1 Introduction
There exist two broad categories of seeds. The one is the so-called orthodox seeds that sustain a strong desiccation by the end of their maturation on the mother plant and retain their germination potential over long periods of storage in the dry state. The other is the so-called recalcitrant seeds, which have high water content at maturity and are intolerant to desiccation. This last category of seeds in fact starts their germination program immediately after the maturation phase, without going through the stage of drying and metabolic quiescence. Because of their storage ability in the dry state, orthodox seeds are the most commonly used in agriculture and the seed industry. However, some economically important species such as coffee (Coffea spp.) or cocoa (Cocao spp.) produce recalcitrant seeds.
The desiccation tolerance of orthodox seeds is under the dependence of many genes [1], whose expression is usually controlled by the phytohormone abscisic acid (ABA) [2], a sesquiterpene compound resulting from the cleavage of carotenoids, and for which virtually the entire biosynthesis pathway has been identified through the characterization of auxotrophic mutants [3]. This molecule exerts a stimulatory role in the synthesis of seed storage reserves. ABA also controls the expression of LEA (Late Embryogenesis Abundant) proteins, which become abundant during late maturation, when desiccation starts and seeds enter in a quiescent state. Owing to their physicochemical properties, the highly hydrophilic LEA proteins could act as chaperons to protect macromolecular structures against desiccation injury [4]; for a review, see [5]. Besides its crucial role on genetic factors modulating seed maturation, ABA exerts an inhibitory effect on mechanisms that trigger seed germination by impeding the expression of germination-related genes. This is important from a physiological point of view because the ability of seeds to germinate (germination potential) builds during embryo development, well before the beginning of the desiccation phase [6]. This inhibitory mechanism allows sustaining the maturation process on the mother plant and the formation of a seed endowed with appropriate reserves required for establishment of a vigorous plantlet following seed germination.
The mature dry seeds of most species require a period of dry storage, known as after ripening, to release from dormancy (a physiological state in which the seed will not germinate even in optimal conditions [7]) and genetic and environmental factors must adjust to determine the potential for germination. Seed germination is traditionally described as corresponding to a process including three phases of water intake during which the seed imbibes (phase 1) and re-initiates metabolic processes (phase 2 also called germination sensu stricto) before the emergence of the radicle through the seed envelopes (phase 3) [6–8]. Phase 2 is a key developmental stage during which decision is taken as to whether continue toward radicle emergence, hence plantlet establishment, or if a dormant state not leading to the completion of germination must be maintained, for example if inappropriate environmental conditions are encountered.
To counteract the inhibitory effect of ABA on germination, after imbibition the seed will i) establish a specific catabolism to reduce the level of this hormone [9] and ii) synthesize another class of hormones, the gibberellins (GAs), which are activators of germination [10,11]. The GAs, which are represented by a large family of tetracyclic diterpenes, are essential to the germination of seeds. Thus, in plants such as Arabidopsis (Arabidopsis thaliana) or tomato (Lycopersicon lycopersicum) GA-deficient mutant plants are unable to germinate without the contribution of exogenous GAs [12,13]. It seems that GAs negatively regulate proteins involved in the repression of germination, as the transcriptional regulators called GRAS involved in cellular differentiation. In other words, GAs act as an “inhibitor of an inhibitor” [14]. In Arabidopsis, the DELLA subfamily of GRAS gene regulators includes GAI (GA Insensitive), RGA (Regulator of GA response), RGA-LIKE1 (RGL1), RGL2 and RGL3. All these proteins contain the DELLA motif, a conserved amino acid sequence in the N-terminus of these proteins. Among them, RGL1 and RGL2 are involved in the germination process [15]. The action of GAs is regulated through the ubiquitin-dependent proteasome [16,17]. In this context, three mechanistically distinct small molecules that inhibit Arabidopsis seed germination (methotrexate, 2,4-dinitrophenol, and cycloheximide) were recently identified through a small-molecule screen and used to probe the germination transcriptome. This analysis identified numerous germination regulators as being germination responsive, including the DELLA proteins GAI, RGA, and RGL3, the ABA-insensitive proteins ABI4, ABI5, ABI8, and FRY1, and the GA receptor GID1A [18].
