Version française abrégée
La thermogenèse est une conséquence obligatoire du métabolisme cellulaire. Elle est liée à l'utilisation de l'oxygène et la mesure de la consommation d'oxygène par des cellules ou des organismes est d'ailleurs utilisée pour la quantifier. Lorsque des voies cataboliques fonctionnent, l'énergie libre disponible est accrue. En général, une grande partie de cet accroissement d'énergie est utilisée par des réactions endergoniques, bénéficiant alors d'un apport d'énergie. Toutefois, le couplage des réactions libérant de l'énergie et des réactions la consommant étant inférieur à 100%, une fraction de l'énergie est obligatoirement dissipée sous forme de chaleur. Les mammifères homéothermes doivent produire de la chaleur s'ils sont exposés à une température externe froide. Ils le font en augmentant l'oxydation de molécules carbonées, celle des acides gras en particulier. Ceci établit un lien avec les éventuels problèmes métaboliques responsables de l'obésité ou avec des stratégies favorisant la lutte contre l'excès de graisse.
La thermogenèse comprend plusieurs composants : la thermogenèse obligatoire liée au métabolisme de base, la thermogenèse post-prandiale, la thermogenèse induite par l'activité physique, la thermogenèse adaptative correspondant essentiellement à la dépense énergétique induite par une température basse de l'environnement. Récemment, des auteurs ont proposé de prendre en considération la dépense énergétique physique non liée à l'activité physique importante. Cette thermogenèse provient des mouvements effectués quotidiennement dans le cadre de la vie courante : par exemple se lever fréquemment ou non.
Les mécanismes moléculaires thermogéniques sont contrôlés par le niveau de couplage entre réactions chimiques exergoniques et endergoniques, ainsi que par les activités des ATP-ases capables d'hydrolyser l'ATP (adénosine triphosphate), qui représente la forme de stockage et d'utilisation de l'énergie chimique. L'obésité résultant d'un déséquilibre entre apport d'énergie et dépense d'énergie, un déficit de thermogenèse pourrait contribuer au stockage de triglycérides. Cette hypothèse est corroborée par le fait que des déficits d'oxydation du glucose ou des acides gras ont été décrits chez certains patients obèses.
Outre les mécanismes liés à la restriction alimentaire et/ou à l'augmentation d'activité physique, le paramètre biochimique qui pourrait influer le plus sur le stock de graisses corporelles est certainement la valeur de la thermogenèse métabolique résultant du niveau de couplage entre réactions cataboliques d'oxydation et réactions anaboliques de synthèse. En d'autres termes, toute réduction de l'oxydation des substrats (glucose, acides gras) sans réduction de la synthèse de l'ATP favorise l'obésité. A contrario, toute augmentation de l'oxydation des molécules carbonées non associée à un accroissement de la synthèse de l'ATP favorise la perte de poids. Cette hypothèse a été, malheureusement, validée il y a plusieurs dizaines d'années chez des patients traités avec le 2,4-dinitrophénol, une substance découplant la respiration de la synthèse de l'ATP.
Les mitochondries sont le lieu de la respiration et de l'oxydation cellulaire. Lors de la respiration cellulaire, une grande quantité d'énergie libre est libérée, mais une part importante de cette énergie est transformée en ATP par l'ATP-synthase mitochondriale. Ceci correspond au couplage de la respiration à la phosphorylation de l'ADP. Ce processus est souvent désigné par les termes : oxydation phoshorylante ou phosphorylation oxydative. Un excès du couplage respiratoire augmente le rendement en ATP et favorise le stockage de l'énergie (et éventuellement le gain de poids) ; un déficit du couplage respiratoire réduit la production d'ATP et défavorise le stockage. Il y a donc ici un mécanisme pouvant contrôler la teneur en graisses et capable d'expliquer certaines obésités et/ou d'être pris en compte pour développer des molécules favorisant l'oxydation des acides gras au détriment de la synthèse d'ATP. Toutefois, l'exemple du 2,4-dinitrophénol cité plus haut indique combien l'utilisation de découplants chimiques peut être dangereuse.
