1 Introduction
Desiccation-tolerant organisms (anhydrobiotes), such as pollen and seeds, are capable of surviving the complete removal of their cellular water. Apart from the ability to tolerate this desiccation, they are also able to survive in the dry state for extended periods of time. The lifespan of seeds can be remarkably long, ranging from decades to centuries [1–3] and even millennia [4]. In the 1980s, Burke [5] discussed the possibility that the cytoplasm of drying seeds could enter into a glassy state as a defense mechanism. A glass is defined as an amorphous metastable state that resembles a solid, brittle material, but retains the disorder and physical properties of the liquid state, as indicated in Fig. 1 [12]. In other words, a glass is a highly viscous solid liquid. Its high viscosity has been shown to slow down severely molecular diffusion and to decrease the probability of chemical reaction – for reviews see [7,8]. Burke suggested that in dry anhydrous organisms, glasses could be formed from cell solutes like sugars that were known in food science to provide protection from denaturation of large molecules and formation of molecular aggregates. Also, it was proposed that glasses might fill spaces in a tissue during dehydration and that their high viscosity may stop all chemical reactions requiring molecular diffusion [5]. Examples of well-known molecules that can undergo glass transitions can be found in food and polymer sciences. They include sugars, proteins, starch, complex food systems, and a wide range of polymers [6–8]. The glassy state is known to confer stability to many of these model systems. Thus, the glass concept turned out to be an interesting hypothesis to account for the survival in the dry state. Subsequently, efforts focused on detecting glasses in plant tissues and investigated its physiological importance in desiccation tolerance and storage longevity and on the assessment of physical properties, such as molecular mobility and density of the intracellular glassy matrix [9]. This review will discuss the physiological functions that these biological glasses exert in plant anhydrobiotes as well as the peculiar properties of intracellular glasses compared to model glasses. For information on the characteristics of glasses, we refer the reader to more detailed reviews on glasses in model systems [6–9].
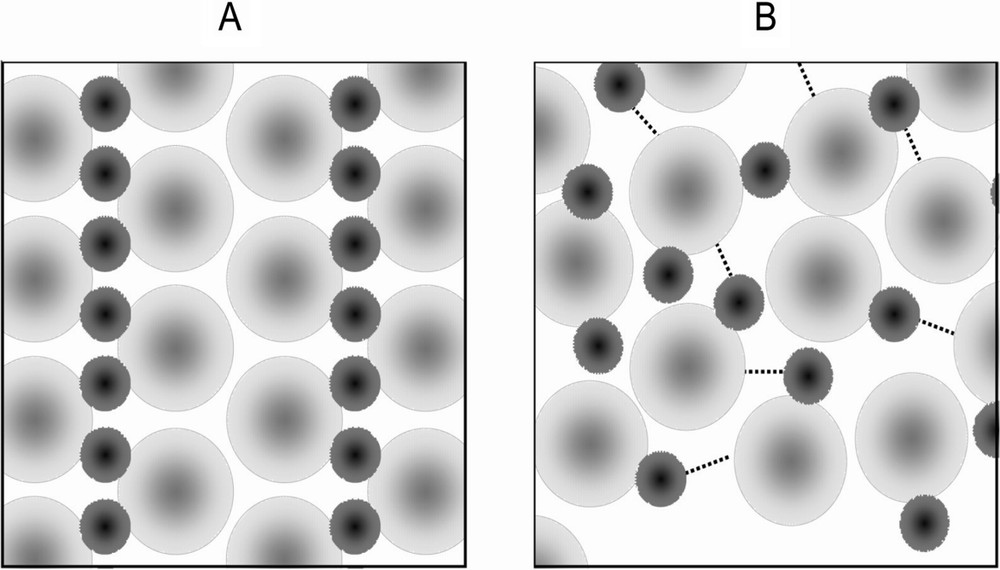
Schematic 2-dimensional diagram of a crystalline structure (A) and glassy state (B) composed of two types of molecules. The crystalline structure is regular and symmetrical, whereas there is no particular orientation to the molecules in the glass. The lines between the molecules represent hydrogen bonding or ionic interactions in the glassy state.
