Abbreviations
GPX; glutathione peroxidase
ROS; reactive oxygen species
SOD; superoxide dismutase
1 Introduction
Except for anaerobic microorganisms, all living organisms require molecular oxygen as an electron acceptor for efficient production of energy. However, oxygen is a strong oxidant, and it is impossible to avoid secondary oxidations not involved in physiological metabolism. These random oxidations would have deleterious consequences if they were not neutralised by an efficient antioxidant system. From this theoretical evidence, biophysicists introduced the concept of ‘oxidative stress’ to describe a situation occurring in aerobes when the undesired secondary oxidations induced by oxygen and its derivatives are not efficiently neutralised, and lead to abnormal metabolism, loss of physiological function, disease, and potentially death [1,2]. For many years, biophysicists and theoretical chemists have communicated about oxidative stress and its biological consequences using an abstruse language that did not really appeal to most biologists and physicians. For this reason, the latter did not pay much attention to the growing number of publications focused on this subject, and eventually imagined oxidative stress more as a theoretical model unusable for applied biology and medicine than a biological reality that could have interesting applications. Actually, those who showed the most interest in this concept where people involved in the cosmetic industry. Considering the low level of interest usually shown by the scientific and medical community for cosmetology, it is not surprising that most physicians continued until very recently to perceive oxidative stress as a theoretical model rather than a biological reality that could improve our knowledge on biochemical mechanisms and help finding the search for new treatments for human diseases.
The aim of this review is to convince biochemists, biologists and physicians that oxidative stress is more than a theoretical model or a nice story used by the cosmetic industry to drive business thanks to the fear people have of ageing.
2 What is oxidative stress?
Oxidative stress corresponds to an imbalance between the rate of oxidant production and that of their degradation [2]. Aerobic organisms such as vertebrates and man in particular produce their energy from the oxidation of organic substrates by molecular oxygen. The complete four-electron reduction of molecular oxygen occurs within mitochondria and produces water, at the end of the respiratory chain. Sometimes molecular oxygen is partly reduced instead of the proteins of the respiratory chain, and superoxide and various reactive oxidant intermediates are produced, leading to secondary oxidations [3]. Besides these physiological oxidations and their unavoidable secondary reactions, many substances contained in food are either oxidants by themselves or oxidant precursors [4,5]. Other oxidants come from the environment: (i) air pollutants and oxidant contained in tobacco and fire smoke react with molecules of the skin surface, and some of them penetrate into the skin and reach the circulation [6,7]; (ii) UV radiation from the sun are absorbed by cutaneous chromophores and induce the formation of a variety of oxidants [8–10]. Finally, although the organism adapts to any unstable situation by preventing undesirable reactions and repairing damaged molecules and tissues, the very few undesirable reactions that escape the prevention and repair systems accumulate little by little, and will invariably be deleterious after a long period of time [11,12], thus confirming the free radical theory of ageing developed by Denham Harman [13]. All these conditions lead to the production of various oxidants, and if the organism fails to neutralise them, these oxidants accumulate and react with a variety of biomolecules, creating an undesirable situation known as oxidative stress [2].