En fait, les travaux de physiologistes de la thermogenèse ont permis de relancer cette voie de recherche. Il s'agit des travaux effectués depuis 1960 sur le tissu adipeux brun des mammifères. En bref, les mammifères adultes de petite taille, les mammifères nouveau-nés et les animaux hibernants possèdent du tissu adipeux brun, dont la fonction est de produire de la chaleur en réponse au froid, à la naissance, ou lors du réveil chez l'hibernant. La chaleur produite est importante et est évacuée par des vaisseaux sanguins vers les régions cérébrale, cardiaque et rénale. Les adipocytes bruns qui composent ces dépôts adipeux particuliers sont caractérisées par un très grand nombre de mitochondries, elles-mêmes très riches en membrane interne, lieu de la respiration cellulaire. Le substrat utilisé correspond aux acides gras libérés par lipolyse à partir des gouttelettes de triglycérides. Le mécanisme de la thermogenèse dans ces cellules a été élucidé. Cette thermogenèse intense ne résulte pas seulement d'un forte oxydation des acides gras, mais est expliquée par un découplage naturel de la respiration. Ainsi, dans les adipocytes bruns oxydatifs et thermogéniques, la respiration est découplée de la phosphorylation de l'ADP. La vitesse de respiration des adipocytes bruns n'étant pas limitée par leur capacité de phosphoryler l'ADP, la respiration est intense et très thermogénique, puisque l'énergie libérée n'est pas consommée par la synthèse d'ATP. La protéine responsable de découplage respiratoire a été identifiée et nommée UCP1 (uncoupling protein). Cette protéine est un cible potentielle de molécules favorisant l'oxydation des graisses, la thermogenèse et la dépense énergétique. Très récemment des protéines homologues de cette UCP1 ont été identifiées, mais leur véritable activité découplante n'est pas prouvée.
1 Introduction
All uni- and multicellular systems are thermogenic. Thermogenesis is linked to oxygen consumption and is an inevitable result of metabolic, and in particular catabolic, activity, since not all the energy released by oxidation can be recovered in chemical form (generally ATP). While hibernating animals must produce a great deal of heat when they awake, non-hibernating mammals, like man, maintain their body temperature at 37 °C by permanent metabolic thermogenesis. At the same time, thermolysis systems prevent a temperature rise. Thermogenesis is largely associated with the oxidation of carbon substrates that accumulate in obese subjects, and so is an area of research that aims both to understand and treat obesity.
2 Physiological components and molecular mechanisms of thermogenesis
Energy expenditure comprises combustion of substrates by oxygen and the body's work (synthesis, maintaining ion gradients, muscle activity, …). This expenditure is conventionally divided into four types: (1) obligatory thermogenesis related to cellular metabolism, functioning of organs, maintenance of the body's vital functions; (2) postprandial thermogenesis, or the thermal effect of food; (3) thermogenesis resulting from physical activity; and (4) adaptive thermogenesis. The most variable part of energy expenditure is that corresponding to physical activity (physical exercise and non-exercise activity thermogenesis, see below). Adaptive thermogenesis corresponds to changes in energy expenditure as a function of ambient temperature and diet.
At the molecular level, thermogenesis arises from reactions that are imperfectly coupled thermodynamically, for example, ion transport and ATP utilization, re-oxidation of coenzymes and ADP phosphorylation. Apart from the particular situation of intense physical exercise, a large proportion of the cellular heat of resting energy expenditure is produced by cellular respiration, which is the re-oxidation of the reduced coenzymes NADH and FADH in the respiratory chain. These oxidations are exergonic and greatly increase the free energy available. In the absence of the coupling of respiration to ATP synthesis, an endergonic process, cellular respiration seems to generate just heat. The coupling of re-oxidation of reduced coenzymes to ADP phosphorylation consumes a large part of the energy released by the oxidations and greatly reduces the proportion of energy released as heat. The molecular mechanism of the coupling of mitochondrial re-oxidation of the reduced coenzymes (via the complexes that form the respiratory chain) to ADP phosphorylation (by ATP synthase) will not be detailed here. Briefly, on oxidation of the coenzymes, three respiratory chain complexes work as proton pumps and expel protons into the mitochondrial intermembrane space, generating there a proton gradient. The energy of this gradient is then utilized by mitochondrial ATP synthase to phosphorylate ADP (Fig. 1).
