Version française abrégée
En référence aux travaux théoriques sur la dynamique des interactions entre enemis et victimes [1,2] et aux travaux montrant l'importance des parasites comme force évolutive pour les espèces libres [3–6], nous explorons deux hypothèses possibles sur les facteurs écologiques agissant sur l'évolution de la charge hémocytaire chez les insectes. La première hypothèse suppose que ce caractère évolue en réponse aux pressions de sélection parasitaire, qui comportent trois composantes, la diversité génétique parasitaire, l'importance des risques infectieux et leur stabilité spatio-temporelle. La seconde hypothèse suppose que cette évolution n'est pas due aux parasites eux seuls, mais à l'ensemble des facteurs de stress environnemental via une sélection pléiotrope sur les taux métaboliques [28,29]. Ces hypothèses sont testées provisoirement à l'aide de données sur les charges hémocytaires, la résistance aux parasitoı̈des et l'écologie de six espèces de Drosophila, du sous-groupe melanogaster. Les données écologiques sont traduites en termes, de stabilité, de diversité et de richesse des habitats. Il apparaı̂t tout d'abord que l'ensemble de ces variables évoluent rapidement et sont peu corrélées à la phylogénie. La charge hémocytaire est significativement corrélée à l'index parasitaire, mais la corrélation avec l'index de stress environmental est non significative. Cette corrélation entre caractères populationnels et caractéristiques spécifiques suggère que la sélection opère au moins en partie au niveau spécifique, du fait du mélange des caractères entre populations. Ceci constitue cependant plus un travail méthodologique qu'un réel test d'hypothèse, les résultats étant basés sur trop peu de données pour nous permettre de conclure défninitivement. D'autres espèces de Drosophila, dont l'écologie est suffisamment connue, devraient être caractérisées pour leur charge hémocytaire afin de tester cette hypothèse.
1 Introduction
Enemy-induced mortality in insect populations varies depending on the habitat, among other factors. An important number of theoretical and empirical studies in insects and other organisms have demonstrated these relationships between parasite or predator prevalence and productivity heterogeneity or stability of the habitat [1,2], but the evolutionary responses of the hosts have rarely been considered. However, parasites and pathogens are known to affect many aspects of the ecology and behaviour of their hosts [3]. Because of the parasite's impact, hosts should invest in defence and, because this investment is at the expense of other tasks such as reproduction, it is emphasized that parasites are major evolutionary driving forces [4,5].
To our knowledge, the only investigations carried out so far concern the coevolutionary responses to habitat productivity. These questions were addressed theoretically in prey-predator systems [6] and empirically in microorganisms [7]. Theoretical models predict that productivity promotes the investment in resistance and virulence, to the detriment of other competitive abilities.
Other investigations, mostly in birds, have shown that related host species exhibit differences in their investment in immune function or immune defense depending on the risks to parasite exposure [8,9]. Birds living in the tropics, where the diversity of parasites they should face is high, have enlarged spleens [8]. It has also been shown that bird species harbouring high parasitic nematode species richness have high investment in immune organs, i.e. spleen size [9].
Comparative studies on invertebrate hosts are rare, despite their major interest. Invertebrates only possess a constitutive immune system involving both cellular and humoral responses but no adaptive immune system (such as antibodies and lymphocytes of vertebrates). For this reason, invertebrates are good models to investigate investment in immune defense as there is no confounding effect of the adaptive responses.
Insect immunocompetence is composed of specific [10] and non-specific [11] factors. Understanding the evolution of target-specific mechanisms will require the knowledge of the abundance or at least the presence–absence of the targeted species. Other mechanisms of resistance, less parasite-specific such as the hemocyte concentration of the insect host, will depend on more general patterns of abundance of a large set of target species. Hemocyte load is an important determinant of the ability to mount a cellular encapsulation response against a wide range of macroparasites in insects. It has proven to correlate with encapsulation ability of Asobara parasitoids parasitoids in Drosophila [12,13], and in the house-cricket, to the ability to encapsulate synthetic beads [14].
Another advantage to consider non-specific mechanisms is the higher impact on the energy balance they should have, compared to ‘key-locker-like’ mechanisms that characterize most target-specific interactions. The former are determined by single molecules, whereas the latter are usually characterized by high energy input.
