1 Introduction
Since we all sleep, we all have a sense of what the process entails: recurrent periods of relative inactivity and unresponsiveness to sensory stimuli. But the physiologic and neural events that give rise to sleep require scientific scrutiny for their elucidation, and the selective forces that operate to maintain the ubiquity of sleep among animal species still remains a mystery [1]. This mystery is deepened by the selective costs of sleep, such as increased risk of predation and time lost that could be devoted to acquisition of food, shelter, and reproductive partners.
We wish here to consider the hypothesis that, in complex functioning nervous systems, sleep is obligated by constraints imposed by fundamental features of cell biology, and that these involve the need to coordinate intracellular and intercellular biochemical processes. For example, in order to function, neurons must extend neurites far from the cell nucleus while keeping in balance the metabolic turnover of all essential components via a cellular apparatus that has to respond dynamically to unanticipated environmental stimuli. Moreover, for the system to function, neurons with many different morphologies and neurotransmitter phenotypes must interact in a coordinated fashion over extended time periods. Sleep might provide the conditions necessary maintain or regain homeostasis by reapportionment of resources among such disparate intracellular and intercellular components.
Investigators who study sleep in vertebrates commonly classify sleep states on the basis of characteristic patterns of electrical activity within the neocortex. These EEG records can be partitioned into two distinct stages: Rapid Eye Movement (REM) sleep and Non-Rapid Eye Movement (NREM) sleep, which is also referred to as slow-wave sleep [2]. The neuronal processes that underlie these two sleep stages have been targets of intensive investigation, and it has been suggested that they arise as a natural consequence of features of the connectivity that characterizes the brains of all higher vertebrates. It has also been suggested that in these animals sleep contributes to higher-order cognitive functions, such as memory consolidation or reorganization, and some evidence in accord with this suggestion has been reported [3,4].
It has recently been reported, however, that fruit flies also experience recurrent periods of inactivity and unresponsiveness that are closely analogous to sleep in vertebrates [5]. As in vertebrates, these rest periods appears to play some homeostatic function, since animals that are actively deprived of the opportunity to sleep for a period of time tend to sleep longer when they subsequently have the chance to do so [6]. In fact, for both rats [7] and flies [8] sleep seems to play some vital function, in that a sufficiently long period of sleep deprivation is fatal. Since flies lack the cognitive abilities and cortical reentrant connectivity found in vertebrates, these observations suggest that sleep meets needs that must be met, not only at the system level, but also at cellular or sub-cellular levels. Some specific examples of cellular or sub-cellular processes that might require periodic periods of sleep for their long-maintenance are described below.
2 Sleep for glutamate redistribution
Most compounds that serve as neurotransmitters are synthesized specifically in neurons to serve that specific function. Glutamate is a notable exception to this rule. Although it is a necessary neurotransmitter at many important synapses in all animals investigated, it also serves many other metabolic roles within all living cells. It can function as an intercellular signaling molecule only because its concentration inside cells is maintained at far higher levels than those in the extracellular milieu. In the CNS of vertebrates this concentration gradient is maintained by glutamate pumps in the cell membranes of glial cells that recover glutamate that was released from neurons into the synaptic cleft. Once internalized in these glial cells, glutamate is aminated to form glutamine, which is then released to be actively taken up by neurons, within which it is hydrolyzed to reform glutamate that is reincorporated into synaptic vesicles [9]. It has recently been found that a similar system of glial cell uptake of released glutamate operates in insects as well [10].
The functioning of this glutamate-glutamine cycle, which is diagrammed in Fig. 1, has been well demonstrated experimentally [11]. It is particularly important in vertebrate brains where almost 80% of the neurons are glutamatergic. The cellular and subcellular location of each enzyme in the cycle illustrated has been well established and shown to be appropriate for its role in the process, with a single exception: the neuronal glutamine transporter. This carrier is commonly assumed to be located presynaptically within an axonal membrane, because this is where glutamate is found stored in synaptic vesicles, and a small fraction of one form of a glutamine transporter protein has been detected on axonal membranes [12]. This and other experimental evidence, however, localizes almost all of the glutamine transporter and uptake to somatodendritic membranes [12–14]. (The phosphate-dependent glutaminase that catalyzes the conversion of glutamine to glutamate is found within the mitochondrial matrix, and thus is present in axons, dendrites, and cell bodies [15].)