2 Drying results in intracellular glass formation
Intuitively, one can imagine that as the concentration of solutes increases, the viscosity of the solution increases, resulting in a decrease in molecular mobility of molecules. The same is true for the cytoplasm of seeds upon drying. To measure this viscosity or mobility in the cells electron paramagnetic resonance (EPR) spectroscopy proved to be an excellent technique to study molecular mobility in vivo in biological samples [10,11]. After incorporation of spin probe molecules into the tissues and removal of the extracellular signals by the addition of potassium ferricyanide, this technique can provide a spectrum of the spin probe from the cytoplasm. From the spectral line shapes, a measure of molecular mobility can be derived [11]. This rotational mobility can be taken as a measure for the intracellular viscosity of the environment in which the spin probe resides. An example of this kind of measurement is shown in Fig. 2. Using 3-carboxy-proxyl, a polar nitroxide spin probe with the size of approximately a glucose molecule, the rotational mobility in pea (Pisum sativum L.) axes was determined during drying at room temperature [11]. In the embryonic tissues below 0.8 g H2O/g DW, the cytoplasm becomes increasingly viscous. The removal of water induces a supersaturation of the cytosolic components leading to an increase in the cohesive forces between molecules and restriction of the molecular mobility within the cytoplasm. Furthermore, drying below 0.3 g H2O/g DW leads to a decrease in the molecular mobility in the cytoplasm of over five orders of magnitude (Fig. 2). Finally, at around 0.1 g H2O/g DW, the cytoplasm vitrifies and enters into the glassy state. Glass formation has been detected in seeds [13–16], pollen [17] and the resurrection plant Craterostigma plantagineum (J. Buitink, unpublished results). Apparently, glass formation is a characteristic that can be found in all desiccation-tolerant tissues. The water content at which the cytoplasm transforms into a glassy state during drying depends on the temperature. The lower the temperature of drying, the higher the cellular water content at which the cytoplasm becomes glassy. From the water content/
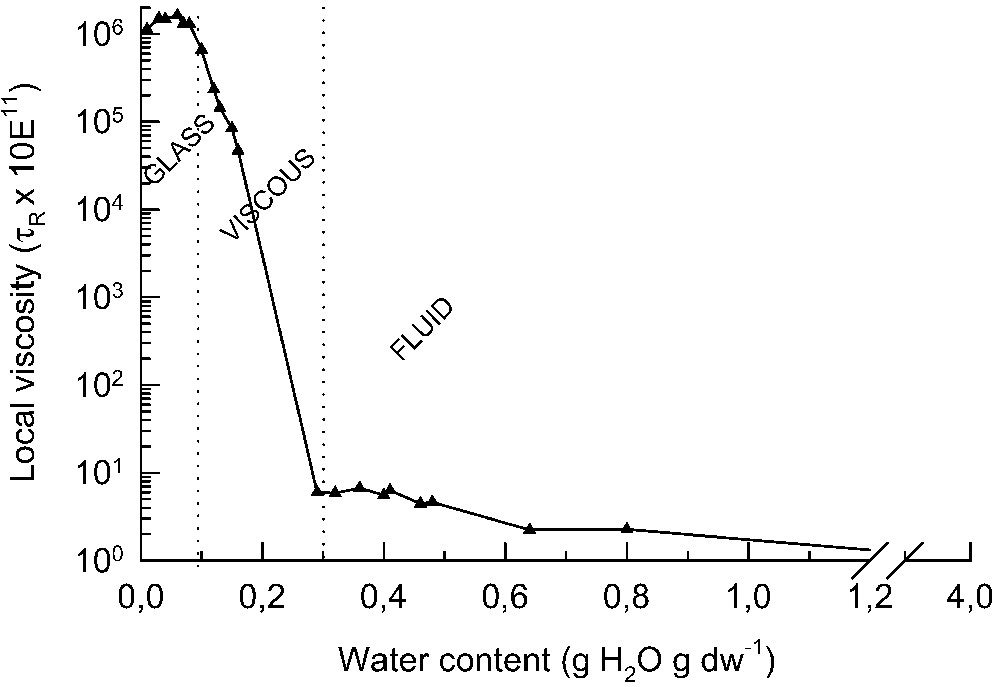
Increase in molecular viscosity in embryonic axes of Pisum sativum during drying at 20 °C. Local viscosity is expressed as the rotational correlation time
Increase in molecular viscosity in embryonic axes of Pisum sativum during drying at 20 °C. Local viscosity is expressed as the rotational correlation time
Water content and corresponding minimum equilibrium relative humidity to be reached to form a cytoplasmic glass at 25 ± 2 °C for various crops containing different amount of storage oil
Species | Oil content (% DW) | Water content (% FW) | Relative humidity (%) |
Glycine max | 19.6 | 9.0 | 47 |
“ “ cv. Dunfield | 20.9 | 9.0 | 49 |
“ “ cv. Peking | 17.6 | 9.0 | 46 |
Pisum sativum | 1.2 | 10.3 | 44 |
Phaseolus vulgaris | 1.0 | 10.3 | 44 |