Here, we investigate the reasons of variability in concentrations of circulating hemocytes in six species of the melanogaster subgroup. Our prediction is that the concentration in circulating hemocytes depends on habitat productivity, stability and diversity of the species considered. These characteristics are species level traits, that combine species averages and heterogeneities in habitat in space and time. Two hypotheses are considered to link habitat and selection on hemocyte load. The first supposes that parasites are the major selective forces and parasite resistance is the trait selected (parasite selection hypothesis), the second assumes that evolution of hemocyte load is driven by pleiotropic selection on metabolic rate and stress resistance (metabolic rate hypothesis). In order to avoid phylogenetic confounding effects, we used the phylogenetic information for the melanogaster subgroup [15].
2 Model and methods
2.1 Data on hosts
Six species of Drosophila belonging to the melanogaster subgroup are considered in this study (Fig. 1A). Phylogenetic informations were obtained from Lachaise et al. [20] and branch lengths, were considered equal for the analysis (Fig. 1A). Data on ecology and distributions of Drosophila species, summarized in Table 1, were obtained from published data [16–19]. The geographical distributions of the six species are given in Fig. 1B.
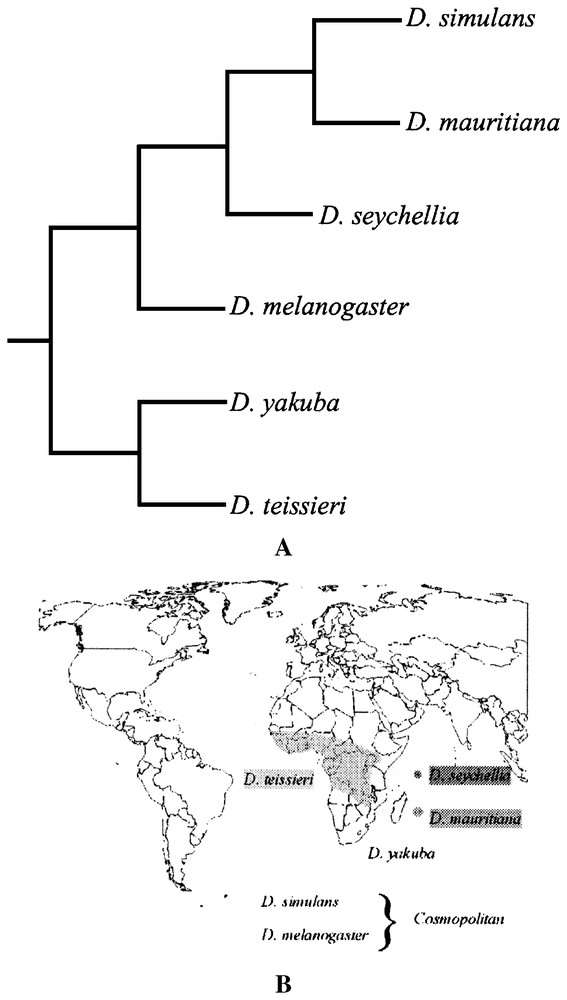
A. Geographic distribution of the melanogaster subgroup species [18]. B. Phylogenetic relationships in the melanogaster species subgroup.
Ecological characteristics of six species of the melanogaster subgroup, predicted indexes of selection and total hemocyte load (THC). Variables: A, habitat; B, domestication; C, specialisation; D, isolation; E, endemism; PSI, parasite selection index; SSI, stress selection index
Variables | A | B | C | D | E | PSI | SSI | THC [12] |
D. melanogaster | Open [18] | Domestic [17] | Specialist [17,18] | Continent [18] | Cosmopolite [18] | 2 | 4 | 7937 |
D. seychellia | Open [18] | Wild [18] | Specialist [18] | Island [18] | Endemic [18] | 1 | 1 | 5030 |
D. mauritiana | Forest [19] | Domestic [17] | Generalist [18] | Island [18] | Endemic [18] | 2 | 2 | 7996 |
D. simulans | Open [18] | Wild [18] | Generalist [17,18] | Continent [18] | Cosmopolite [18] | 4 | 4 | 43 279 |
D. teissieri | Forest [18] | Wild [18] | Specialist [17,18] | Continent [18] | Endemic [18] | 3 | 1 | 33 750 |
D. yakuba | Open [18] | Wild [18] | Specialist [17,18] | Continent [18] | Endemic [18] | 2 | 2 | 14 405 |
Data on hemocyte concentrations and immune resistance (percentage of encapsulated parasitic eggs) of the different species were obtained from Eslin and Prévost [12]. All host larvae were maintained in the same conditions at 20 °C and 13 h light: 11 h dark, during the experiment and for several generations of rearing. Hemocyte concentrations were estimated with Thoma hemocytometer from hemolymph of 6-day-old, non-parasitized larvae [12].