Proposed modification of glutamate–glutamine cycle characteristic of animal nervous systems. Glial glutamate transporters (1) take up synaptically released glutamate, which then reacts on glutamine synthetase (2) and the glutamine formed is released on a glial transporter (3). Neurons take up glutamine using the post-synaptically localized glutamine transporter (4). In neurons, glutamine is hydrolyzed by intramitochondrial glutaminase (5), and the glutamate formed is pumped into vesicles by the vesicular glutamate transporter (6). Operation of this cycle results in the net transfer of glutamate among neurons such that the vesicular glutamate concentration tends to decrease (pink color) in the more synaptically active neurons and increase (red color) in those that are less active.
If these empirical data are not misleading us, it appears that in order for glutamate to be replenished to physiologic levels in presynaptic boutons, the neurotransmitter must be transported all the way from the cell body. The glutamate vesicular transporter within the vesicular membrane maintains a high intravesicular neurotransmitter concentration by acting as a proton antiporter such that a significant fraction of glutamate in the axons is inside vesicles [16]. Even though there are usually several hundred vesicles in each bouton, if vesicles are released at only a moderate rate, say once per second, essentially all the glutamate molecules in each presynaptic site, (unless replenished), would be depleted within an hour.
It is plausible that during sleep those synapses that were most active during the previous wake period would become less so, and this period could serve as a time for vesicular replenishment. During the waking state one would expect that presynaptic glutamate would increase faster and to a higher degree in those cells that are least active, and diminish in those that are most active. This is because the site of neuronal glutamine uptake is so far from the site of glutamate release into the synaptic cleft; no known mechanism is in place to ensure that this neurotransmitter is taken up selectively by those cells that have become most depleted. Plausibly, the population of synaptic sites at which glutamate is released during slow wave sleep differs from the population of synapses active during the waking. If this is the case, then neural activity during sleep would tend to redistribute or reapportion glutamate from synaptic sites that had accumulated neurotransmitter (because of low release during waking) to those synapses that had become relatively depleted as a result of synaptic release during waking.
The need to reapportion glutamate among excitatory neurons in the CNS in the manner proposed here would also impact inhibitory interneurons. GABA, the major inhibitory neurotransmitter, is formed intraneuronally by the enzymatic decarboxylation of glutamate, which also enters the somatodendritic compartment of these cells in the form of glutamine. Thus, sustained activity of a subset of excitatory and inhibitory neurons within a neural network would tend to redistribute both glutamate and GABA to synaptic vesicles within the less active neurons, while depleting the remainder.
What would happen should an animal be deprived of sleep? According to this proposal, glutamate levels at the most active presynaptic sites would gradually decrease (Fig. 1), and there would be a consequent decrease in the efficacy of synaptic transmission. Since there are so many degenerate neural pathways in the brain [17], the effect of this synaptic fatigue might become evident only very gradually. One symptom might be a felt increase in the difficulty of concentrating or holding onto a thought, just as is commonly observed when one is sleepy.
Although this proposal for the function of sleep is relatively easy to state or understand, it is less easy to test experimentally. It predicts a decrease in the glutamate content at presynaptic sites of neurons that were active during the waking state, and that this decrease would be enhanced during sleep deprivation. It does not, however, necessarily predict an overall decrease in vesicular glutamate in the brain, since less active neurons would accumulate more during this process. At present no experimental method is available to quantitatively measure the glutamate concentration in individual vesicles. The demonstration of the presence of a presynaptic glutamine transporter [12] does not invalidate the proposal, since the difficulty of returning the released glutamate to just those cells and synapses where it was released remains formidable.