3 Which molecules mediate oxidative stress?
Two classes of molecules mediate most of the reactions leading to oxidative stress: free radicals and reactive oxygen species (ROS) (Table 1, Fig. 1). Free radicals are molecules possessing at least one unpaired electron; for this reason, they are unstable and promote electron transfers, i.e., oxidations and reductions. Lipid radicals are formed by hydrogen abstraction, a process elicited by UV irradiation or the hydroxyl radical (•OH) [14,15]. The latter, as well as other reactive radicals, belong to the class of ROS. These unstable intermediates derive from molecular oxygen (•O2• or 3O2), a biradical. In the case of molecular oxygen, the triplet (biradical) state is more stable than the singlet one (non radical, with a double bond); thus singlet oxygen (1O2) is a ROS. It can be produced by several biochemical oxidations involving peroxidases and lipoxygenases, by reactions between various ROS or in the presence of light, oxygen and a photosensitizer such as porphyrins, as it is the case in congenital erythropoietic porphyria [1,16,17]:
Molecules mediating oxidative stress
Name | Structure | Main reactions |
Superoxide | •O–O− | Catalysis of Haber–Weiß reaction by recycling Fe2+ and Cu+ ions; formation of hydrogen peroxide or peroxynitrite |
Hydrogen peroxide | HO–OH | Formation of hydroxyl radical; enzyme inactivation; oxidation of biomolecules |
Hydroxyl radical | •OH | Hydrogen abstraction; production of free radicals and lipid peroxides; oxidation of thiols |
Ozone | −OO+O | Oxidation of all kinds of biomolecules, especially those containing double bonds; formation of ozonides and cytotoxic aldehydes |
Singlet oxygen | OO | Reaction with double bonds, formation of peroxides; decomposition of amino acids and nucleotides |
Nitric oxide | •NO | Formation of peroxynitrite; reaction with other radicals |
Peroxynitrite | ONOO− | Formation of hydroxyl radical; oxidation of thiols and aromatic groups; conversion of xanthine dehydrogenase to xanthine oxidase; oxidation of biomolecules |
Hypochlorite | ClO− | Oxidation of amino and sulphur-containing groups; formation of chlorine |
Radical | R• | Hydrogen abstraction; formation of peroxyl radicals and other radicals; decomposition of lipids and other biomolecules |
Peroxyl radical | ROO• | Hydrogen abstraction; formation of radicals; decomposition of lipids and other biomolecules |
Hydroperoxide | ROOH | Oxidation of biomolecules; disruption of biological membranes |
Copper and iron ions | Cu2+, Fe3+ | Formation of hydroxyl radical by Fenton and Haber–Weiß reactions |

Overview of free radical and ROS formation and elimination. The main pathway of free radical and ROS formation starts with the partial reduction of molecular oxygen to superoxide by oxidases or the reduced or semiquinone forms of ubiquinol (coenzyme Q). The hydrogen peroxide produced by the dismutation of superoxide can decompose in the presence of transition metals to give rise to hydroxyl radical, an extremely instable oxidant. The latter can initiate lipid peroxidation in the presence of oxygen. Another strong oxidant, peroxynitrite, is formed by reaction between superoxide and nitric oxide, an intercellular messenger produced from arginine, oxygen and NADPH. It thus appears that the more important antioxidants are scavengers of superoxide and hydrogen peroxide. Since the dismutation of superoxide produces hydrogen peroxide, catalase and GPX, the enzymes that remove hydrogen peroxide, should be associated to SOD. Catalase does not require a cofactor, since it catalyses a dismutation (2 H2O2→2 H2O+O2), but GPX oxidises glutathione, which is then reduced by NADPH, and the latter is finally regenerated by one of the pathways of glucose oxidation; thus glucose appears as a key component of the antioxidant reaction cascade. Abbreviations: 6PGδL, 6-phosphoglucono-δ-lactone; A, an oxidised substrate; AH2, a reduced substrate; Arg, arginine; ASC, ascorbate; ASC•, semi-dehydroascorbate; Cat, catalase; Cit, citrulline; DHA, dehydroascorbate; G6P, glucose 6-phosphate; GSH, reduced glutathione; GSSG, oxidised glutathione; GSR, glutathione reductase; HK, hexokinase; LH, a lipid, L•, a lipid radical; LOH, an hydroxylated lipid; LOO•, a lipid peroxyradical; LOOH, a lipid hydroperoxide; MPO, myeloperoxidase; NR, NAD(P)H reductase; PS, photosensitizer; Q, ubiquinone; QH•, ubisemiquinone; QH2, ubiquinol; TOC, α-tocopherol, TOC•, α-tocopheryl radical.
Other ROS derive from the secondary reduction of 3O2 in the inner membrane of mitochondria (mainly from ubiquinol oxidation), where oxygen receives one electron in the place of a metalloprotein of the respiratory chain [3,18]. The superoxide radical anion thus formed (•O2−) can then dismutate into oxygen (3O2) and hydrogen peroxide (H2O2), either spontaneously at acidic pH or in a reaction catalysed by superoxide dismutase (SOD) [1]:
Superoxide and hydrogen peroxide can react with transition metals such as iron or copper to form the strong oxidant hydroxyl radical (•OH) [1,19,20]:
Superoxide and hydrogen peroxide are also enzymatically formed by NADPH oxidase in activated neutrophils or macrophages during inflammatory processes [21,22]:
Generally speaking, all endogenous oxidases – enzymes that transfer two electrons from a substrate (AH2) to molecular oxygen – produce superoxide and hydrogen peroxide [1]:
Myeloperoxidase, an enzyme present in neutrophils, but not in macrophages, can produce hypochlorous acid (HOCl) by oxidising chloride ions with hydrogen peroxide [1,23]:
Hypochlorous acid (or its conjugated base hypochlorite, depending of the pH), is a powerful oxidant towards various biomolecules, especially amino groups; furthermore, in acidic pH it readily decomposes to liberate the highly toxic chlorine gas Cl2 [1,24]:
As mentioned above, hydrogen peroxide can react with hypochlorite to form singlet oxygen. Under physiological conditions, 1–2% of the consumed oxygen is converted to ROS. Nitric oxide (•NO), an intercellular messenger, is produced from oxygen by various nitric oxide synthases [1,25]:
4 Endogenous defences against oxidative stress
Strict aerobic organisms cannot live in the presence of oxygen, because they have insufficient defences against the multiple secondary reactions induced by oxygen. All other organisms which can or must live in the presence of oxygen possess an efficient battery of antioxidant defences able to trap reactive intermediates before they have time to oxidise biomolecules or reduce those which have been oxidised (Table 2, Fig. 1) [31–33]. Due to the great variety of reactive intermediates that must be neutralised, as well as the variety of oxidised biomolecules which must be reduced, there are a lot of different antioxidants; moreover, there is a need for antioxidants for both hydrophilic (cytosol, extracellular fluids) and lipophilic (membranes, lipids) phases [1,31–36].
