1 Introduction
Most ecosystems on Earth depend on photosynthetic primary production for the transformation of inorganic carbon into organic compounds and its entry into food chains. Because light does not penetrate very deep in the seawater, very low biomasses occur at the seafloor where organisms depend upon the meteoritic input of sinking organic matter. However, high biomasses of invertebrates were discovered in the vicinity of deep-sea hydrothermal vents in the late 1970s, and later, on cold seeps, raising questions about how inorganic carbon was converted into organic matter at high rates in these ecosystems. The existence of symbioses between the dominant fauna and bacteria was proven to be the key adaptation explaining the high biomasses observed. Many invertebrates indeed live in association with bacteria which ensure a light-independent type of primary production named ‘chemoautotrophy’ [1,2]. Chemoautotrophic symbionts use reduced compounds, such as hydrogen sulphide present in the environment, as electron donors and oxygen as an electron acceptor to generate large amounts of energy required to fix inorganic carbon, providing a source of carbon to their metazoan host. The co-occurrence of energy-rich electron donors and oxygen is unusual because reduced compounds normally get oxidized in oxygenated seawater. However, the presence of hydrothermal vents and cold seeps is linked with processes generating huge amounts of reduced compounds. Briefly, hydrothermal vents result from geothermally-driven circulation of seawater in the crust linked to magmatic activity at oceanic ridges, and cold seeps occur where hydrocarbons and/or gas hydrates are present in the subsurface at continental margins. Both environments are characterized by emissions of fluids enriched in reduced compounds originating from the subsurface such as hydrogen sulphide, methane and dihydrogen which fuel diverse chemotrophic prokaryotes (for reviews see [3–5]).
Invertebrates associated with chemotrophic symbionts include mostly bivalves (within families Lucinidae, Mytilidae, Solemyidae, Thyasiridae, and Vesicomyidae), gastropods (Provannidae), annelids (Alvinellidae, Siboglinidae) and arthropods (Alvinocarididae) (for reviews, see [6] and [7]). Among these groups, mussels of the subfamily Bathymodiolinae (Bivalvia: Mytilidae) are of particular interest for several reasons. They are dominant at many vents and seep sites with species reaching lengths up to 40 cm, and smaller species (up to 3 cm) are also found at large falls of decaying organic matter such as sunken wood and whale bones, where hydrogen sulphur is present as a by-product of the degradation of organic matter [8]. Mussels attach by their byssal threads to hard substrates just above the interface between bottom seawater and sediment or rocks. Most of the species harbour chemoautotrophic sulphur-oxidizing symbionts, as do many other invertebrates cited above, but some also display chemotrophic methane-oxidizing bacteria, and some display multiple symbioses within their gill tissue [9–11]. Bathymodiolines thus constitute good models to study the functioning and evolution of associations involving multiple partners. Symbiosis is important in the nutrition of mussels, as shown by the reduction of the gut observed in some species, but filter feeding can also play a role [12,13]. Information is scarce regarding the physiology and metabolism of bacterial symbionts due to the unability to cultivate them, but numerous studies have investigated various aspects of mussel symbioses. In this article we summarize the current knowledge about the phylogeny of mussels and their symbionts, and the diversity and role of bacteria. Trends in the evolution of mussel symbioses are discussed, focusing on mussel species for which information is available for both host and symbionts (Table 1). New TEM (Transmission Electronic Microscope) observations and sequence data obtained from small mussels associated with sunken woods collected around the Philippines are included.
Species of Bathymodiolinae presented in this paper.