If there is a unidirectional flux of glutamate through axons from cell body to presynaptic sites of active neurons, might this not give rise to unequal distribution of glutamate throughout neural tissue? A parallel bundle of firing glutamatergic axons should generate a gradient of glutamate concentration with higher levels in volumes containing sites of vesicular release than in volumes containing the somatodendritic compartments where glutamate uptake takes place. This anatomical situation is not found in the vertebrate neocortex because the patterns of local axonal collaterals of excitatory neurons ensure that sites of glutamate release and uptake are not separated for significant distances.
In contrast, the relay neurons that comprise the bulk of thalamic nuclei do send signals in only one direction along the thalamocortical tract, and therefore mediate a net transfer of glutamate molecules from thalamus to cortex. Plausibly, a stable equilibrium is established by an equivalent flux of glutamate in the opposite direction via the corticothalamic fibers. Is it too far-fetched to suppose that the need to provide a mechanism for thalamic glutamate repletion was an important component of the selective pressure shaping the evolution and maintenance of the corticothalamic tract? Although this structure has obvious properties that could be utilized in coordinating and unifying neural activities in the brain through reentry, its complete function is somewhat mysterious, and one should not overlook a possible additional role in the necessary but mundane task of returning and thus reapportioning glutamate ions from cortex to thalamus.
3 Sleep for mitochondrial redistribution
Maintaining a population of neurons in a state capable of responding swiftly with appropriately timed and spatially distributed axon potentials and neurotransmitter release is a metabolically expensive process. A significant fraction of an animal's oxygen consumption takes place in the brain, for neurons rely chiefly on oxidative phosphorylation within mitochondria to generate most of the ATP utilized. These mitochondria are distributed throughout the cell; most synapses have one or more mitochondria located either pre- or post-synaptically, which implies that an average cortical pyramidal neuron contains at least 10 000 mitochondria at these locations far from the cell nucleus, and a Purkinje cell dendritic arbor can presumably contain ten times that number. Thus, neurons have to maintain a far larger population of mitochondria than do almost any other cells in the body. Since nuclear genes encode almost all polypeptide chains found within mitochondria, one might expect that most components of neuronal mitochondria are synthesized in the cell body close to the nuclear membrane, and experimental evidence suggests that this is the case [18]. It appears reasonable to assume that appropriate intraneuronal mechanisms have evolved to assure that mitochondria are transported throughout all cell processes in a manner sufficient to optimize ATP generation and meet metabolic needs.
Surprisingly little is known is regarding these processes. For example, we know little about what the half-life of brain mitochondria, how their intracellular distribution is regulated, or even how they are degraded. Since mitochondria tend to accumulate at sites of high levels of ATP utilization, it appears likely that an important intracellular signal sensed in this process either is, or correlates with, the local concentration of ADP, but this has not been experimentally verified.
Since most brain ATP consumption takes place in the neuropil, most newly formed mitochondria move, or are moved, up an ADP gradient into dendrites and axons and localize near synapses. Since energy consumption within the neuropil is higher in the waking state than during sleep [19], this might suggest that that the net flux of mitochondria away from the cell body is increased during the wake state.
If mitochondria leave the cell body, however, this would mean that fewer mitochondrial DNA molecules would remain in a cellular compartment where they are capable of replicating. Thus a dilemma has been created; mitochondria are required both far from the nucleus to supply local metabolic needs as well as close to the nucleus in order to maintain and increase the size of the intracellular mitochondrial population.
One way that natural selection might deal with this dilemma might be to lengthen the turnover time of mitochondrial components in neural tissue relative to that of other tissues of the body. Experimental results have shown this to be the case [20,21]. A second way to deal with this potential conflict would be to evolve animal drives that would insure that the CNS periodically enter a physiologic state characterized by lower synaptic activity and hence less energy consumption in neuronal processes, i.e. sleep. During this state the spatial ADP gradient in the cell would tend to reverse, and this would favor the movement of mitochondria back to the cell soma reapportioning them to a locale where they would be able to replicate, as schematically diagrammed in Fig. 2.