Zea mays (embryo) | 8.0 | 10.2 | 48 |
Although glass formation in seeds drastically decreases molecular mobility, the molecules in a glass are not completely restricted in their movement. In time, diffusion will be possible, albeit at a rate presumably considerably slower than that in hydrated cytoplasm. This explains why seeds still age, because deteriorative processes such as lipid oxidation can take place, though at a very slow rate. Another example of this is the natural after-ripening process taking place in dry seeds after harvest, during which seeds escape dormancy (reviewed in [18]). Indeed, Walters [19] demonstrated using theoretical considerations coupled to measurements of relaxation times that mobility is not restricted until at least 70 °C below the glass transition temperature, which corresponds to
3 Importance of the glassy state for desiccation tolerance
Intracellular glasses were originally suggested to confer desiccation tolerance. Williams and Leopold [20] showed that the
4 Role of the glassy state for seed longevity
One of the most studied functions of glasses is preservation of the structural and functional integrity of macromolecules [7,8]. Glasses are known to decrease detrimental reactions, such as the rate of browning reactions [23], to increase the stability of enzymes [24] and to prevent conformational changes of proteins [25]. The stabilizing effect of glasses on macromolecular and structural components during storage makes it likely that glasses play an essential role in the longevity of seeds and pollen. Indeed, the secondary structure of proteins in dry seeds of several species appears to be very stable and remains preserved after several decades of open storage. For example, no protein aggregation and denaturation was found after 28 years of dry storage of wheat (Triticum spp.) seeds, despite loss in viability [26]. Aging rates of plant anhydrobiotes are strongly dependent on the water content and temperature of storage, conditions that determine glass formation [27]. Furthermore, a link with the intracellular glass properties under the same storage conditions has been well established [28–30]. Typically, seed and pollen deterioration is accelerated when the tissues are not in a glassy state [28,31]. Conditions that bring the anhydrobiotes above their
5 Intracellular glasses have peculiar properties that confer extreme stability to the seeds
The consequences of being in or near the glassy state gained further physiological significance with a study on the molecular mobility in desiccation-tolerant tissues indicating that the cytoplasm remains quite immobile even when the glass has melted, as measured by DSC [33]. In model systems, it has been observed that melting of the glass increases the molecular mobility. However, only at a slightly higher temperature, the collapse temperature (
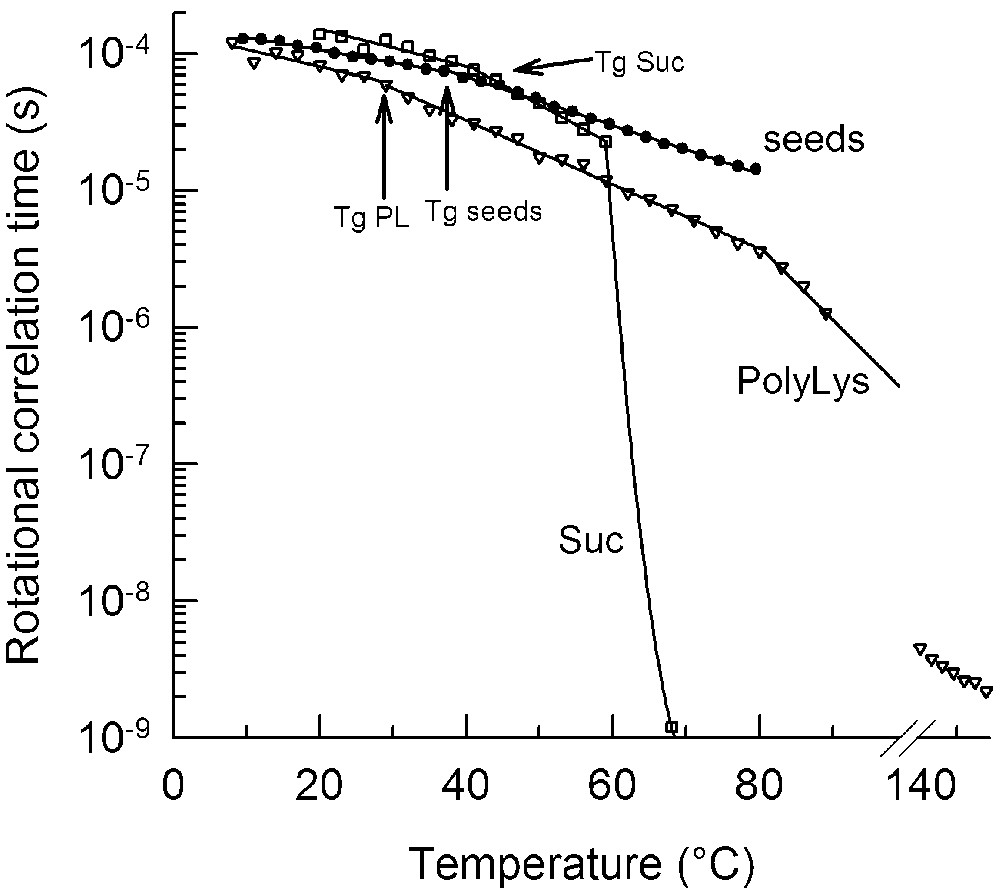
Temperature dependence of the rotational correlation time (
Temperature dependence of the rotational correlation time (