2.2 Score attribution and indexes of selection
In Table 1, the species are assigned to five binary variables representing habitat characteristics according to published biogeography data [16–19]. Each habitat characteristic is attibuted a score 0 or 1, depending on the hypothesis. Score attibution is described in Fig. 2A for parasite-resistance hypothesis and Fig. 2B for environmental-stress hypothesis. Score attribution was performed by linking habitat characteristics of each species to variables representing the different components of the selection forces. The indexes of selection of each species are then obtained by summing the scores obtained for each variable (Table 1). For any of the two models, we did not find any a priori argument to apply different weight to each habitat characteristic and we attributed the same weight to each binary variable.

Models of selection. A. Parasite selection hypothesis. Relationship between ecological characteristics and predicted selection for parasite resistance in Drosophila. Parasite selection index was computed by adding the species ecological characteristics coded by 0 or 1 (between brackets). B. Metabolic rate selection hypothesis. Relationship between ecological characteristics and predicted selection for stress tolerance and reduced metabolic rate in Drosophila. Geographic distribution of the melanogaster subgroup species [18].
2.2.1 The parasite selection index
As described in Fig. 2A, we considered three components of selection: (i) the mean prevalence of the parasites, (ii) its quantitative heterogeneity in space and time, and (iii) its genetic heterogeneity.
(i) It is predicted that parasite prevalence increases selection on immunocompetence traits [11].
(ii) It is predicted that variation of parasite prevalence in space and time reduces the selection pressures, as expected from basic theory of selection across time [20] or space [21]. The fitnesses of genotypes are given by the geometric mean across environments or time, which makes them closer to the population mean fitness compared to a situation with a single-patch constant selection, thereby reducing selective pressures. Holt and Hochberg [22] also explained the reduced selection for resistance against biocontrol compared to chemical control, by arguing that spatial structuring and dynamical instability can lead to situations where strong mortality effect is de-coupled from strong selection.
(iii) It is expected that selection for hemocyte load (non-specific immune factor) will increase with the genetic heterogeneity of the parasite community. All things (prevalences) being equal, the investment in immunity should be higher when the parasite community is more diversified. It takes more energy to develop a battery of specific mechanisms providing resistance against a wide range of enemies than to specialize against a low number of specific enemies. Another way around is to increase the investment in non-specific mechanisms of resistance to the detriment of multiple specific resistance mechanisms. Selection through parasite diversity should therefore positively affect mechanisms such as hemocyte load that helps to resist a wide range of parasites.
In Table 1, binary variables of habitat characteristics of the species considered were linked to the three components of selection, as explained in Fig. 2A according to the following rationale.
A – Habitat. Forest (score=1) vs open habitats (score=0). Adult flies are known to be highly dependent on water resources and to have a low tolerance to desiccation [16]. Habitat productivity should be higher in the forest, leading to greater parasitism rate [6] (hypothesis (i) on prevalence). Year-to-year variation of drosophilid abundance is indeed correlated with humidity [16]. Resources of the evergreen forest (as with fig trees) should also be more stable and predictable than resources from savannah or other open habitats leading to increased prevalence homogeneity [23] (hypothesis (ii) on prevalence homogeneity). Open habitats are also expected to be more fragmented in their resource. Habitat fragmentation is generally correlated with lower parasitism rate and parasitoid species loss [24].
B – Domestication. Wild (score=1) vs domestic (score=0). Hosts living in wild habitats should face a more diverse array of parasites. The decrease in parasite species diversity (hypothesis (iii)), particularly parasitoid diversity, is likely to occur in urban areas, as it occurs for their drosophilid hosts [17].
C – Isolation. Continent (score=1) vs island (score=0). Hosts living on continents should be more at risk of infection than hosts living on islands. Parasitic pressures are known to be less important and parasite less genetically diverse on islands and it is emphasized that insular hosts reduce their investment in immune defence [25,26] (hypothesis (i) and (iii) parasite prevalence and genetic diversity).
D – Distribution. Cosmopolitan (score=1) vs endemic (score=0). Cosmopolitan hosts (D. simulans and D. melanogaster) are attacked by a wider range of parasites and pathogens than endemic species (hypothesis (iii)).