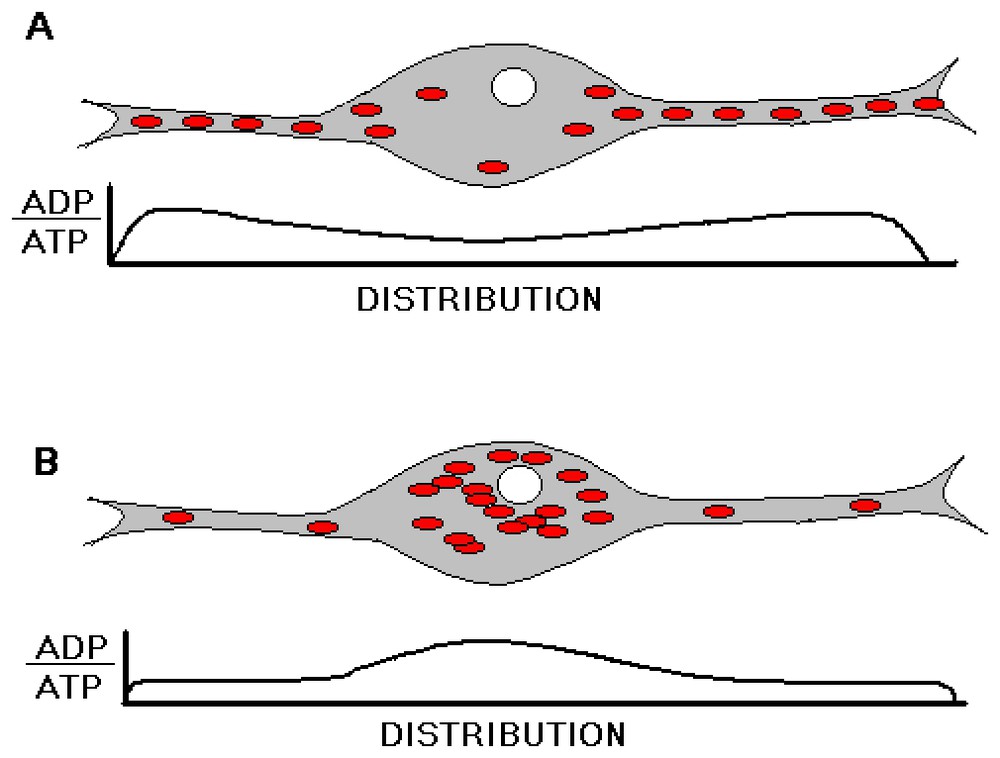
Schematic diagram of the proposed changes in the relative intraneuronal mitochondrial and energy level distributions during a sleep-wake cycle. In a state of sleep deprivation (A), synaptic activity in the neuropil is assumed to enhance ATP hydrolysis, and hence mitochondria accumulation, in the neuropil. Following sufficient sleep (B), neuropil becomes replete with ATP, and mitochondria accordingly move to the cell soma where they can replicate.
What would be the consequence of sleep deprivation, if we assume this mechanism contributes to the need for sleep? A decrease in the ability to regenerate new mitochondria to replace adequately those that, for one reason or another, become defective would tend to result in a deterioration of all energy-utilizing processes in the cell. Since most of the ATP consumed is coupled to ion pumps that maintain transmembrane potentials necessary for the transmission of action potentials and neurotransmitter turnover, very diffuse, generalized deficits in CNS function might appear. These deficits would tend to be self-aggravating, since efforts to compensate would tend also to utilize ATP, thus attracting more mitochondria into neuronal processes and depleting the store of mitochondria remaining in the cell body.
One clear-cut but experimentally untested prediction of this hypothetical function of sleep is that the ratio of mitochondrial DNA to nuclear DNA should decrease in the brains of animals that have experienced an extended period of sleep deprivation. In fact, it has been reported that transcripts of mitochondrial DNA are among the very few RNA species in the brain whose concentration varies in accord with the sleep-wake cycle [22].