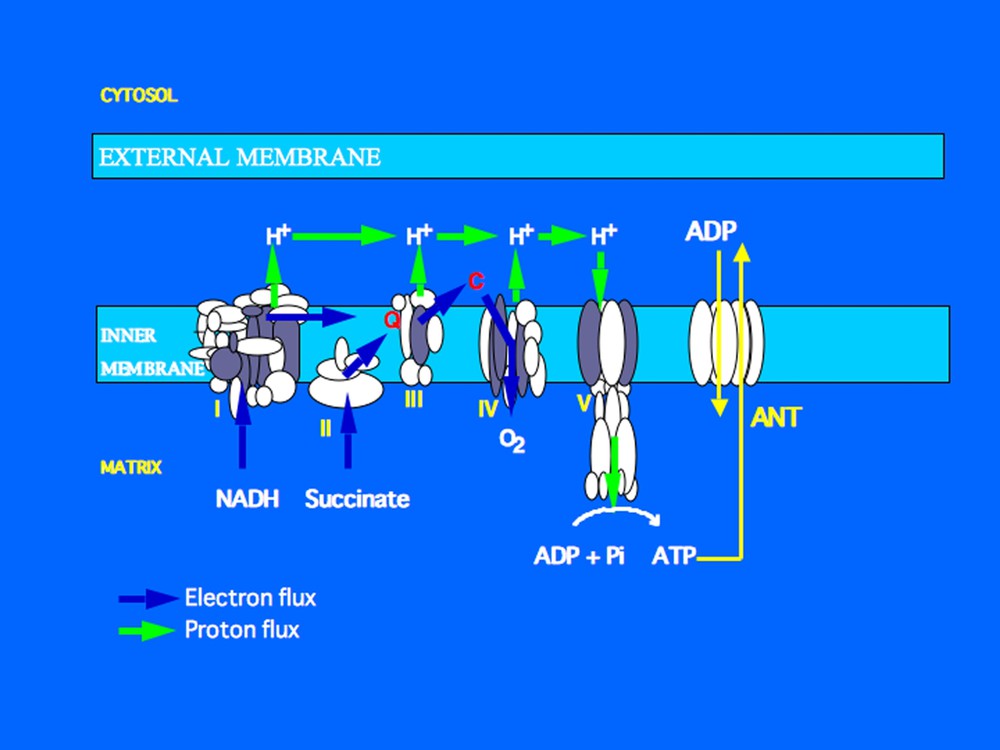
The mitochondrial respiratory chain is located in the inner mitochondrial membrane. It is composed of four complexes (roman numerals). These complexes re-oxidise reduced coenzymes (NADH produced by the Krebs cycle, FADH produced during oxidation of succinate). The complexes and coenzyme Q (Q) work as electron and/or proton transporters. The final acceptor of electrons is molecular oxygen which interacts with electrons and protons to generate water. Complexes I, III and IV work as proton pumps and generate a proton electrochemical gradient which is used by the ATP-synthase (also referred to as complex V) to phosphorylate ADP into ATP. ADP enters the matrix through the adenine nucleotide translocator (ANT) which exchanges ADP against ATP.
In standard conditions, the mitochondrial respiration of all cells is coupled to ATP production and constitutes the main source of ATP. However, as the coupling of respiration to ADP phosphorylation is less than 100% efficient energetically, respiration also releases heat. This mechanism is considered to explain in large part the heat produced by resting thermogenesis (Fig. 1). We will discuss below the question of the contribution of the partial coupling (or uncoupling) of respiration/ATP synthesis to adaptive thermogenesis or to the energy imbalance involved in obesity.
The other thermogenic biochemical reactions principally involve futile cycles and ATPases, which include the energy-dissipating systems ATPase-Na+/K+ of the plasma membrane and Ca++-ATPase of the sarcoplasmic reticulum. The Na+/K+-ATPase pump maintains the plasma membrane potential and ATP hydrolysis and contributes to energy expenditure. Intracellular calcium homeostasis and calcium recycling lead to a high consumption of ATP by the Ca++-ATPase of the sarcoplasmic reticulum.
3 Thermogenesis and obesity
Obesity can be said to result from a difference between energy input and energy output. Excessive food intake is a frequent cause of obesity, but, in theory, a deficit in energy expenditure can also contribute to the storage of triglycerides and to obesity. This is why resting energy expenditure must be measured in obese patients [1,2]. Many studies have been based on measurements of the oxidation of glucose or fatty acids. Deficient fat oxidation may lead to the accumulation of triglycerides in obese subjects. Although it can be very slight and difficult to detect, this effect can result in overweight in the long term. Deficits in Ca++-ATPase of the sarcoplasmic reticulum or in the Na+/K+-ATPase pump of the plasma membrane have not been implicated in human obesity. Gene polymorphism studies have shown that the protein UCP1, which is responsible for respiratory uncoupling of brown adipocytes and for the dissipation of energy from fatty acid oxidation, contributes over time to control of gain in body fat [3]. The notion of non-exercise activity thermogenesis (NEAT) has recently been put forward in terms of the control of body weight and fat mass [4]. This is the physical activity associated with the usual, everyday movements.