E – Specialisation. Generalist (score=1) vs specialist (score=0). Generalist hosts like D. simulans, D. melanogaster and D. mauritiana, which are able to produce large populations in all habitats [17,19], are submitted to a more diversified range of parasites and pathogens. Because of the increased genetic diversity of the parasites, generalists should invest more in traits related to non-specific immunity, like hemocyte load, than species specialized on a single habitat (D. seychellia, D. yakuba or D. teissieri; [18]) (hypothesis (iii)).
2.2.2 The stress selection index
It is based on the observation of Fellowes and Godfray [27] and Fellowes et al. [28] that parasitoid resistance and metabolic rate are negatively correlated in the same species we analysed. The relationship we observed between hemocyte load and habitat could therefore be driven by indirect selection on metabolic rate instead of direct selection on parasite resistance. Since higher metabolic rate is advantageous in non-stressful conditions [29], habitat productivity and predictability should select for a higher metabolic rate and due to pleiotropy, for reduced hemocyte load [28]. Inversely, environemental stress should correlate to higher hemocyte load. The same procedure as for parasite selection hypothesis was applied to compute an index of stress selection on hemocyte load (Fig. 2B, Tables 1 and 2). We considered three variables: (i) habitat productivity, (ii) habitat instability, and (iii) habitat heterogeneity. The model is described in Fig. 2B. Fig. 2B describes the habitat characteristics considered to be stressfull and their link to the ecological variables (A, B, C, D, E) obtained from the literature.
Correlations between species ecological indexes, total hemocyte load (THC) and encapsulation rate of Azobara tabida eggs (ER). Ecological indexes are described in Fig. 2 and Table 1
Variable 1 | Variable 2 | Regular correlation | Independent contrasts | ||
r | p-value | r | p-value | ||
ER | THC | 0.947 | 0.004 | 0.956 | 0.011 |
Parasite selection index | THC | 0.888 | 0.018 | 0.872 | 0.001 |
″ | ER | 0.948 | 0.004 | 0.975 | 0.005 |
Stress selection index | THC | 0.087 | NS | 0.034 | NS |
″ | ER | 0.179 | NS | 0.305 | NS |
Computation of the theoretical indexes of selection are described in Table 1 depending on the hypothesis. For variables C, D, and E, the effect had the same direction, whereas for A and B the direction was opposed. Since the two hypotheses were not tested against each other but independently, variables C, D, and E were not removed from the analysis.
2.3 Statistical analysis
The method of independent contrasts is a conservative test for correlation between traits in related species, but regular correlation is still valid and more powerful when phylogenetic inertia is absent [30]. In independant contrasts, ancestor trait values, on which some of the contrasts are made, are reconstructed from present species. This reconstruction is based on the hypothesis of phylogenetic inertia. But when the trait evolves rapidly, it increases dramatically the error term in the analysis [30]. To test for phylogenetic inertia on encapsulation rate, hemocyte counts and infection risk, we evaluated the correlation between pairwise phylogenetic distances and the pairwise differences in the trait. If pairwise differences are not phylogenetically independent, this test will reject the null hypothesis more often than by chance. This can be seen as an advantage in our case, since we needed to be confident in the null hypothesis of the phylogenetic independence to allow the use of regular correlation instead of independent contrasts.
Both methods, independent contrasts and regular correlation, were used to test the relationship between hemocyte load and infection risk. Independent contrasts were calculated using the CAIC program [36]. In order to verify that contrasts were properly standardized, we performed a regression of the absolute values of standardized contrasts versus their standard deviations [31].
3 Results and discussion
Encapsulation rate, hemocyte load and infection risk did not significantly depend on the phylogeny, i.e. there was no significant correlation between pairwise phylogenetic distances and difference in hemocyte counts in any of the traits (r=0.00±0.25 for encapsulation rate, r=+0.06±0.25 for hemocyte count and r=−0.14±0.25 for infection risk). This could justify the use of regular correlation instead of independent contrasts to test for the relation between traits and environmental variables [30]. However, independent contrasts are still valid, although less powerful.
Both methods showed the same strong and significant effect of parasite selection index on hemocyte concentration (r=0.888, p=0.018 for regular correlation and r=0.9239, p=0.0022 for independent contrasts) (Fig. 3, Table 2). However, correlation with the index of environmental stress was not significant (r=0.037 for regular correlation and r=0.019, p>0.5 for independant contrasts) (Table 2).

Correlation between hemocyte concentration and parasite selection index in the melanogaster subgroup.