Area | Species name | HAB | Sites | Symbionts | Reference for symbionts | Accession number | Reference for hosts | Accession number |
Atlantic | B. azoricus | H | Menez Gwen, Lucky Strike, Rainbow, Broken Spur (Mid-Atlantic ridge) | M, S | [36] | AM083950 & 74 | [17] | AY649795 |
B. puteoserpentis | H | Broken Spur, Snake Pit, Logatchev (Mid-Atlantic ridge) | M, S | [36] | AM083950 & 74 | [17] | AY649796 | |
B. cf. boomerang | S | Regab (Gulf of Guinea, Gabon continental margin) | M, S | [26] | AJ745717-8 | [74] | DQ513451 | |
B. heckerae | S | West Florida Escarpment (Gulf of Mexico), Blake Ridge | M, S1, S2, My | [75] | AM236325-8 | [17] | AY649794 | |
B. brooksi | S | Alaminos Canyon, Atwater Canyon (Gulf of Mexico) | M, S | [75] | AM236330-1 | [17] | AY649797 | |
“B.” childressi | S | Alaminos canyon, Lousiana Slope (Gulf of Mexico) | M | [70] | AM236329 | [17] | AY649800 | |
Eastern Mediterranean | Idas sp. | S | Central Province | M, S1, S2, My, G, CFB | [11] | AM402955-60 | [11] | EF210072 |
Eastern Pacific | B. sp. | H | Juan de Fuca Ridge | S | [32] | DQ077893 | [32] | DQ077892 |
B. thermophilus | H | East Pacific Rise, Galapagos Ridge | S | [64] | M99445 | [76] | AF456285 | |
Western Pacific | “B.” japonicus | H, S | Sagami Bay, Okinawa Trough | M | [77] | AB036711 | [15] | AB101423 |
“B.” platifrons | H, S | Sagami Bay, Okinawa Trough | M | [77] | AB036710 | [15] | AB101421 | |
B. septemdierum | H | Suiyo Seamount, Myojin Knoll | S | [77] | AB036709 | [15] | AB101430 | |
B. brevior | H | Lau Basin, Central Indian Ridge (Indian ocean) | S | [78] | DQ077891 | [17] | AY649799 | |
MOTU 16 | W | Bohol sea (8.815°N, 123.627°E, 357–372 m depth) | S | this study | AM931532 | this study | EU350070 | |
A. longissima (BC 279) | W | Bohol sea (9°25.6′N, 124°02.1′E, 1750–1763 m depth) | S | [29], BC 279 | AM851095 | this study | EU350072 | |
MOTU 5 (BC 294) | W | Bohol sea (9°20.9′N, 124°08.7′E, 1764 m depth) | S | [29], BC 294 | AM503922 | this study | EU350071 |
2 Phylogenetic relationships within the subfamily Bathymodiolinae
Bathymodioline mussels form a monophyletic group within the mytilid family. Following early work by Craddock and co-workers, detailed accounts of the phylogeny within the bathymodioline group using analyses of mitochondrial and nuclear genes have been proposed by groups led by Jones, Iwasaki et Miyazaki [14–17]. Three main clades of mussels are recognized in all studies cited above, but the relationships between these clades are poorly resolved (Fig. 1). The “childressi” clade includes among others the seep species “B.” childressi and two species occurring on both vents and seeps, “B.” japonicus and “B.” platifrons. Whether this group represents a new genus, different from Bathymodiolus is under discussion, and thus the genus name is noted between quotation marks [17,18]. The “thermophilus” lineage includes the vent species B. azoricus, B. brevior, B. puteoserpentis, B. septemdierum, B. thermophilus and the seep species B. cf. boomerang, B. brooksi and B. heckerae. Although weakly supported in the cytochrome c oxidase subunit I (COI)-based tree displayed in Fig. 1 (bootstrap value of 44), this clade is found in most studies based on COI, and is well-supported in phylogenies based on several genes including ND4 and 28S rRNA [16,17,19]. A third clade includes two vent species, B. aduloides and an undescribed species from Manus basin, but no information is available regarding their symbioses, and it is thus not displayed in Fig. 1 [17]. A study by Distel et al. has enlarged the diversity of habitats represented by including sequences from species associated with sunken organic substrates (woods, whale bones, shark teeth...) and showed that these mussels, known since the 19th century and classified in the genera Adipicola, Idas, Idasolas, and Myrina, clustered together with vent and seep species, although symbiosis was not investigated in his study [20]. The lack of resolution of 18S rDNA-based phylogenies did not allow one to clarify the relationships between lineages from organic substrates and those from seeps and vents, but authors suggested that ancestors of bathymodiolines were living in shallow water, and colonized deep-sea vents and seeps via intermediate forms living on sunken woods or organic debris [20]. This hypothesis received support from a recent study based on 4 genes including 7 species living on organic substrates, out of which 5 were found only on sunken wood. Two of these 5 species branched close to the root of the tree [21]. Sunken wood species displayed in the tree presented here cluster in distinct positions, with MOTU 5 appearing as a sister taxon to Idas sp. from the eastern Mediterranean, Adipicola longissima clustering in the multifurcation, and MOTU 16 branching in a relatively basal position in the tree, but with a low bootstrap value (Fig. 1). The most basal bathymodioline species in the tree is Bathymodiolus sp. from Juan de Fuca (JdF), but Benthomodiolus lignicola, for which no information is available regarding symbionts and which is thus not displayed, is more basal in published phylogenies [20]. Wood-associated mussels displayed here are thus neither early-emerging, nor monophyletic.