4 Brown adipose tissue
Deposits of brown fat, as distinct from white or yellow fat, in many mammals have long been known to biologists and histopathologists, and correspond to brown adipose tissue (BAT) and white adipose tissue (WAT), respectively. Extensive work over the last 30 years, principally on rodents, has demonstrated the thermogenic function of BAT. Activated BAT rapidly oxidizes fatty acids and produces heat. This is achieved by the numerous mitochondria in brown adipocytes and a specific mitochondrial membrane protein called UCP (uncoupling protein), which activates respiration and diverts the free energy of oxidation to thermogenesis. BAT therefore contrasts with WAT, which stores energy. BAT is generally undeveloped or inactive in animal models of obesity. This inactivity may reduce fat oxidation and so promote increased adiposity.
4.1 Morphology, development and presence of brown adipose tissue
The most abundant cells in BAT are brown adipocytes and endothelial cells associated with precursor cells that can proliferate and differentiate into brown adipocytes. A brown adipocyte contains numerous fat droplets, innumerable mitochondria and a central nucleus (Fig. 2). The mitochondria are striated and contain numerous crests formed by the inner membrane containing the uncoupling protein UCP. Brown adipocytes can also be seen among white adipocytes in WAT, particularly in animals chronically treated with β-adrenergic agonists. These observations illustrate the orthosympathetic and noradrenergic activation of the development of brown adipocytes [5–8].
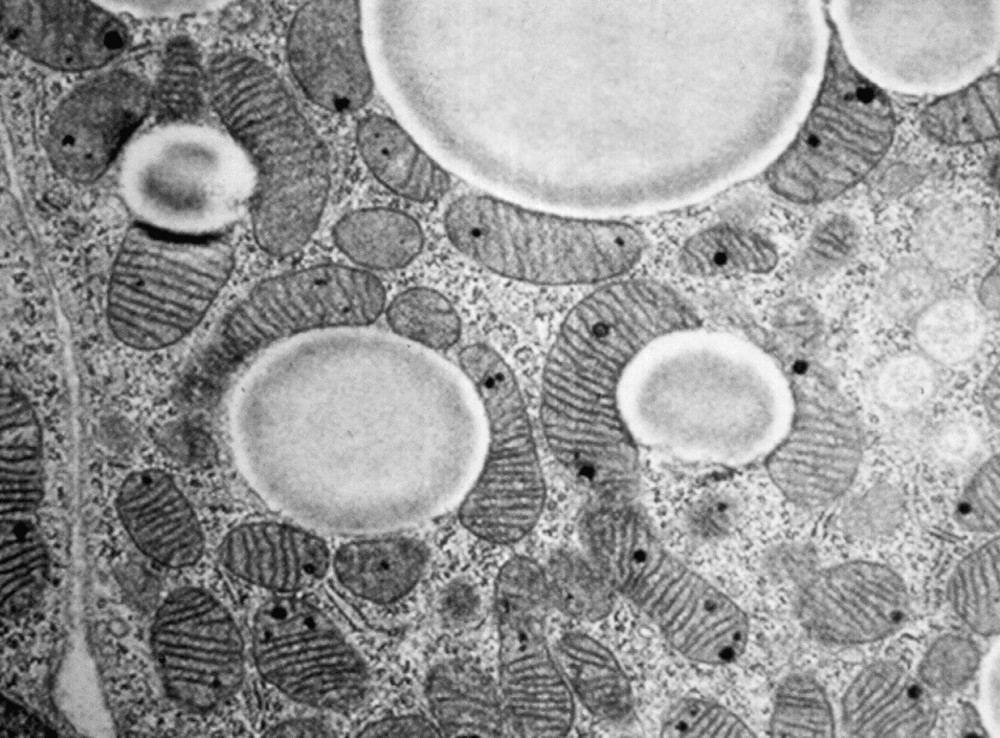
Morphology of brown adipose tissue (documents kindly provided by Professor S. Cinti, University of Ancona, Italy). Brown adipocytes contain fat droplets and numerous mitochondria.
BAT is located bilaterally principally in the cervical, periaortic, pericardiac, perirenal and interscapular (in rodents) areas. Arteries supply blood to BAT and veins drain it. Numerous anastomoses control the arrival of blood in the capillary beds. On activation of BAT, the shunts open and blood flow through the tissue increases very rapidly, 20- to 200-fold, for example, in resting BAT. The blood leaving BAT is distinctly warmer than the incoming arterial blood. Brown adipocytes and thermogenesis are activated by orthosympathetic noradrenergic fibers innervating each cell. This orthosympathetic innervation is itself controlled by the hypothalamic centers.