Understanding the factors driving coevolutionary interactions should be very useful to explain the variations observed among species, geographic localities or habitats. Models predicting the evolution of resistance to predators and parasitoids along productivity gradients provide a significant insight into this respect [6], but have been tested on few occasions [7]. In addition to productivity [5], immune functions in insects might also evolve in response to parasite diversity and prevalence stability. We developed this hypothesis that investment of a species in non-specific immunity should correlate with its habitat productivity, predictability and diversity and started to test it with data available on hemocyte load of Drosophila species and their ecology. Our results, although based on few host species, seemed to support this view. As expected, hemocyte load was correlated with an index of parasite selection combining habitat productivity, predictability and diversity of Drosophila species of the melanogaster subgroup. Another hypothesis that it is driven by metabolic rate was proposed. We calculated an index of environmental stress that should correlate positively with hemocyte load under this hypothesis of epistatic selection. It did not give any significant relationship, but the present test is not powerful enough to definitely reject this metabolic rate hypothesis.
Our results are supported by correlation estimates between a trait and some environmental variables in various species. Felsenstein [30] noted that these correlations may not be statistically valid when the trait is phylogenetically constrained. Several methods have been developed to take into account phylogeny in the statistical analyses on species trait values. Using the technique of independant constrasts [30], Fellowes and Godfray [27] confirmed the correlation between encapsulation rate and hemocyte load in the melanogaster subgroup obtained by [12]. This technique, although commonly used and always statistically valid, may not be necessary in this case, since there does not seem to be any phylogenetic inertia on these traits, as suggested by the absence of significant correlation between phylogenetic distance and trait differences.
Several theoretical and comparative arguments emphasize that the maintenance of performing immune defenses are dependent on parasite pressures. Immunity is costly [5,32,33]. The investment in immune defense in Drosophila should be paid at the expense of other tasks. Reduced fecundity or decreased longevity are such life traits that may be affected by this investment. Kraaijeveld and Godfray [33] and Fellowes et al. [28] selected flies for increased resistance against Asobara tabida or Leptopilina boulardi, respectively. These selected flies invariably showed reduced competitive abilities and reduced feeding rate in conditions of high larval density. Whether this cost is related to hemocyte load is not known, although Kraaijeveld et al. [13] noted a developmental association between hematopoietic organs and head musculature. This reinforces the hypothesis that the production of hemocytes is under balancing selection depending on the infection risk.
The correlation observed between species-level selective factors and hemocyte load measured on single populations would suggest that selection on this trait acts at least partly at the species level. Even if some traits are measured on single populations, there seems however to be enough genetic homogeneity within species for correlations to be observed. This species-level ecological effect is not inconsistent with the geographic mosaic theory of coevolution that states that the evolution of traits of interactions is driven by geographic mosaics of selection and trait remixing between populations. In this case, trait remixing between populations would be more powerful than heterogenetity of selection between populations to drive the evolution of the trait. The present results are however based on a small number of species and they are not robust enough to be confident on the effect of species-level ecology on the immune competence. This analysis is more a methodological work than an empirical evidence for the existence of such a relationship.
Other Drosophila species are known for their resource and habitat specialization [17,23], which should allow to measure their hemocyte counts and challenge our theoretical predictions with more powerful tests. To quantify the geographic mosaic of selection within one species, generalist species like D. simulans confronted to different habitats should be a good model to investigate by studying the correlation between infection rates and hemocyte load along a longitudinal transect accross habitats. The following step would be to distinguish between the different factors of variation (i.e. habitat productivity, stability, and diversity), and to test and quantify their relative impact within and between species.
Despite the ecological success of many groups of invertebrates, most studies on ecological immunity focus on vertebrates [5]. This study in Drosophila suggests a correlation between species-level ecological characteristics and investment in immune function in single populations. This indicates that although the evolution of interaction can vary geographically within species due to selection mosaics, species-level ecological characteristics like geographical range or ecological heterogeneity can also affect directly the investment in immunity: due to trait remixing between populations, species are still meaningful genetically homogenous entities. Selection mosaics and trait remixing are the two components of the evolution of interactions emphasized in the geographic mosaic theory of coevolution. These components could be quantified in further studies combining within and between species trait comparisons.
Acknowledgements
The authors are grateful to Dr D. Lachaise, J. David for their conceptual inputs, ecological knowledges on Drosophila and to G. Doury and A. Adams for their comments and corrections on the manuscripts to the CNRS GDR 2153 for financial and scientific support.