Consistent with this idea that the ABA/GA ratio plays a role in regulating the metabolic activity required for germination, imbibed dormant seeds (which will not therefore germinate [7]) are not able to establish the catabolic pathway of ABA degradation and accordingly retain high levels of this germination inhibitor [9].
With the advent of post-genomics technologies, high throughput analyses of expression profiles were implemented at the RNA (transcriptome), proteins (proteome) and metabolites (metabolome) [19] levels to unveil specific features of the complex germination process (for a review, see [20]). These studies have shown that the physiological changes observed during germination are subject to both transcriptional and post-transcriptional control. It appears that a control at both levels is essential for seeds to adapt to their fluctuating environment and maximize the efficiency of germination.
2 Translation plays a major role in seed germination
De novo transcription is not required for completion of seed germination, as inferred from the observation that radicle protrusion through the seed coats can occur in Arabidopsis following imbibition of mature non-dormant seeds in the presence of α-amanitin, a highly potent and specific inhibitor of DNA-dependent RNA polymerase II [21]. The speed and uniformity of germination were nonetheless strongly affected under these conditions, implying that de novo transcripts must be synthesized during germination to increase germination vigor, notably those encoding enzymes and proteins required for the biosynthesis of GAs and/or those involved in the sensitivity of germination to this hormone. In contrast, the germination of Arabidopsis seeds proved to be totally blocked in the presence of cycloheximide, an inhibitor of translation [21]. These results demonstrated the need for protein synthesis for the completion of germination, particularly from the RNA stored in the seeds as templates. All these results highlighted the role of RNA and protein stored in seeds during their maturation on the mother plant and showed that in Arabidopsis, the potential for seed germination is largely programmed during the maturation process.
The major role of translation in the germination process has also been identified in other studies. Thus, transcriptomic analysis confirmed the massive participation of mRNAs stored in Arabidopsis mature seeds during germination [22]. Also, this study underlined the requirement of protein synthesis during the early stages of germination, as indeed among the 2 to 3% of genes showing the strongest expression, those encoding ribosomal proteins, translation initiation and elongation factors were particularly evident [22].
Recent proteomic analysis of sugarbeet (Beta vulgaris L.) seeds led to the identification of 758 proteins allowing inferring and reconstructing in detail their metabolic status [23]. As in Arabidopsis, the mature sugarbeet seeds appeared to be well prepared to mobilize during germination the major classes of reserves that are the proteins, triglycerides, phytate and starch, indicating that preparation to germination is mainly achieved during seed maturation on the mother plant. Furthermore, this study identified a number of proteins never described in seeds. Intriguingly, the data revealed the opportunity for the sugarbeet seeds to initiate translation either through the traditional cap-dependent mechanism or by the alternative cap-independent process. It is known that at some points of a cell's life, for example during mitosis, cell stress, or viral infection, the standard mechanism of translation initiation via cap-recognition at the 5′-end of cellular mRNAs is compromised, but at these times, some mRNAs use an alternative cap-independent form of initiation as a congregation site for initiation factors [24]. These findings suggest a model in which the two different mRNA pools existing in germinating seeds, namely the stored mRNAs and the de novo synthesized mRNAs, make use of these two different initiation systems to allow their respective recruitment during germination [23]. Consistent with this, stored mRNAs were shown to be efficiently translated via a cap-independent mechanism during maize (Zea mays) embryonic axes germination [25].
3 Commitment to germination
It is not clear yet what are the signaling pathways that are at the intersection between underlying the decision of to maintain the state of dormancy or initiate radicle protrusion. Two important questions are whether germination is an irreversible phenomenon once non-dormant seeds are imbibed and at what time is the decision taken to commit the germinating seeds to the establishment of seedlings. Recent data provided new information about these issues, showing that the germination process and the interconversion between dormant and germination-competent states were far more complex than imagined.