BAT is present in the newborn and young of most animal species that require non-shivering thermogenesis to compensate for heat loss when they leave the nest. In most species BAT appears during fetal life. It is fully developed in the newborn guinea pig, but several days are needed in the young rat and several weeks in the golden hamster for a developed and active BAT. When BAT develops, adipocyte differentiation is accompanied by morphological changes including an increase in the number of mitochondria and in the mitochondrial crests. UCP appears two to three days before birth in non-hibernating rodents and carnivores. Very old work describes BAT in newborn rabbits and guinea pigs, in animals emerging from hibernation, and in cold-acclimated rats. Birth, the end of hibernation, and exposure to cold all require heat production and illustrate the thermogenic activity of BAT. BAT accounts for 1% of body weight in adult rats and 5% in newborn rabbits. BAT is present in babies, very young lambs, goats, calves, deer and monkeys, but absent in monotremes, marsupials and pigs. UCP has been found in brown adipocytes in newborn lambs and calves, as well as in the babies at birth [5–8].
Brown adipocytes are observed at the 20th week of gestation in the human fetus, and UCP is detectable at the 25th week. Six main deposits account for 90% of total BAT in babies. These deposits are located in the cervical, axillary, perirenal, periadrenal, and pericardiac areas, and also in the posterior part of the abdominal cavity. The remaining 10% of the BAT is located along the thoracic aorta, intercostal arteries, abdominal aorta, and saphenous veins, and in the omentum. In the months and years after birth, BAT accumulates lipids and the morphology of most brown adipocytes is that of white adipocytes. So, human BAT is soon replaced by WAT from which the brown adipocytes have disappeared or have become thermogenically inactive. Characteristic islands of brown adipocytes can, however, be observed in the human adult in body areas containing BAT in babies. BAT can also be present in adult humans in the form of a tumor called a hibernoma. It is also easily detected in patients with pheochromocytomas, thus confirming the adrenergic control of its development [5].
4.2 Physiological functions of brown adipose tissue and their control
It has been known since the 1960s that BAT contributes to the homeothermy of mammals. In mammals, the metabolic activities of all cells generate heat, which contributes to basal metabolism. Heat produced by BAT corresponds to so-called facultative or regulatory thermogenesis, and is ‘extra heat’ rapidly generated by brown adipocytes to meet immediate heat requirements. The other mechanism that generates regulatory heat is muscle shivering. Compared with large mammals and adult mammals, small mammals and newborn large mammals have a high body surface/weight ratio, which means that heat loss is greater and the need for thermogenesis more marked. BAT meets these requirements and is thermogenically active in newborn mammals and small rodents (especially if exposed to cold). For example, in the adult rat exposed to a temperature of 4 °C, BAT supplies most of the heat, which is about four times the amount required for basal metabolism.
Newborn humans exposed to cold are incapable of shivering, and the thermogenic activity of BAT maintains the body temperature [5–8]. Activation of BAT can double the body's oxygen consumption in babies exposed to a moderately low temperature. Certain anesthetics inhibit the thermogenic response of isolated brown adipocytes, suggesting that inhibition of BAT activity contributes to hypothermia in babies undergoing surgery. Some authors have proposed that BAT participates in the excess thermogenesis sometimes described in sudden infant death syndrome, although there is as yet no proof of this. The thermogenic activity of BAT in adults is probably very low, but is perhaps not negligible since BAT is easily detected in people who are often exposed to cold. The small quantity of BAT found in human adults cannot, however, account for more than 1 to 2% of fatty acid oxidation and total energy expenditure.
The thermogenic function of BAT was initially observed in hibernating animals. Before entering hibernation, these animals exhibit hyperphagia and develop seasonal obesity, and their BAT increases in abundance. At the end of hibernation, BAT plays an essential part in rapid body warming, to which muscle shivering also contributes.
The low thermogenic activity of BAT in genetically obese ob/ob mice could predispose to obesity. In rats, the ‘cafeteria diet’ stimulates the growth and thermogenesis of BAT. These observations suggest that BAT thermogenesis participates in diet-induced thermogenesis and in the energy balance [9]. This hypothesis is confirmed by the fact that mice whose BAT has been destroyed by transgenesis become obese, although these observations are questioned because the same mice rapidly develop hyperphagia.