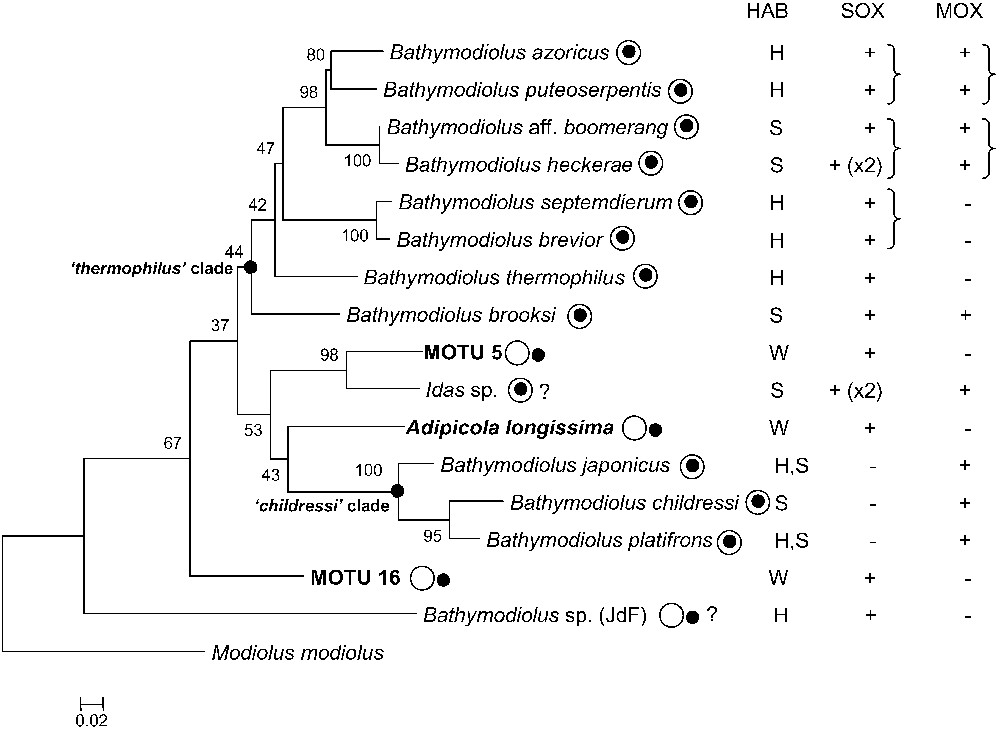
Phylogenetic reconstruction of bathymodioline species based on cytochrome c oxidase subunit I (COI) nucleotidic sequences. The tree was built using PHYML (GTR substitution model, estimated proportion of invariable sites, four categories of substitution rates, 500 bootstrap replicates) [73], and rooted on Modiolus modiolus. New specimens from this study appear in bold. Scale bar represents 2% estimated base substitution. Bold nodes represent the ‘thermophilus’ and ‘childressi’ clades (see text). The most terminal nodes are well supported, but their relationships are much less clear, due to the poor resolutive power of the COI. The localization of the black spot relative to the white circle indicates whether symbionts are intra- (within) or extracellular (outside). HABitat: hydrothermal vents (H), cold seep (S), sunken wood (W). The presence of sulphur- (SOX) and methane-oxidizing (MOX) symbionts is indicated, a parenthesis indicating that the symbionts are most closely related to one another based on their 16S rRNA sequence (see text). Masquer
Phylogenetic reconstruction of bathymodioline species based on cytochrome c oxidase subunit I (COI) nucleotidic sequences. The tree was built using PHYML (GTR substitution model, estimated proportion of invariable sites, four categories of substitution rates, 500 bootstrap replicates) [73], and ... Lire la suite
Molecular estimations suggest a divergence time between 23.7 and 74.3 MY for the bathymodioline group [17]. This is congruent with the age of several fossils of sunken wood- and whale fall-associated mussels belonging to the Idas genus recovered from the late Eocene (60 MY) to the Miocene (30 MY) and with the age of the oldest cold seep mussel, Bathymodiolus willapaensis, which was found in a geologic layer from the middle Eocene [22]. A recently-discovered fossil wood-fall community from the late Cretaceous did not yield mussels [23]. Unfortunately, the fossil record is still too patchy to really help answering whether mussels associated with organic substrates appeared first, but habitats in which mussels were found strongly suggest they already harboured bacterial symbionts. An origin around 60 MY could explain the absence of biogeographical patterns within lineages because at that time, the distribution of continental masses was different, allowing exchange between the future Pacific and Atlantic fauna.
