Piper 1998; Bortolotti et al. 2004, 2006; Monjoie et al. 2008; Chiari et al. 2012].
Dolerite parental magmas of the investigated rocks were likely generated at depths up to ∼40 km and are related to upper to middle levels of lithospheric mantle. During the Late Anisian the west Paleotethys was characterized by passive upwelling of asthenospheric mantle, i.e. uplift of a mantle dome that, in turn, caused an adiabatic decompression along a system of subparallel faults [Slovenec et al. 2020]. In that sense the paleogeographic area of the Adria Plate and Dinarides experienced an extension coupled with subsidence which led to the formation of locally aborted rift systems which evolved into a range of intracontinental basins behind the peri-continental volcanic arc [Vlahović et al. 2005; Smirčić 2017; Smirčić et al. 2018]. The basins were prone to the accumulation of sedimentary material depending on the sea bathymetry. During Middle Triassic along one of those ephemeral and undeveloped aborted rift fault systems in External Dinarides investigated dolerite was crystallized (Figure 13a2). Finally, the regional geotectonic events during the Middle to Late Triassic in the area of External Dinarides dictated an abrupt closure of this passive syn-rift system which hence failed to evolve into an open ocean. Instead, a continuous terrigenous sedimentation ensued with a lack of magmatic activity after the Late Triassic time [Pamić and Balen 2005; Vlahović et al. 2005, 2012].
It is important to note that during Middle Triassic time the arc-related magmatism of the southern active European continental margin was coeval with the proto back-arc rifting of the intracontinental lithosphere behind the pericontinental volcanic arc (Figure 13a2). The arc-related magmatism was however not genetically linked with the ophiolites generated in the Meliata-Maliak-Pindos back-arc basins. As a result of the upper Anisian uplift of mantle dome the carbonate platform gets disintegrated along the continental margin of the north-western segment of the Paleotethys. This was followed by the proto back-arc rifting of the intracontinental lithosphere behind the pericontinental volcanic arc [e.g. Slovenec et al. 2011; Figure 13a2]. The rifting produced uncontaminated primitive (OIB-type) alkali lavas free of crustal contamination (Figure 13a2). This calls for a significant rollback and retreat of the subducted sinking slab [Slovenec et al. 2010, 2011]. Further Middle-Late Triassic events in the western segment of Paleotethys included a terminal intracontinental rifting which prograded into an initial drifting and spreading of an ensialic back-arc basin [Slovenec et al. 2011; Figure 13a3–a4], which belonged to the larger Meliata-Maliak-Pindos Neotethyan oceanic system developed along the Laurasian (southern European) margin during Paleotethyan final subduction stages [Bortolotti et al. 2013; Stampfli et al. 2013; Stampfli and Borel 2002, 2004]. The oldest spreading ridge oceanic crust related to the proto oceanization is represented by P-MORB-type and, thereafter, E-MORB-type volcanic rocks [e.g., [Slovenec et al. 2011]; Figure 13a4]. The newly formed oceanic realm in the western segment of Neotethys has continuously expanded during the Late Triassic and Early Jurassic leading to the formation of N-MORB-type oceanic crust. The occurrence of Middle-Late Triassic alkaline (OIB-type) and tholeiitic (P-, E-, N-MORB) volcanism in the Dinarides [e.g., Pamić 1982; Vishnevskaya et al. 2009], in the Albanide-Hellenide belt [e.g. Pe-Piper and Panagos 1989; Pe-Piper and Mavronichi 1990; Capedri et al. 1997; Pe-Piper 1998; Bortolotti et al. 2004, 2006; Monjoie et al. 2008; Chiari et al. 2012] and in the Bükk Mts. of Hungary [e.g., Harangi 1996; Velledits 2004, 2006] indicates a large-scale rifting of the continental lithosphere, followed by spreading and formation of the oceanic crust between the Apulia/Adria Plate and the continental margin of the southern Laurussia [e.g. Bortolotti et al. 2013; Saccani et al. 2015, and references therein].