The thermostatic theory of food intake control has been neither refuted nor confirmed. Given that food intake activates the BAT of rodents, it has been suggested that BAT thermogenesis could control both food intake and satiety. Thus, BAT would be activated following a meal and would raise the central and brain temperature, which in turn would inhibit food intake and return BAT thermogenesis to resting levels. The low BAT thermogenesis of obese animals could therefore explain why these animals do not stop eating. Himms-Hagen has suggested that this model may be applicable to human newborns during the first weeks of life since they have abundant BAT [5].
4.3 Molecular mechanisms
Morphological examination indicates that brown adipocytes and white adipocytes differ and constitute two distinct types of adipose tissue: BAT is not a fetal or immature form of WAT. In fact, the precursors of white and brown adipocytes differ, even though their morphology is similar. Precursors isolated from WAT differentiate into white adipocytes in vitro and do not express the uncoupling protein UCP. Precursors isolated from brown adipose tissue, on the other hand, differentiate in vitro into brown adipocytes containing UCP.
While some adipose deposits are exclusively white, and others brown, many contain a few adipocytes of the other type. For example, characteristic brown adipocytes are seen in the parametrial white fat of the mouse or the periovarian fat of the rat. The same is true of the WAT of baboons and adult humans. These observations raise questions regarding the possible plasticity of WAT and BAT and the existence of adipocytes that can switch from the white to brown phenotype, and vice versa.
The spectacular induction of true brown adipocytes in patients with pheochromocytoma, and in adult dogs or in rats treated with β3-adrenergic agonists may be explained both by the recruitment of new brown adipocytes and the activation of ‘dormant’ brown adipocytes, which must receive the appropriate stimulus to express UCP and all the morphological and functional features of brown adipocytes [5]. In fact, it is unclear what mechanisms underpin the differentiation of adipoblasts into white or brown adipocytes. It seems certain that there are adipocytes that will never be able to express UCP or to possess numerous thermogenic mitochondria. Moreover, depending on the animal species, their anatomical location, and the strength of the adrenergic stimulation, brown adipocytes may express their characteristics weakly (dormant brown adipocytes) or strongly (mature brown adipocytes).
White and brown preadipocytes contain many mitochondria. However, white adipocytes lose a large proportion of their mitochondria on differentiation, whereas brown adipocytes keep many mitochondria characterized by numerous folds of the inner membrane (Fig. 2). The mechanisms controlling the marked development of the chondrioma in brown adipocytes have not been identified, although it is known that the transcriptional co-activator PGC-1 plays a vital role in this control [10]. UCP1 plays a key part in BAT physiology as it is a characteristic marker of brown adipocytes and confers on mitochondria and on brown adipocytes their specific physiological capacity to dissipate oxidation energy as heat [7,8,11].
In all cells, the coupling of respiration to ATP synthesis means that the respiration rate is limited by the ADP available and by the rate of ADP phosphorylation. Surprisingly, the first work on the mitochondrial bioenergetics of brown adipocytes showed that their respiration is not coupled to mitochondrial ADP phosphorylation. In other words, the mitochondrial respiration of brown adipocytes is not limited by the ADP available or ATP synthesis, and is therefore intense and generates heat. The mechanisms involved are explained by Peter Mitchell's chemiosmotic theory which holds that the proton conductance of the inner mitochondrial membrane controls the rate of respiration and the coupling of this respiration to ATP synthesis (see Fig. 1). In the mitochondria of thermogenic brown adipocytes, the return of protons to the matrix is not mediated by ATP synthase. These mitochondria have a specific proton return that short-circuits ATP synthase and inhibits ATP synthesis while activating respiration. The mitochondria of brown adipocytes therefore exhibit rapid respiration and produce heat since the energy of oxidation is not coupled to ADP phosphorylation, which is energy-consuming. The protein that carries protons through the inner membrane of the mitochondria of brown adipocytes and uncouples respiration was identified in 1976–1978 and dubbed UCP, or the uncoupling protein (Fig. 3). The name UCP1 has been used since 1997, when the protein homologs UCP2 and UCP3 were identified. UCP1, whose apparent molecular weight is 33 000, is present only in the mitochondria of brown adipocytes. It is induced in the BAT of rats exposed to cold and its respiration uncoupling activity is inhibited by certain nucleotides and activated by free fatty acids. UCP1 contains 300 amino acids and has a trimeric structure similar to that of the mitochondrial ADP/ATP exchanger and of the mitochondrial phosphate transporter. UCP1 belongs to the large family of mitochondrial anion transporters, all of which derive from the triplication of an ancestral gene [11].