3 Diversity and role of bacterial symbioses in bathymodiolines
3.1 Sulphur oxidizing symbiosis
Most bathymodioline mussels harbour chemoautotrophic, sulphur oxidizing, bacterial symbionts. Bacteria appear as ∼0.5 μm diameter inclusions displaying double membranes typical of Gram-negative bacteria [24]. In most mussel species, symbionts occur in vacuoles produced by the host cells and localized within specialized epithelial cells of the lateral zone of the gill filament, hence named bacteriocytes (Figs. 2 and 3) [25]. Vacuoles contain between one to several bacteria (for example, in B. cf. boomerang (Fig. 3E) and B. azoricus respectively [26,27]), and dividing bacteria were observed in most species analyzed to date (see Fig. 3E). Interestingly, recent work has shown that thiotrophs occur extracellularly in small mussels associated with sunken woods collected in the Bohol Sea (Philippines), namely morphotypes BC 279 (Adipicola longissima), BC 288, BC 294 (herein referred to as MOTU 5), BC 1007, and MOTU 16 (this study Fig. 3A, B, F, [28–30]). In these species, bacteria are located between long microvilli developed by bivalve cells throughout the lateral zone of each gill filament, and thickness of the bacterial layer depends upon the species and probably on local environmental conditions (Fig. 3) [29]. Similar extracellular bacteria were also reported from I. simpsoni from a whale skull, and I. washingtoniana and Adipicola sp. from the Juan de Fuca hydrothermal vents, although the thiotrophic nature of bacteria was only inferred from morphological evidence [31]. Two additional mussels with possible extracellular bacteria are Bathymodiolus sp. from the Juan de Fuca Ridge (vents), although results from TEM were ambiguous according to authors [32], and Tamu fisheri from the Gulf of Mexico (Hourdez, pers. comm.).