Endogenous antioxidants
Antioxidant | Phase | Action |
Superoxide dismutases (SOD) | Hydrophilic | Dismutation of O2− into H2O2 and O2 |
Catalase | Hydrophilic | Dismutation of H2O2 into H2O and O2 |
Glutathione peroxidases (GPX) | Hydrophilic or lipophilic | Reduction of R–OOH into R–OH |
Glutathione reductase (GSR) | Hydrophilic | Reduction of oxidised glutathione |
Glutathione-S-transferases (GST) | Hydrophilic | Conjugation of R–OOH to GSH (→ GS–OR) |
Metallothioneins | Hydrophilic | Binding to transition metals (= neutralisation) |
Thioredoxins | Hydrophilic | Reduction of R–S–S–R into R–SH |
Glutathione | Hydrophilic | Reduction of R–S–S–R into R–SH |
Free radical scavenger | ||
Cofactor of GPX and GST | ||
Ubiquinol | Lipophilic | Free radical scavenger (prevents LPO) |
(Dihydro)lipoic acid | Amphiphilic | ROS scavenger |
Increases antioxidant and phase II enzymes | ||
Ascorbic acid (vitamin C) | Hydrophilic | Free radical scavenger |
Recycles tocopherols (vitamin E) | ||
Maintains enzymes in their reduced state | ||
Retinoids (vit. A) and carotenoids | Lipophilic | Free radical scavengers |
Singlet oxygen (1O2) quencher | ||
Tocopherols (vitamin E) | Lipophilic | Free radical scavenger (prevents LPO) |
Increases selenium absorption | ||
Selenium | Amphiphilic | Constituent of GPX and thioredoxins |
The most efficient antioxidants are enzymes that catalyse the reduction of ROS: superoxide dismutase (SOD) catalyses the dismutation of superoxide (•O2−) into hydrogen peroxide (H2O2) and oxygen (3O2), catalase catalyses hydrogen peroxide (H2O2) dismutation into water (H2O) and oxygen (3O2), glutathione peroxidases (GPX) reduce both hydrogen (H2O2) and organic (R-OOH) hydroperoxides. The oxidised glutathione cofactor (GS–SG) is then reduced (2 GSH) by glutathione reductase (GSR). Various GPX isoforms exist, which are specific for hydrophilic or lipophilic phases. Metallothioneins are small proteins with several cysteine residues which bind transition metal ions: this can both detoxify metals and avoid them catalysing the Haber–Weiß and Fenton reactions that lead to the production of the hydroxyl radical (OH). The tripeptide glutathione and the selenoprotein thioredoxin are efficient in reducing disulphur bridges (R–S–S–R′) into thiols (R–SH+R′–SH), as well as reducing thiyl radicals (R–S•); the whole system involving thioredoxin, thioredoxin peroxidase and thioredoxin reductase is known as peroxiredoxin. Besides being an antioxidant by itself, glutathione is also the cofactor for GPX and GSR. Ubiquinol (coenzyme Q, QH2), plays an essential role in the mitochondrial electron-transport chain in creating a proton gradient between both sides of inner mitochondrial membrane. Due to its two-step oxidation via a radical intermediate:
5 Oxidative stress is involved in many pathologies
An oxidative stress is often associated to all kinds of diseases, although it is not always easy to determine whether it is a cause or a consequence of the observed condition. A selection of pathologies for which the mechanism of oxidative stress is well documented is described below.