3.1 The maturation program can be recapitulated during early stages of germination
Several physiological studies established that the development of a germinating embryo into an autotrophic seedling could be arrested under conditions of water deficit. This growth arrest was shown to correspond to an ABA-dependent checkpoint of germination involving the transcription factor ABI5 [26]. Genetic analyses disclosed that the embryogenic ABI3 factor, which acts upstream of ABI5 and that is essential for the expression of ABI5, was also required to promote this growth arrest. Since the activity of ABI3 is essential at the end of the maturation phase, its accumulation during the early stages of germination in the presence of ABA or an osmotic stress reflects a reinduction of developmental processes normally taking place at the end of maturation. In other words, this initial phase of germination, up to the ABI5-dependent checkpoint, is a reversible process in which the maturation program can be recapitulated. Based on the known function of the genes controlled by ABI3 during seed maturation, including those encoding LEA proteins, Lopez-Molina et al. [26] proposed that the formation of embryos arrested in their progress toward germination corresponds to an adaptive mechanism that increases the survival of seeds under conditions of water stress encountered in the soil. Arabidopsis seeds germinated in the presence of α-amanitin were also shown to reset the maturation program, as the de novo synthesis of LEA proteins and storage proteins (cruciferins) was observed in such conditions [21]. The possibility of recapitulating the late maturation program at two levels, the transcriptional [26] and translational [21,27] levels, would allow rapid adjustment of the response of imbibed seeds confronted with rapid fluctuations of environmental conditions in the soil (e.g., soil water content).
3.2 The action of GAs occurs late during seed germination
A proteomic study of seeds from the Arabidopsis ga1-deficient mutant, which is GA deficient, demonstrated a very late implication of GAs in seed germination, occurring at a stage coinciding or being very close to radicle emergence (which marks the end of germination sensu stricto) [28]. This study disclosed that this hormone, although being required for the completion of germination, is not directly involved in many processes taking place during the germination sensu stricto (phases 1 and 2 of germination) as, for example, the initial mobilization of proteins and lipids stored in the mature seed. This behavior was confirmed by a study of the germination of Arabidopsis seeds combining measurements of endogenous GA levels by GC-MS and transcriptomic analyses of GA-regulated genes [29]. Moreover, these two studies revealed a role of GAs in preparation for cell division, an event occurring late in the germination process. Consistent with this, a recent transcriptomic analysis showed that the activation of the cell cycle in the Arabidopsis root meristem precedes the penetration of the seed envelopes by the radicle and that D cyclins are limiting factors for this process [30].
3.3 A final control point in the germination process occurs between the action of GAs and the radicle protrusion step
A recent publication indicated that establishment of GA action in imbibed seeds does not probably correspond to the final stage of germination sensu stricto, before the germinated seed is irreversibly committed to seedling establishment and growth. Thus, Carrera et al. [31] showed that COMATOSE (CTS), a single copy gene encoding an ABC carrier involved in the mobilization of reserve lipids [32], was required for germination. The phenotype of the cts mutants is an inability to germinate and, not surprisingly, their protein profile resembles that of dormant seeds, which led Russell et al. [33] to describe the seeds of this mutant as forever-dormant. However, contrarily to expectation the transcriptomic profile of the imbibed cts seeds strongly resembles that of imbibed non-dormant seeds [31]. In other words, the cts mutant seeds can manage to leave their dormant sate though they are unable to germinate. This led to the assumption that CTS has a direct role in germination, just before the emergence of the radicle, rather than inhibit dormancy release during dry storage of dormant seeds (after-ripening) [31]. More precisely, a comparison of the transcriptome of imbibed cts mutant seeds with that of wild-type non-dormant seeds incubated in the presence of GAs [29] showed that the function of CTS is required after the establishment of the mode of action of GAs during germination [31].
4 A model to account for the different checkpoints occurring during seed germination up to radicle emergence
Based on these various reports, a model can be elaborated to describe the various control points occurring up to seedling establishment (Fig. 1). Briefly, primary dormancy (PD in Fig. 1) is acquired during seed development on the mother plant. A set of appropriate environmental conditions (for example, dry storage) is required to remove this primary dormancy, which leads to the non-dormant state represented by AR (“after-ripened”). The non-dormant seeds germinate if favorable environmental conditions are met. In the presence of an osmotic stress, for example generated by limiting water in the soil, the seeds having reached the checkpoint involving ABI5 (represented by “Check” in Fig. 1) can be reversibly recycled toward the AR state allowing them to mount appropriate defense reactions until favorable conditions for germination are finally encountered. Such defense reactions could for example allow the imbibed AR seeds to maintain desiccation tolerance [34] and/or trigger repair mechanisms [35].