(2) According to the geodynamic concept of van Hinsbergen et al. [2020] and van Hinsbergen and Schouten [2021] school of thoughts the basins and orogens of the Mediterranean region initiated the opening of the Eastern Mediterranean Ocean and the Balkan Neotethys Ocean during the early break-up of Pangea since the Triassic time. Beginning with the Jurassic these forms were obliterated through the subduction and terminal convergence between the African and Eurasian Plates. The opening of the Balkan Neotethys Ocean was a consequence of east-directed subduction of Balkan Paleotethys Ocean below the northeastern Adria/Dacia margin accompanied by the gradual northeastward roll-back of the Balkan Paleotethys slab (Figures 13b1 and b2). In such context the Mediterranean region represents the plate boundary zone between the African and Eurasian plates (Figure 13b1). With the opening of the oceanic basins in the area, the continental fragments separated from Eurasia and Gondwana were rifted and internally deformed into platforms and local basins [Dercourt et al. 1986, 2000]. The subduction of the oceanic and continental lithosphere was related to the stacking of upper crust at subduction plate boundaries, forming the regional nappe stacks of the Alps, the Carpathians, and the Dinaride-Hellenides, whereas their original lower crustal and mantle underpinnings had however subducted [Schmid et al. 2008; van Hinsbergen et al. 2005]. In that sense the Dinarides, as a part of the Greater Adria, placed within the North African margin, were composed of several continental-derived nappes (Figure 13b1). One of those was the High Karst nappe characterized by shallow water Triassic sedimentation interrupted solely by a short episode of Middle Triassic deepening and rifting-related volcanism. This has led to subsidence and formation of the Dinaridic aborted rift [Smirčić 2017]. Studied Middle Triassic (?) dolerite spatially associated with the High Karst unit, according to this geodynamic concept, characterize a syn-rift stage of the Dinaridic aborted rift (Figures 13b1 and b2).
The previous paragraph has shown that the origin of investigated dolerite, according to the model of van Hinsbergen et al. [2020] and van Hinsbergen and Schouten [2021] (Figure 13b1), may explain the petrogenetic model suggested by Saccani et al. [2015]. Contrary to the concept already discussed (proposed by Stampfli and Borel [2002, 2004] and Stampfli and Hochard [2009]; Figure 13a2) this alternative model (Figure 13b2) does not assume influence of an active subduction. This suggests that calc-alkaline rocks might have been generated by partial melting of the heterogeneous hydrated lithospheric (subcontinental) mantle metasomatized during older Variscan subductions. Late Paleozoic Hercynian subduction events which led to the formation of an Andean-type infant arc along the northwestern margin of Gondwana likely played an important role in hydrating the subcontinental lithospheric mantle [Pe-Piper 1998]. An inference can therefore be made putting forth the formation of studied dolerite at the time of Middle Triassic extension and ephemeral syn-rift episode (Figure 13b2). The petrogenesis of dolerite is analogue to those of similar rocks found in the Albanide-Hellenide belt, especially with those from the southern Hellenides [Pe-Piper and Panagos 1989; Pe-Piper and Mavronichi 1990; Capedri et al. 1997; Pe-Piper 1998; Monjoie et al. 2008; Chiari et al. 2012] and Southern Alps [e.g. Bonadiman et al. 1994; Lustrino et al. 2019]. Contemporaneous to Middle Triassic formation of calc-alkaline and shoshonitic rocks at the junction area of the Greater Adria and Dacia plates an initial rifting of intracontinental lithosphere took place (Figure 13b1–b2). The rifting was characterized by local passive upwelling and mixing of primitive asthenospheric mantle and metasomatized mantle producing OIB-type alkali lavas lacking a crustal contamination and that of the subducted Balkan Paleotethys slab. Later Middle to Late Triassic events in the area included an initial drifting and spreading (Figure 13b3–b4) which gave rise to the development of oceanic crust represented by P-MORB-type and then E-MORB-type and N-MORB-type volcanic rocks in the Dinaride-Albanide-Hellenide belt [e.g. Pamić 1982; Capedri et al. 1997; Pe-Piper 1998; Trubelja et al. 2004; Bortolotti et al. 2004, 2006; Monjoie et al. 2008; Vishnevskaya et al. 2009; Chiari et al. 2012; Koutsovitis 2020]. The newly formed oceanic realm, known as the Balkan Neotethys Ocean [van Hinsbergen et al. 2020], progressively expanded during the Late Triassic and Early Jurassic time (Figure 13b3–b4).