5.1 Ischaemia-reperfusion
The mechanism of ischaemia and tissue injury during reperfusion has been extensively studied, especially in the heart [47]; it is thus a good example of the involvement of oxidative stress in a frequent pathology.
During ischaemia, blood glucose falls, glycolysis and oxidative phosphorylation rates decrease, and subsequently ATP stores are consumed. The two consequences of ATP depletion are (i) accumulation of AMP [48] due to the following reactions:
In summary, during ischaemia, (i) the tissues accumulate a substrate for xanthine oxidase, (ii) the latter is formed by the alteration of xanthine dehydrogenase, and (iii) the tissue defences against oxidative stress are decreased. Thus, as soon as oxygen is reperfused, all these conditions act synergistically to produce high levels of ROS in the tissues that have undergone ischaemia (Fig. 2).
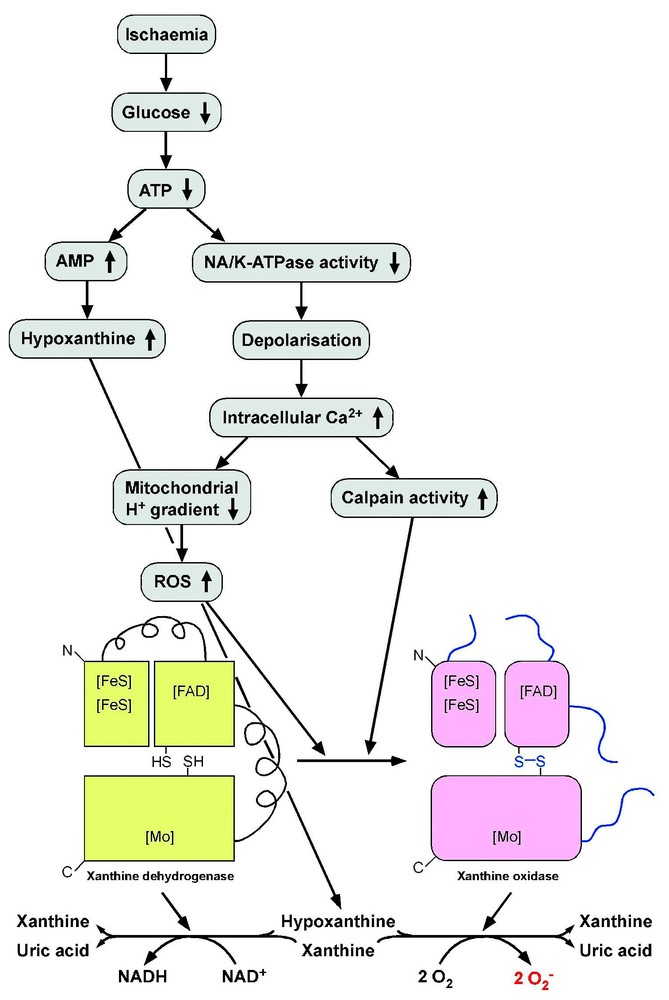
Mechanism of ROS production during reperfusion after ischaemia. During ischaemia, blood glucose falls down, leading to ATP depletion. As a consequence, hypoxanthine, a substrate for xanthine dehydrogenase and xanthine oxidase, accumulates in the cells. Due to the lack of ATP, the activity of ATP-dependent pumps such as Na+/K+-ATPase decreases, which leads to depolarisation and a massive influx of Ca2+ ions, promoting the loss of mitochondrial proton gradient and then the production of superoxide and other ROS, as well as the induction of calcium-dependent proteases such as calpains. The latter, as well as ROS, convert xanthine dehydrogenase to its oxidase form, and when oxygen is reperfusated, the high hypoxanthine concentration activates xanthine oxidase, which produces high levels of ROS.
5.2 Atherosclerosis
Many cases of myocardial infarctions and localised cerebral ischaemia are the consequence of atherosclerosis, a disease of arteries characterised by a local thickening of the inner coat of the vessels. It is now widely accepted that ROS play an important role in the initiation and the development of atherosclerosis. Many genetic and environmental conditions (hypercholesterolemia, diabetes, hypertension, smoking, ageing...) can lead to the peroxidation of LDL lipids. These oxidised LDL bind to special LDL receptors, called scavenger receptors, expressed by macrophages; the latter become then foam cells, i.e. lipid-laden distorted cells from macrophage or vascular origin that contribute to the formation of atheroma plaques. Activated enzymes such as xanthine oxidase, NADPH oxidase or nitric oxide synthase then produce more ROS, leading to the formation of fibrous plaques; the latter consist of fibrous cap, composed mostly of smooth muscle cells and dense connective tissue surrounding by macrophages, T lymphocytes and other smooth muscle cells [47,57–59].