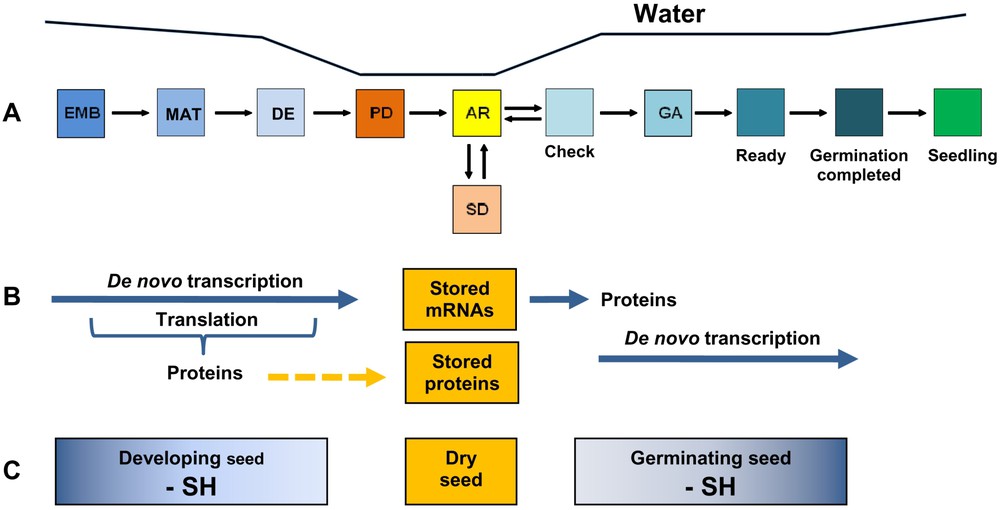
The various checkpoint control stages during germination leading to seedling establishment. (A) A model describing the various checkpoint control stages, adapted from [20]. PD, primary dormancy; SD, secondary dormancy; AR (“after ripened” seeds) non-dormant seeds; Check, control checkpoint involving ABA and a possible reinduction of the maturation program; GA, biosynthesis of GAs and expression of GA-regulated germination genes; Ready, expression of the CTS gene, final step before radicle emergence; Germination completed, radicle emergence; Seedling, establishment of the seedlings. Developmental stages leading to the mature dry seeds are also shown: EMB, embryogenesis; MAT, maturation; DE, desiccation. The evolution of seed water content during development and germination is schematized on top (water content). (B) Transcription and translation during seed development and germination. During embryo formation and maturation protein synthesis proceeds through translation of de novo synthesized mRNAs. In contrast, during germination protein synthesis proceeds through translation of both stored mRNAs accumulated during seed maturation and de novo synthesized transcripts [21]. The completion of germination does not require de novo transcription and can solely proceed from proteins stored in the dry mature seed and the proteins synthesized from stored mRNAs [21]. De novo transcription during imbibition is required for increasing the rate and uniformity of seed germination as well as for seedling establishment [21]. (C) Redox changes occurring in the proteome during seed development and germination, according to [36]. The exact developmental steps at which the redox transitions occur between the developmental stages corresponding to developing, dry and germinated seeds are not yet known.
It can be hypothesized that if environmental conditions are too severe to allow germination in a reasonable period of time (e.g., a few days), seeds will enter into secondary dormancy [7] (SD, Fig. 1) to reacquire a state of quiescence allowing them to preserve their stored reserves for a next attempt of germination. However, if environmental conditions become favorable, this dormancy can be released again to accomplish germination through the AR state (Fig. 1). It is likely that such cycles can be repeated provided that the seed can maintain/reconstitute its pool of reserves mobilized over the successive non-productive germination attempts. When the seeds are able to cross the “Check” stage, they can establish the GA biosynthesis pathway and express the GA- dependent genes to activate radicle protrusion (GA stage in Fig. 1), for example the genes of the cell cycle [28–30]. The final step before the emergence of the radicle corresponds to CTS gene expression (“Ready” in Fig. 1), which is required for the establishment and survival of the seedlings [31].