Presented geodynamic models seem to be both acceptable; this study, however, gives preference to the second one that does not include a contemporaneous active subduction because, heretofore, there is no geological evidence in favor of its existence. Considering a high degree of resemblance of studied Dinaridic dolerite with its Triassic equivalents from the Albanide-Hellenide segment of Neotethys and extant geological data it is suggested that Triassic extension and ensuing incipient oceanization at the ocean-continent transition zones were not associated with a major mantle plume event or a contemporaneous active subduction [Pe-Piper 1998].
6. Conclusions
- Subvolcanic calc-alkaline dolerite rocks that crop out in the vicinity of the city of Knin in the central part of External Dinarides belongs to the High Karst Unit.
- Petrography of the studied rocks revealed the crystallization order: clinopyroxene → plagioclase → alkali-feldspar ± Ca-amphibole ± Fe–Ti oxides.
- The estimations of melting pressure and temperature vary from 0.86 and 1.21 (±0.33) GPa and 1265 and 1298 (±30) °C, respectively. This is in line with P–T clinopyroxene conditions of 0.91 to 1.37 GPa and 1205 to 1244 °C, respectively. The mantle source(s) was suggested to have existed at depths between 26 and 41 km which corresponds to middle and upper segments of lithospheric mantle.
- Alteration hydrothermal processes indicating pumpellyite–prehnite facies conditions (P = 0.20–0.25 GPa (±0.006 GPa); T = 78–145 °C) at crystallization depths of ca. 6 to 8 km.
- As per geochemical and isotopic data of key incompatible trace elements the magmas which gave birth to analyzed dolerite likely separated from the garnet bearing mantle plume. Such melt(s) later mixed with the magmas generated at shallower mantle levels where residual spinel prevailed.
- Given the complexity of the petrogenetic processes and geotectonic environments of the origin of the investigated subvolcanic rocks, as well as the geodynamic concepts associated with them, two models are proposed: (1) analyzed dolerite was formed through processes of low-grade partial melting (11–13%) of the (a) subduction-modified oceanic lithosphere and/or subducted/recycled sediments beneath a metasomatically altered active mantle wedge and (b) lithospheric mantle modified by the crustal component during the melting of the lower crustal material (Figures 7a, 8 and 13a2).This model supports the geodynamic concept of Middle Triassic north-directed active subduction of the Paleotethys beneath the Laurasian active continental margin, which is accompanied by the formation of the pericontinental volcanic arc. In the arc marginal area, within the Adria Plate, an extension and back-arc rifting of the intracontinental lithosphere took place, which was followed by the emergence of locally aborted rift systems and subsidence of the platform (Figures 13a1, a2). (2) The investigated calc-alkaline rocks were generated by low-grade partial melting of the subduction-modified heterogeneous hydrated lithospheric (subcontinental) mantle (Figure 13b2), which was contaminated and/or metasomatized during earlier Variscan subduction events when local geotherms were raised due to the passive upwelling of asthenospheric mantle along the rifted margins (Figure 13b1). This model does not include an impact of a contemporaneous active subduction. Instead, it places the studied dolerite in the area of the Greater Adria where Middle Triassic extension of the High Karst nappe unit resulted with an ephemeral syn-rift volcanic phase and formation of the Dinaridic aborted rift system. Further platform subsidence ensued coupled with emergence of the local sedimentation basins (Figures 13b1, b2). Taken together, the results of this study are in favor of the second model.
Conflicts of interest
Authors have no conflict of interest to declare.
Acknowledgements
This work was supported by the Croatian Science Foundation under the project (IP-2019-04-3824). Kevin Byerly and Giovanni Zanoni are thanked for proofreading the manuscript. Critical comments and constructive reviews by two anonymous reviewers, as well as the editorial handling by Michel Faure, contributed significantly to the manuscript quality.