Thus the initiation process can be due either to excess of fat, a defective metabolism of lipids, an infection, the presence of toxins or toxic metabolites, or an oxidative stress, then oxidative stress promotes the development of the disease.
5.3 Neurodegenerative diseases
The brain accounts for only ≈2% of body mass, but it processes 20% of basal oxygen consumption in order to support the neuronal electric activity. On the other hand, nitric oxide plays a crucial role as a biological messenger, and is produced by three kinds of nitric oxide synthases (neuronal, endothelial and inducible) [25,60]. Thus the brain produces high levels of superoxide and nitric oxide, and these molecules can react to form peroxynitrite, one of the most reactive oxygen species [61]. For these reasons, oxidative stress plays a pivotal role in neurodegenerative disorders, and we discuss below the three major neurodegenerative diseases, in which the link with oxidative stress has been extensively studied [62,63].
5.3.1 Excitotoxicity
A major consequence of ROS production in the brain is the inhibition by various ROS of glutamate uptake by astrocytes [64–66]. The latter constitute most of the cortical mass of the brain, and one of their vital roles is the efficient uptake of glutamate that is released into the extracellular space by neurones during their electric activity. A decrease in glutamate uptake by astrocytes leads to a depolarisation of neuronal plasma membranes due to excess activation on NMDA receptors; this induces a high influx of calcium ions within neurones, a condition that activates calcium-dependent enzymes such as calpains and phospholipase A2, as well as a decrease of mitochondrial activity. As discussed above, calpains convert xanthine dehydrogenase to its oxidase form, giving rise to superoxide and hydrogen peroxide, whereas activation of phospholipase A2 releases arachidonate, thus producing ROS via its metabolism to bioactive lipids, as well as a direct inhibition of glutamate uptake [67]. On the other hand, a high level of glutamate or other excitatory amino acids inhibits the uptake of cystine by brain cells; since cystine transport into brain cells is essential to supply cysteine for synthesis of the antioxidant and GPX-cofactor glutathione, this decreases the antioxidant defences of brain cells against oxidative stress; this cytotoxic mechanism elicited by excitatory amino acids is called excitotoxicity [68]. Taken together, these conditions act synergistically in different cycles to induce cell death due to the generation of an oxidative stress and intracellular calcium overload [63,69] (Fig. 3).
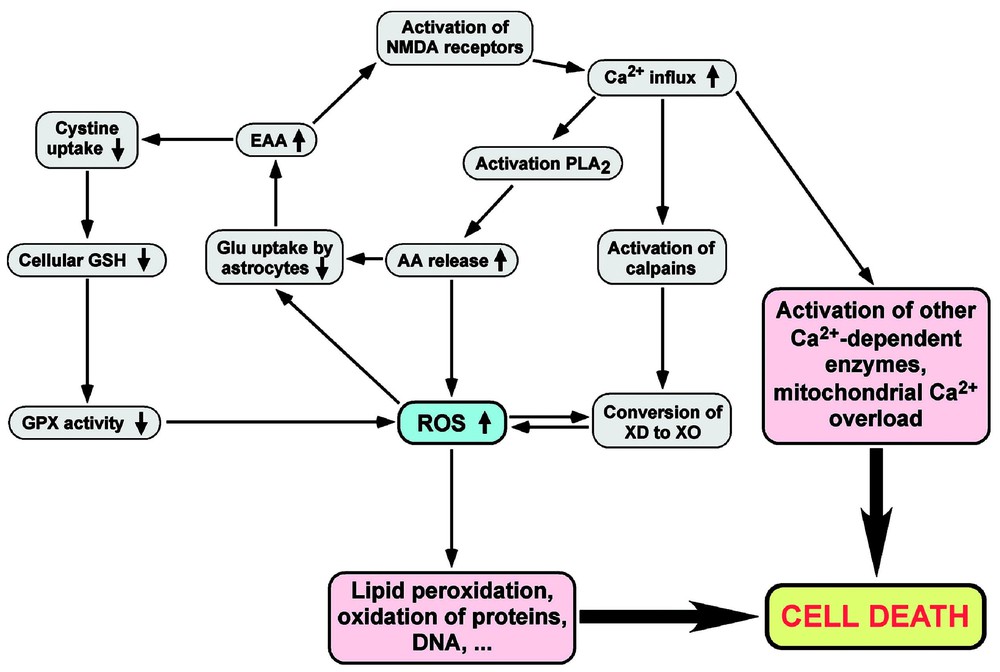
Relationships between oxidative stress, neuronal activity and cytotoxicity. The release of EAA such as Glu, excessive Ca2+ ions influx and ROS production are linked together in various cycles, leading to cell death. If Glu uptake by astrocytes is inhibited by ROS or excessive AA release, this increases the extracellular EAA concentration, which in turn decreases antioxidant defences, increases ROS production, induces the depolarisation of cellular membranes, and finally provokes cell death. Abbreviations: AA, arachidonate; EAA, excitatory amino acids; Glu, glutamate; PLA2, phospholipase A2; XD, xanthine dehydrogenase; XO, xanthine oxidase.