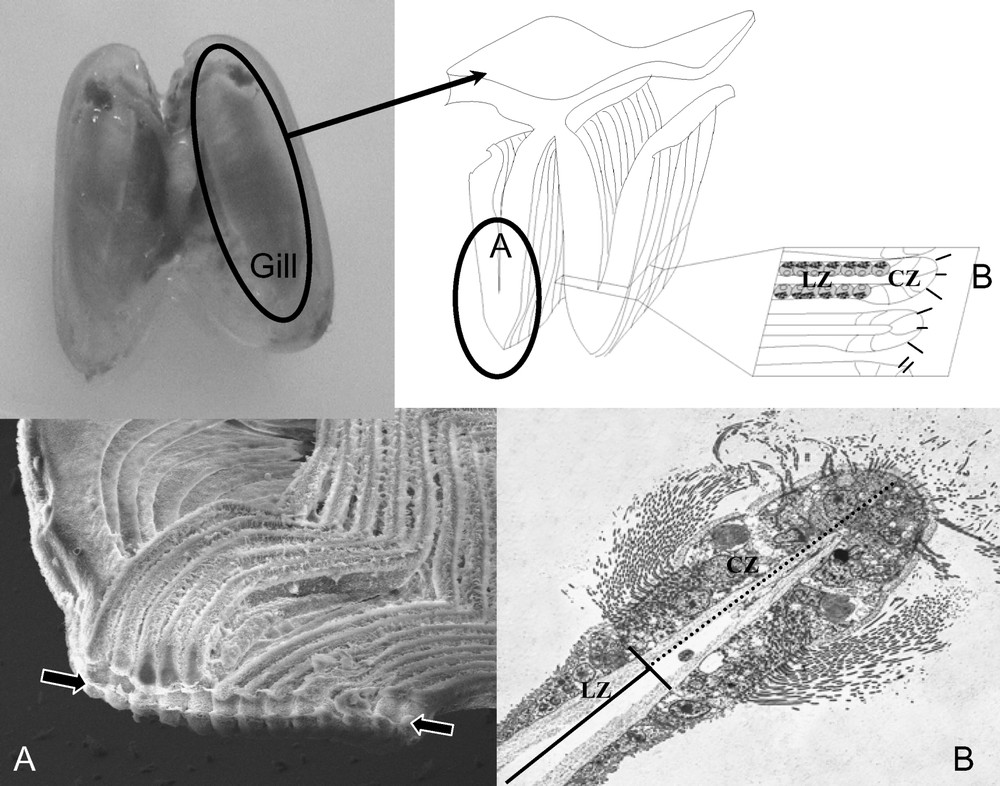
Localization of gill filaments, ciliary and lateral zones, and bacteriocytes in the gills of mussels using Electron Microscopy (EM). Up: overview of the gill displaying how sections used for microscopy are obtained. A: Scanning electron micrograph of gill filaments. In the bottom of the image is the food groove (arrows). B: Transverse section through a single gill filament displaying the ciliated zone (CZ) and the lateral zone (LZ) where bacteriocytes are located.
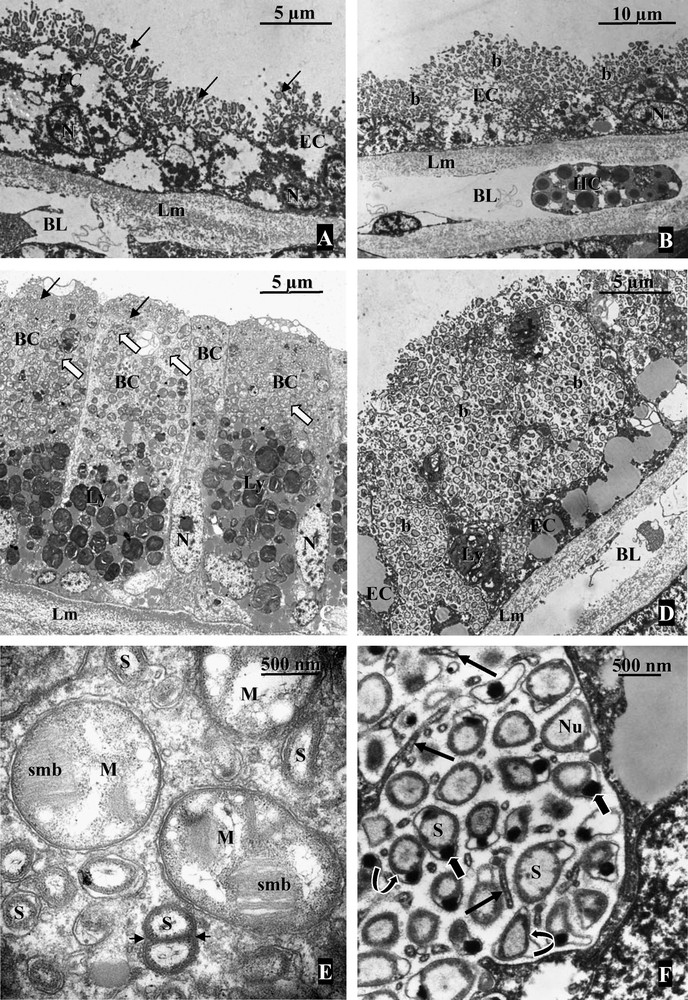
Localization of bacteria in the gill tissue of mussels (TEM). A: Gill filament from MOTU 5. Epithelial cells (EC) harbor numerous extracellular bacteria (arrows) located between microvilli developed by the host cell. BL: blood lacuna; Lm: basal lamina; N: nucleus. B: Gill filament from Adipicola longissima. Extracellular bacteria (b) are located on the apical surface of the host cells (EC) in contact with microvilli. The bacterial layer appears thicker compared to MOTU 5. BL: blood lacuna; HC: hemocyte; Lm: basal lamina; N: nucleus of the host cell. C: Bathymodiolus cf. boomerang. Transverse section through a gill filament showing few bacteriocytes (BC) containing sulfur oxidizing bacteria (small arrows) occupying the apical pole of each host cell while methanotrophic symbionts (large arrows) are more abundant in the basal region of the bacteriocyte. Lm: basal lamina; Ly: lysosome-like bodies; N: nucleus. D: In the mytilid OGS45 from Vanuatu islands, the extracellular bacterial layer is thick with invaginations between very long microvilli developed by the thin epithelial cells (EC). Lm: basal lamina; N: nucleus. E: High magnification of the two symbionts from Bathymodiolus cf. boomerang. The large morphotype (M) corresponding to the methanotrophic symbionts is characterized by the presence of stacked internal membranes (smb). Sulphur-oxidizing symbionts (S) are smaller and a dividing stage can be observed on the section (arrow heads). F: Sulphur-oxidizing bacteria in OGS45, extracellularly located between the microvilli (straight arrows) of the host cells, possess a double membrane (curved