5 Post-translational control of seed germination via redox signaling
A growing body of evidence indicates that the germination of seeds is accompanied by extensive change in the redox state of proteins. In cereals, proteins of both the starchy endosperm and embryo that are present mainly in the oxidized (S-S) form in the dry seed are converted to the reduced or sulfhydryl (-SH) state following imbibition [36]. The regulatory disulfide protein, thioredoxin (Trx), appears to play a central role in this redox conversion. When reduced enzymatically in the presence of NADPH, Trx acts as a signal early in germination to facilitate the mobilization of reserves by (1) reducing storage proteins, enhancing their solubility and susceptibility to proteolysis; (2) reducing and inactivating disulfide proteins that inhibit specific amylases and proteases, thereby facilitating the breakdown of stored starch and proteins; and (3) reductively activating individual enzymes functional in germination (Fig. 1) [36]. Recently, this question was investigated during germination of seeds of a model legume, Medicago truncatula [37]. The data provided evidence that Trx functions in the germination of seeds of dicotyledons as well as monocotyledons. In summary, it can be proposed that the oxidized form of the proteome in mature dry seeds accounts for metabolic quiescence; this proteome is activated during germination by reduction of protein disulfide bridges in the presence of specific Trxs (Fig. 1). Given the large number of proteins affected (more than 100) [37], such post-translational processes would provide simple yet very efficient means to control complex processes important for seed germination. It follows that the question of the targets of such a post-translational modification becomes an increasingly important question, as they might be important regulators of cellular activity following imbibition.
Another oxidative modification of proteins results from protein carbonylation by reactive oxygen species (ROS). ROS are involved in a variety of diseases, including aging, Alzheimer's, Parkinson's, cancer and heart disease. A proteomic study showed that various carbonylated proteins accumulate during imbibition of Arabidopsis seeds [38]. This oxidation damage was not evenly distributed among seed proteins and targeted specific metabolic enzymes, translation factors, and several molecular chaperones. Although accumulation of carbonylated proteins is usually considered in the context of aging in a variety of model systems, this was clearly not the case for the Arabidopsis seeds since they germinated at a high rate and yielded vigorous plantlets. The results indicate that the observed specific changes in protein carbonylation patterns are probably required for counteracting and/or utilizing the production of ROS caused by recovery of metabolic activity in the germinating seeds. Accordingly, this study proposed that carbonylation of seed storage proteins occurring during seed development would help facilitating their mobilization during germination [38].
6 Compartmentalization of seed metabolism
A recent proteomic study of sugarbeet seeds disclosed that the seed proteome exhibits tissue-specific features [23]. This study unveiled a compartmentalization of metabolic activity between the radicle, cotyledons and perisperm, indicative of a division of metabolic tasks between the various tissues. These data support the results of Gallardo et al. [39] showing that in developing Medicago truncatula seeds sulfur metabolism is partitioned between seed coats and the embryo, and the results of Koller et al. [40] documenting the existence of divergent regulatory mechanisms in starch biosynthesis and degradation in different rice (Oryza sativa) tissues.
7 Epigenetic control of seed germination
In addition to the different mechanisms discussed above, recent data documented the importance of chromatin remodeling in the germination process [22]. For example, a well-studied chromatin-remodeling factor that is expressed in seeds is PICKLE (PKL). PKL represses the expression of embryonic traits during germination [41–43]. Also, histone deacetylases, which are chromatin-remodeling factors contributing to transcriptional repression in eukaryotes, were shown to regulate during germination the repression of embryonic properties directly or indirectly via repression of embryo-specific gene functions [44,45]. Interestingly, recent data also documented a role for chromatin remodeling in seed dormancy [46].
8 Concluding remarks
Post-genomics studies have provided important new information about mechanisms controlling germination. A major control seems to be exerted at the translational level. Future studies will illuminate the identification and characterization of transcripts required to be translated following imbibition, notably in the view that germinating seeds are able to initiate translation either through the traditional cap-dependent mechanism or by the alternative cap-independent process. Furthermore, in future work, it would be of interest to investigate more thoroughly the role of redox modifications on the expression/activity of the proteome in developing and germinating seeds, notably in relation with seed vigor. It is noted that most transcriptome and proteome studies have used whole seeds. Yet, our recent work highlighted the need to examine expression patterns within different tissues and compartments of germinating seeds [23]. Growing evidence unveiled the major role of epigenetic control during the progress of germination, notably for the repression of embryonic traits during this process.
Acknowledgements
The PhD thesis of Julie Catusse was supported by a French–German joint program in plant genomics (Génoplante-GABI).