5.3.2 Alzheimer's disease
Alzheimer's disease (AD), one of several disorders that cause the gradual loss of brain cells, is the leading cause of dementia. Its neuropathological hallmarks are neurofibrillary tangles and senile plaques, two forms of protein aggregation, i.e., β-amyloid and hyperphosphorylated tau, respectively. Although the participation of oxidative stress as a cause of the disease is still a matter of debate, the brain of Alzheimer's patients is associated with many markers of oxidative stress, and the latter increases the severity of symptoms [62,70–72].
5.3.3 Amyotrophic lateral sclerosis
Amyotrophic lateral sclerosis (ALS) is caused by the degeneration of both upper and lower motor neurons, resulting in skeletal muscle atrophy and weakness, and culminating in respiratory insufficiency. Although the cause of ALS is still unknown, several mechanisms are implicated in the pathogenesis of motor neuron degeneration, including excitotoxicity, immune activation, mitochondrial dysfunction, protein aggregation, altered proteosomal function, and apoptosis [73–75]. These mechanisms are not mutually exclusive, but rather act in synergy to worsen the resulting cytotoxicity. 10–15% of ALS cases are inherited, among them 25–30% are due to mutations of SOD. These mutations seem to be associated with a toxic gain of function rather than a loss of function; these abnormal forms of SOD are thus believed to play a role in the generation of an oxidative stress by a mechanism that remains to be elucidated [76–78]. As for AD, several markers of oxidative damage are found in the brain of all ALS patients, confirming the role of oxidative stress in the pathology of ALS, although it is not clear whether it participates as an initiator or an amplifier of the symptoms, or both.
5.3.4 Parkinson's disease
Parkinson's disease (PD) is the most common neurodegenerative movement disorder; it is due to the degeneration of dopaminergic neurones in substantia nigra. As for ALS and AD, there are both sporadic and familial forms of the disease [62,79]. Autoxidation of dopamine, as well as its catabolism by monoamine oxidase, produce superoxide and hydrogen peroxide [80,81]. This led to the concept that the metabolism of dopamine might be responsible for the high basal levels of oxidative stress in substantia nigra. On the other hand, since the story of a student in chemistry who unwittingly produced the neurotoxin 1-methyl-4-phenyl-1,2,3,6-tetrahydropyridine (MPTP) for his own consumption, this substance has been extensively used in animal models and was shown to be taken up by astrocytes, and then oxidised by monoamine oxidase in another compound, 1-methyl-4-phenyl-pyridinium ion (MPP+), which cannot diffuse through cellular membranes, but is specifically taken up by dopaminergic neurones and produces symptoms very similar to those of PD; once in the cytoplasm, MPP+ kills the cells by interfering with the mitochondrial respiratory chain by a mechanism that increases superoxide production [82,83]. Thus dopaminergic neurones are submitted to a chronic oxidative stress and can degenerate when ROS and free radicals are not efficiently neutralised by their antioxidant defences.
For the three major neurodegenerative diseases mentioned above, a familial form has been found and could explain a part of the prevalence of the disease, although the relationships between the known genetic mutations and the development of the disease is still not clear. However, for a large part of patients suffering from one of these diseases, an important question concerns the possible involvement of environmental factors. As discussed above, the neurotoxin MPTP is an example of an exogenous substance that induces similar pathological alterations to those found in idiopathic PD, with similar clinical symptoms.