6 Composition of intracellular glasses
For a long period of time sugars were considered to play an important role in the composition of the glassy state in seeds. Indeed, sugars are present in large amounts in desiccation-tolerant tissues and are known to be excellent glass formers. The correlation between oligosaccharides and longevity [35,36] and the knowledge that oligosaccharides increase
7 Role of LEA proteins in intracellular glass formation
Proteins that are thought to be implicated in intracellular glasses are those from the LEA (late embryogenesis abundant) class, a family of highly hydrophilic proteins that are heat-soluble [21,36,44]. Considering that during maturation, seeds and pollen accumulate both non-reducing sugars and LEA proteins [45], it is thought that both types of molecules interact together in the formation of a glassy state. That these molecules interact was demonstrated in a study on the hydration characteristics of heat-soluble proteins extracted from mature wheat embryos. It was shown that after protein purification, the extract still contained sugars at about 1:1 (w:w) ratio. Only about half of the sugars could be removed by exhaustive dialysis and the rest appeared to be tightly associated with the proteins [46]. Wolkers et al. [44] showed the hydrogen bonding strength of a sucrose/LEA mixture is higher than that of a sucrose glass alone. More research is needed to confirm the implication of LEA proteins in biological glasses or elucidate whether other types of proteins play a role in the glass formation. Yet, the advantage of a combination of sugars and LEA proteins in the glass mixture would be that both types of molecules exhibit several and distinct functions, all being important for the preservation of structural functionality. Considering that LEAs are a heterogeneous class of proteins, the question as to whether there are specific LEA proteins involved in determining glass properties in dry anhydrobiotes remains to be addressed. Using a proteomic approach, a subset of LEA proteins have been identified in relation to survival in the dry state [47], making them possible candidates for the stabilization of the glassy state. Besides a putative role in the dry state, LEA proteins are also known to confer tolerance to osmotic and cold stress. The protective mechanisms behind this action are described in details in a recent review on LEA proteins [48].
8 Concluding remarks
The concept of a glassy state applied to seeds has greatly improved our understanding of anhydrobiosis. It provides a global explanation as to why metabolism is arrested in the dry state, why seeds gain so much stability after drying and deteriorate fast when moisture and temperature increase during storage. Also the characterization of the dry cytoplasm at the molecular level near and below
Acknowledgements
This work was supported in part by grants from the Ministère de l'Agriculture et de la Pêche and from the region Pays de la Loire (COSAVE).