arrows) typical of Gram negative bacteria. The DNA (Nu) occupies most of the volume of the bacterial cytoplasm. The periplasmic space does not seem to contain vesicles (such as sulphur granules) usually observed in thioautotrophic bacteria but possesses poly-hydroxybutyrate electron dense granules (large arrows). Masquer
Localization of bacteria in the gill tissue of mussels (TEM). A: Gill filament from MOTU 5. Epithelial cells (EC) harbor numerous extracellular bacteria (arrows) located between microvilli developed by the host cell. BL: blood lacuna; Lm: basal lamina; N: nucleus. ... Lire la suite
Evidence from enzymatic tests, immunology and gene sequencing indicate that symbionts harbour and express enzymes involved in the metabolism of sulphur such as adenosine phosphosulfate (APS) reductase and ATP sulfurylase, and in the Calvin Benson cycle such as ribulose 1,5 bisphosphate carboxylase oxygenase (RubisCO) [27,33–37]. Incubation experiments indicating that sulphide or thiosulfate stimulate CO2 uptake under aerobic conditions, for example, in the thiosulfate specialist B. thermophilus, confirmed that symbionts use reduced sulphur compounds as electron donors to produce the energy necessary to fix dissolved inorganic carbon, although data from more species would be needed [38,39]. While sulphur-oxidizing symbionts associated with many metazoans such as siboglinid tubeworms and lucinid clams deposit inorganic sulfur in granules, symbionts of mussels usually do not [25,33]. Debris of bacterial origin have been identified within phagolysosome-like structures located at the basis of bacteriocytes in mussel species with endosymbiotic thiotrophs, confirming the nutritional role of bacteria [40]. The nutritional role of thiotrophs is less clear in the case of mussels with extracellular bacteria, as degraded bacteria were not convincingly identified within phagolysosomes in these species [29]. Because sulphide poisons cytochrome oxidase in metazoans, another possible role for thiotrophs would be to detoxify reduced sulphur compounds, an adaptation to variable environments with temporary bursts of sulphide-rich fluids [26,41,42].