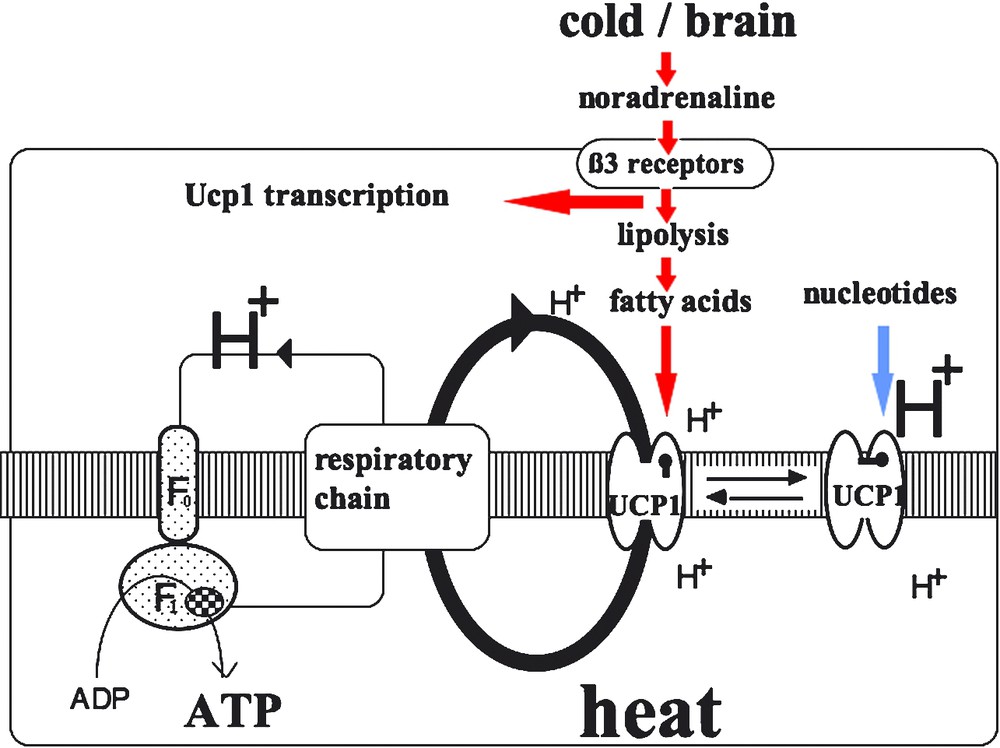
Activation of respiratory uncoupling and heat production in brown adipocytes. On the left is illustrated the formation of a proton gradient by the respiratory chain complexes and the proton circuit though the inner mitochondrial membrane coupling respiration to ATP synthesis in the mitochondrial matrix. If active UCP is present and becomes activated, the proton gradient is dissipated by short-circuiting ATP synthase (F0F1 complex). In such a situation, energy gradient is not used for ADP phosphorylation and is dissipated as heat. In parallel, the respiration rate is greatly increased since dissipation of the proton gradient by UCP results in a drop in membrane potential which in turn stimulates the proton pumps of the respiratory chains. When no extra-heat production is required, the brown adipocyte is not stimulated by noradrenaline and UCP1 is inhibited by nucleotides such as ATP, GDP, GTP. When thermogenesis is required, e.g., during exposure to the cold, the brain is informed by thermo-receptors and triggers the sympathetic nervous fibers innervating brown adipocytes. Noradrenaline binds adrenoceptors (in particular β3-adrenoceptors) which activate adenylate cyclase, cAMP synthesis and lipolysis and produces free fatty acids. These fatty acids overcome the inhibition of UCP1 by nucleotides and make the protein able to derive protons from ATP-synthase. In addition, cAMP increase induced by the noradrenaline activation of cells stimulates the transcription of the UCP1 gene.
The mechanism of the action of UCP1 has not yet been fully elucidated. When incorporated in liposomes, UCP1 transports protons, but is also able to transport other ions, notably anions. The uncoupling activity of UCP1 is strongly controlled by inhibiting and activating ligands. When there is no need for extra heat, brown adipocytes are not thermogenic, since they are not stimulated by catecholamines and also because ATP and GDP bind to and inactivate UCP1. In a situation involving thermogenesis, brown adipocytes are activated by the noradrenaline of orthosympathetic fibers. Lipolysis is then activated in brown adipocytes and the resulting fatty acids directly activate UCP1, which uncouples and activates respiration (Fig. 3). Various studies indicate that UCP1 transports not protons but rather fatty acids: protonated fatty acids passively enter the matrix where they release a proton, giving rise to anionic fatty acids, which UCP1 transports to the intermembrane space [12–14].