5.3.5 Parkinsonism–dementia complex
In this context, it is interesting to report a surprising observation made in Marianne Islands, especially Guam and Rota. Investigators found a possible link between an ALS-like disease and a toxic substance produced by cyanobacteria. In these islands, the diet of the indigenous Chamorro people may account for the high incidence of a neurological disease called amyotrophic lateral sclerosis/parkinsonism–dementia complex (ALS–PDC), which has symptoms of ALS, Parkinson's, or Alzheimer's disease [84,85]. The bats forage on seeds from cycad trees, which contain cyanobacteria in their roots that produce the neurotoxin β-methylamino-l-alanine (BMAA). BMMA has a similar structure to that of the excitatory aminoacid glutamate, and is an excitotoxin, causing a persistent excitation of neurons that ultimately exhausts the cells until they can no longer function. This substance was shown to be concentrated 100 000 times by the food chain from bacteria to flying foxes. Chamorro people who died of ALS–PDC had high levels of BMAA in their brain, while healthy brain tissue does not have the toxin. Thus a neurotoxin that induces excitotoxicity and oxidative stress could be responsible, at least in part, for the very high incidence of a neurodegenerative syndrome observed in a limited region under special circumstances, and similar to that of the three major neurodegenerative diseases found in the world.
ROS, as short-lived and reactive molecules, are good candidates for intracellular signalling [86–88].
5.4 Modulation of signal transduction by ROS
If the role of nitric oxide as a cellular messenger is well known, such a role for the other ROS arose only recently. Although the precise mechanism linking the binding of extracellular ligands to the intracellular generation of ROS remains to be established, the latter have been shown to modulate gene expression in response to extracellular messengers. Thus cellular transcriptional signalling by ROS in response to cytotoxic injury and inflammation is mediated mainly by activation of MAP kinases, the Rho family of small G proteins, the Src family of tyrosine kinases, Ras, and cytokines; the modulation of gene expression is then mediated by the transcription factors AP-1, ATF, and NF-kappaB [88,89]. On the other hand, specific genes are also induced during hypoxia, i.e., under conditions opposite to oxidative stress. Cellular transcriptional response on hypoxia was shown to be mediated by activation of the transcription factors HIF-1 and AP-1 [89].
5.5 Ageing
Two general theories explain intrinsic ageing: genetic theories and theories about the accumulation of cellular damages. According to the latter, the natural metabolic activity produces “metabolic waste” that the organism cannot always eliminate properly. These catabolites are oxidised molecules that can become oxidants for other ones if they are not eliminated. Indeed, many markers of oxidative stress are increased in the tissues of aged individuals [90–93]. With the wire of time, these cellular damages accumulate in the organism and become cytotoxic. This concept, already mentioned by Denham Harman in 1956 as ‘free radical theory of ageing’ [94] was confirmed by many studies which reported the involvement of oxidative stress in ageing processes [1,13,92,95,96].
6 Are reactive oxygen species always deleterious?
In the previous sections, we have discussed the inevitable formation of ROS and free radicals in normal metabolism of aerobes, the defences of the organism against them, as well as the possible deleterious consequences when the rate of their formation exceeds that of their neutralisation. But do ROS and free radicals always represent an undesirable consequence of oxygen metabolism, or do they play a beneficial role too?
For instance, the reactive oxygen or nitrogen species nitric oxide, besides being an intermediate in the formation of the strong oxidant peroxynitrite, as mentioned above, has several physiological functions: in the nervous system, it acts as a neuromodulator and plays a role in synaptic plasticity and long-term memory, whereas in the vascular system, it controls blood pressure, inhibits platelet aggregation and kills certain pathogenic microorganisms [97,98].
Many physiological reactions are oxidoreduction reactions, i.e., electrons are transferred from one molecule to another, giving rise to free radical intermediates; furthermore, many enzyme-catalysed reactions produce free radicals as intermediates, which allows decreasing the activation energy of the general reaction.
When considering a ‘useful role’ for ROS, we usually design the killing mechanism of microorganisms by neutrophils and other phagocytic cells. Phagocytosis is a complex process, requiring the activation of neutrophils or macrophages [24,99]. One of its many steps involves the production in the extracellular space of superoxide and hydrogen peroxide (see Section 3). Hydrogen peroxide crosses biological membranes easily, and is toxic to microorganisms either directly by promoting several oxidations, or indirectly by forming a more reactive species such as the hydroxyl radical or hypochlorite. The highly reactive hydroxyl radical is produced from superoxide and/or hydrogen peroxide by Haber–Weiß or Fenton reactions, whereas hypochlorite is produced by myeloperoxidase [1,23,24,100]. Thus the production of several ROS by activated neutrophils or macrophages is a defence mechanism against invading microorganisms. Since the latter are much more sensitive to ROS than human tissue, there is a ‘bactericidal window’ for which the ROS concentration is sufficient to kill bacteria without causing damage to host tissue (in normal conditions).