3.2 Methane-oxidizing symbiosis
Shortly after the discovery of thiotrophic symbionts, methane-oxidizing endosymbionts were shown to occur in the gill epithelium of several mussel species from the Gulf of Mexico, in vacuoles within bacteriocytes [10,43,44]. To date, mussel-associated methanotrophs have only been identified in cold seep and hydrothermal vent mussel species (Table 1). Cavanaugh and co-workers demonstrated the presence of bacteria-like inclusions, 1.5 μm diameter, displaying stacked internal membranes typical of Type I methanotrophs in B. heckerae. Based on their morphology and highly negative stable carbon isotope signatures displayed by gill tissue, close to that of biogenic methane, symbionts were suggested to be methanotrophs (typical morphology of methanotrophs can be seen in Fig. 3C and E). Gills of “B.” childressi were shown to consume methane, and mussel growth was observed with methane as both a carbon and energy source [43,44]. Methane was confirmed to be the dominant source of carbon in several cold seep mussels [10,45]. Experiments using radiolabelled methane indicated rapid transfer of carbon from the symbionts to the host tissue, and lipids typical of methanotrophs were found in the foot of “B.” childressi, a tissue that contrary to the gill does not contain symbionts [46–48]. Methanol dehydrogenase activity was identified early on, but this enzyme is also found in non methane-consuming methylotrophs [10,46]. Presence of methane monooxygenase, the specific enzyme responsible for the first step of methane oxidation, was confirmed using immunology and sequence analysis [45,49]. Although methane can be used as both an electron donor and a carbon source by symbionts, recent evidence show that one methanotrophic symbiont also possesses RuBisCO, which could indicate the presence of a functional Calvin cycle [50]. Besides carbon, methanotrophs have been suggested to participate in nitrogen uptake in “B.” childressi [51,52].
3.3 Multiple symbioses
The first multiple symbiosis discovered in a chemosynthesis-based ecosystem was ‘dual symbiosis’ in which both sulphur- and methane-oxidizing symbionts co-occurred within the same host cells. Dual symbiosis is reported in at least 5 to 6 mussel species: B. azoricus and B. puteoserpentis (Mid Atlantic Ridge (MAR) vents), B. heckerae, B. brooksi and B. boomerang (Gulf of Mexico (GoM) seeps), and B. cf. boomerang (Gulf of Guinea (GoG) seeps) (see Fig. 1 and Table 1) [10,26,27,45,53,54]. Because sulphur- and methane-oxidizing symbionts have distinct requirements with regards to electron donors and carbon sources, their co-occurrence is thought to increase the flexibility of the association, which could improve the fitness of their host in varying environments such as seeps with possible bursts of sulphide or vents with variable fluid regimes. Both sulphur- and methane-oxidizers contribute significantly to the carbon nutrition of their host, as shown in a study where respective contributions of 20% and 15–25%, respectively, were estimated based on the analysis of lipid
In recent years, the screening of large 16S rRNA clone libraries and the use of fluorescence in situ hybridization techniques led to the discovery of 4 and 6 co-occurring symbionts in the gills of B. heckerae and Idas sp., respectively [11,45]. Both species harbour 3 symbionts which belong to the two groups discussed above: a methanotroph and two related, but distinct sulphur-oxidizers (labelled S1 and S2 in Table 1). Both also harbour a bacterium related to free-living methylotrophs of the genus Methylophaga, i.e. bacteria able to oxidize many C1 compound except methane. Despite the lack of direct evidence, it has been suggested that these symbionts could consume methanol, a compound present in fluids, but also a by-product of methanotrophs activity. Idas sp. shelters two additional symbionts, a gammaproteobacterium with no cultivated close relatives and a member of the Bacteroidetes (CFB) related to pathogens and cellulose-oxidizing bacteria. While their role is unknown, a stimulating hypothesis was that the later would be a symbiont able to degrade sunken wooden substrates because close relatives of Idas sp. occur in such environments. However, no CFBs were identified in 16S rRNA clone libraries obtained from wood-associated mussels [29].