In vivo and in vitro work has shown that the synthesis of UCP1 is essentially controlled transcriptionally and is strongly activated by catecholamines and cyclic AMP. Thyroid hormones and retinoic acid also activate gene transcription. Several molecules controlling UCP1 gene transcription have been identified, and also enable this transcription to occur specifically in brown adipocytes. Human UCP1 gene transcription is also controlled by molecules similar to those identified in the rat and mouse. Transcription factor PPAR-γ controls UCP1 gene transcription, and PGC-1 coactivator seems to play a key role in UCP1 gene transcription in mice and humans, by co-activating PPAR-γ, the RXRα receptor, thyroid hormone receptors and retinoid receptors [10]. UCP1 gene knockout by homologous recombination prevents the mutant mice from maintaining their body temperature on exposure to cold [15]. This shows that UCP1 and its respiration uncoupling activity are responsible for cold-induced thermogenesis in the mouse.
The diverse effects of catecholamines on BAT (development, activation) are mediated by several types of adrenergic receptors. Pharmacological work shows that the principal β-adrenergic receptor is neither type β1 (as in the myocardium) nor type β2 (as in the airways), but rather a β3-adrenergic receptor whose cDNA was cloned a few years ago. The β3 receptor is present in white and brown adipocytes of rodents. Treatment of rats with a β3-adrenergic agonist strongly induces UCP1, as well as brown adipocytes in various adipose deposits. This is also observed in the dog and macaque [5].
5 Thermogenesis and the pharmacotherapy of obesity
Hormonal effectors that activate thermogenesis are mainly those of the thyroid and adrenergic systems. However, thyroid hormones or catecholamines cannot be utilized to activate thermogenesis in obese patients. Malignant hyperthermia is due to perturbation of ryanodine receptors controlling calcium transport in the endoplasmic reticulum. These observations provide no clues to the pharmacotherapy of obesity. Knowledge of thermogenic mechanisms and of the thermogenic functioning of brown adipocytes provides some pointers for pharmacological research. β-adrenergic receptors, the transcriptional coactivator PGC-1, the transcription factor PPAR-γ, and UCP1 are potential targets for drugs that promote BAT development, fatty acid oxidation, and energy dissipation as heat. Noradrenaline's activation of brown adipocytes is mediated by β3-adrenergic receptors, which stimulate cAMP production and lipolysis, resulting in free fatty acids which activate UCP1 and respiratory uncoupling. Catecholamines acting on β3 receptors also have a trophic effect on BAT. PGC-1 and PPAR-γ stimulate UCP1 synthesis, and adrenergic receptors are rare in adult humans. It seems that brown adipocytes are the only human cells that have such receptors, and whereas babies have brown adipose tissue, brown adipocytes are rare in adults. These findings suggest another approach, which is to stimulate BAT. Encouraging results have been recorded by D. Langin's team, which has succeeded in inducing UCP1 and activating palmitate oxidation in cultured human adipocytes that overexpress PGC-1 [16]. Certain PPAR-γ ligands activate UCP1 gene transcription.
The UCP1 homologs UCP2 and UCP3 identified a few years ago [17–21] are probably not involved in thermogenesis and their exact biological roles remain to be clarified. However, the development of drugs to activate or induce UCP1, or other UCPs, or even other mitochondrial transporters that slightly uncouple respiration from mitochondrial ATP synthesis, could help to characterize novel anti-obesity drugs. It should be remembered that Luft's disease (hypermetabolism) involves hypercatabolism and weight loss due to marked respiratory uncoupling in muscle mitochondria [22]. The involvement of UCP in Luft's disease has not yet been studied. This very rare disease demonstrates the metabolic importance of the degree of coupling of mitochondrial respiration to ATP synthesis, as also illustrated by transgenic mouse models that overexpress a UCP [23,24].
Acknowledgements
This work was funded by the ‘Centre national de la recherche scientifique’ (CNRS), the ‘Institut national de la santé et de la recherche médicale’, the ‘Association de recherches sur le cancer’, the ‘Institut de recherches Servier’, the European Union (contract Diabesity No. LSHM-CT-2003-503041), the ‘Actions concertées incitatives du ministère de la Recherche’ (France).