Eicosanoids constitute an important family of bioactive lipids, comprising prostaglandins, thromboxanes and leukotrienes. They are derived from arachidonate (C20:4) under the action of prostaglandin H synthase (cyclooxygenase) or lipoxygenases. Eicosanoids locally exert various biological roles such as the mediation of inflammation, the regulation of blood flow, ion transport across membranes, the modulation of synaptic activity or the induction of sleep [101,102].
An interesting example of the involvement of ROS in signal transduction for a physiological process is the capacitation of spermatozoa by superoxide. ROS have beneficial or detrimental effects on sperm functions depending on the nature and the concentration of the ROS involved, as well as the moment and the location of exposure. Sperm capacitation and the acrosome reaction are complex processes required for ovule fertilisation; they are regulated by signal transduction mechanisms involving G proteins, calcium ions and ROS [103,104]. In particular, low concentrations of superoxide trigger this phenomenon, whereas excessive generation of hydrogen peroxide in semen could be a cause for infertility. Conversely, removal of superoxide by superoxide dismutase prevents sperm hyperactivation and capacitation induced by various biological fluids [103].
Besides the participation of ROS in physiological and defence processes, ROS are used as therapeutic agents too. The best example is probably photodynamic therapy (PDT). It requires a photosensitiser, visible light, and molecular oxygen to selectively kill cells. When localised in the target tissue, the photosensitiser is activated by light to produce oxygen intermediates (e.g., singlet oxygen) that destroy target tissue cells (see Section 3). The easy access of skin to visible light and molecular oxygen has led dermatologists to apply PDT to cutaneous disorders. In particular, PDT was shown to be successful in treating actinic keratoses (precancerous skin lesions), basal cell carcinoma, and Bowen's disease. The most popular photosensitiser is δ-aminolevulinic acid, the endogenous precursor of porphyrins, which does not make patients susceptible to phototoxicity for extended periods. Thus besides serious conditions such as nonmelanoma skin cancers and premalignant lesions, PDT is also used for the treatment of acne and as an adjuvant to photorejuvenation procedures [105,106].
7 Discussion
There are so many publications reporting the involvement of ROS and free radicals in biological processes that it is impossible to ignore them. A problem encountered with these molecules is the difficulty to detect them, due to their short half-life. Thus a complex physical instrumentation (and knowledge!) is required to analyse them directly, precluding their use in many laboratories equipped for standard biochemical research. For this reason, indirect analytical methods were developed, but the interpretation of the results, and their relationships to biological phenomena is often difficult. This explains in part the scepticism of many biologists and physicians towards this exciting subject.
In this review I discussed the ineluctable production of ROS and secondary oxidations in any aerobic organism. The nature of the ROS involved is well defined, and superoxide anion plays a pivotal role in the formation of other ROS, since it represents the required intermediate in any reduction of molecular oxygen. Another important point which one should bear in mind, is the biradical nature of molecular oxygen: this explains its moderate reactivity towards most (non-radical) biological molecules, but its high reactivity in the presence of free radicals. The detrimental role of ROS in frequent pathologies such as ischaemia and neurodegenerative disorders has been extensively studied, and the knowledge of the biochemical mechanisms involving ROS should allow developing new strategies to prevent and care these diseases [58]. Due to the unavoidable presence of ROS and free radicals in all aerobic organisms, the latter possess efficient antioxidant systems that prevent an excessive concentration of these molecules in biological tissues in normal conditions. However, various genetic or environmental conditions sometimes lead to an imbalance between the production and the decomposition of these reactive intermediates: this condition is called oxidative stress, and this balance disruption can have deleterious consequences. Conversely, an adequate level of certain ROS can have a physiological role, as for instance the catalysis of many biochemical reactions, the defence against invading pathogens or the capacitation of spermatozoa.
As a conclusion, the generation of ROS and free radicals is inevitable and useful in any aerobic organism, whereas oxidative stress is an undesirable condition that interferes with normal metabolism. A better understanding of this field, which is at the border of physics and life sciences, by all scientists involved in biomedical research, is desired and should improve our knowledge in biology and medicine.
Acknowledgements
I would like to thank Dr. Lars French for careful reading of the manuscript and his helpful comments.