4 Sleep to reapportion resources in disparate neural sub-systems
The two hypotheses outlined above illustrate the principle that large-scale properties of such basic organismal behavior, such as eating and sleeping, are shaped by constraints arising at the level of cells and molecules; we advance them here to point out surprising lacunae in our knowledge of these constraints. Some of the difficulties dealt with by natural selection during the evolution of neurons arose from the need to adapt complex intracellular structural and metabolic networks to function effectively over extended spatial and temporal scales. But to contribute to animal survival, neurons must organize themselves into integrated but multicellular subsystems comprised of heterogeneous cell types. At this level of organization inhomogeneities also arise that must be dealt with. For example, the time required to deplete or resupply presynaptic vesicles to boutons of catecholinergic axons almost certainly differs from that needed for glutamatergic, GABAergic, or cholinergic axons. It may be that stages of sleep provide a period of time during which such diverse populations of neurons can adjust their internal constituents to allow optimal coordination among their mutual interactions required for useful waking behavior.
Jouvet has demonstrated that maintenance of the waking state requires the functioning of the diffuse projection systems arising in the brainstem, most significantly noradrenergic neurons in the locus coeruleus, serotonergic neurons in the dorsal raphé nucleus, and cholinergic neurons in tegmental nuclei [23,24]. The neurons in these nuclei are more active when an animal is awake than when in slow wave sleep, and the extracellular concentrations of these neurotransmitters vary in phase with the sleep-wake cycle. Evidence suggests that signals mediated by these compounds are required to tune the properties of cells in the corticothalamic system in a manner that permits mental functioning. These systems acting together are thus considered to serve as major controllers of sleep and wakefulness [25].
We wish here to put forward the complementary notion that the coordination of the activity of such biochemically diverse neural populations cannot be sustained for an indefinite time period. As time passes, precursors are depleted, products and side-products accumulate and become maldistributed, synthetic and catabolic processes become ‘out-of-sync’, and the need to reapportion neural resources grows. The lowered activity of cells in these systems during sleep might be required to allow this reapportionment to take place. From this perspective, these brainstem systems have not just evolved to control sleep; rather sleep has evolved to permit these systems to function effectively.
None of the three reapportionment functions proposed here for sleep excludes the other two, nor do they exclude the possibility that sleep serves to mediate some other necessary physiologic functions. For example, it has been proposed that sleep provides an opportunity to regenerate glycogen stores depleted by waking activity [26], and evidence consistent with that hypothesis has been reported [27]. Moreover, the mechanisms proposed for the redistribution of glutamate and mitochondria would very likely function during slow-wave sleep. The third proposal, which involves the realignment of neural subsystems employing disparate neurotransmitters, may require that most cells of the diffuse ascending system cease firing, a characteristic feature of rapid-eye-movement (REM) sleep. The fact that the length of REM sleep periods are homeostatically regulated suggests that this sleep state provides the physiologic conditions for some specific reapportionment process. Evidence has been presented suggesting that REM sleep occurs in order to compensate for some unknown process that takes place during only during non-REM sleep periods [26]. Other evidence, however, argues that the build up of REM pressure and its subsequent rebound is independent of time spent in slow-wave sleep [28]. Since REM sleep has so far not been described in invertebrates, its function may also relate to other constraints inherent to neuroanatomical features that are found only in vertebrates, e.g., reentrantly interconnected cortices [29].
If sleep has evolved to perform basic cellular functions such as those proposed here, this would entail the simultaneous evolution of the CNS and other physiologic systems in a way that would create the appropriate neural structures and drives to generate and maintain relatively safe sleep. It is important, however, to distinguish between the functions of sleep, per se, and the function of systems that operate to insure that sleep does, in fact, occur.
Even if it is found that animals need to sleep in order to maintain states of cellular homeostasis by one of the mechanisms proposed here, it is probable that the sleep state would take on additional functions in more complex organisms. It is plausible, for example, that sleep does provide useful, perhaps essential, physiologic conditions needed for memory storage, consolidation, or reconfiguration, and perhaps even more importantly, time to ‘knit up the raveled sleeve of care’.
Acknowledgment
This work was supported by the Neurosciences Research Foundation.