3.4 Dynamics of symbiont populations
Bathymodiolines from hydrothermal vents experience local environments where hydrogen sulphur concentrations are variable and sometimes high, reaching for example 325 μM at the Rose Garden vent field, and more typically comprised between 1 and 40 μM [56]. Seep species such as B. cf. boomerang live in environments displaying high methane concentrations, reaching, for example, up to 33.7 μM around mussels at the Régab site in the Gulf of Guinea [26]. Although accurate and relevant measurements are hard to perform in situ at the deep-sea, it is clear that reduced compounds are abundant around mussels, although spatial and temporal variability is high [57,58]. Some data confirm that these compounds are necessary for the survival of mussels and their symbionts. B. thermophilus specimens from the waning vent site Animal Farm (South East Pacific Rise) displayed thinner bacteriocytes with fewer bacteria and lower condition indexes than specimens from an active site, indicating mussels in bad condition; in the same study, thiotrophic symbionts disappeared quickly after transfer of specimens from an active site to an inactive zone of barren basalt [59]. Starvation experiments on B. azoricus specimens kept in aquaria confirm that the loss of symbionts is rapid, but also that it is reversible [60]. Symbionts are thus quickly lost when reduced compounds are not available anymore, and mussels having lost their symbionts are not healthy. These results indicate that symbiosis is obligatory for the mussels. This is congruent with the observation that early post-larval stages of B. azoricus already display their typical two symbionts [61].
In the case of dual symbiotic mussels, several studies have pointed the link between environmental variables and abundances and contributions of symbionts. B. azoricus and B. puteoserpentis from the Mid Atlantic Ridge were shown to harbour a smaller proportion of methanotrophs versus thiotrophs at sites where end-member fluids contained less methane [27,36,62]. Total abundances of symbionts were shown to be variable between specimens of the dual symbiotic species B. brooksi from Atwater Canyon and Alaminos Canyon, two cold seep sites in the Gulf of Mexico, although methanotrophs were dominant over thiotrophs at both sites [45]. Recently, short pulses of sulphur were shown to increase the density and relative abundance of sulphur-oxidizers in bacteriocytes of B. azoricus using quantification techniques based on fluorescence in situ hybridization (FISH) [63]. Such quantifications of symbiont abundances will have to be coupled with detailed investigations of the chemical characteristics in the immediate environment of mussels to really quantify the effect of in situ environmental parameters, but it is becoming clear that the dynamics of symbiont populations are influenced by the environment.
4 Phylogenetic relationships of bacterial symbionts based on 16S rRNA sequences and evolution of symbiosis in bathymodiolines
All sulphur-oxidizing symbionts of mussels belong to a single large, well-defined clade within the Gammaproteobacteria. This clade also includes several sequences from free-living bacteria and several from other bivalve symbionts, namely thyasirid-associated ectosymbionts and vesicomyid-associated endosymbionts, as shown in trees from the following references [7,26,64,65]. Reconstructed symbiont phylogenies are usually poorly resolved and display very few supported nodes [45]. The poor resolution is probably a consequence of the low variability of 16S rRNA in this group (only up to 4.9% divergence between sequences within the group), and the lack of a good (i.e. close) outgroup clearly hampers the quality and reliability of the reconstruction. Altogether, this indicates that 16S rRNA is not an accurate marker to investigate symbiont phylogeny, and that markers containing more phylogenetic information, such as 23S rRNA or Internal Transcribed Spacers, would be more appropriate as shown by two studies [66,67].
Because the two paraphyletic, deep-branching, mussels Bathymodiolus sp. (Juan de Fuca) and MOTU 16, as well as all bathymodiolines but three (“B.” japonicus, “B.” platifrons, and “B.” childressi, representing a single clade) display sulphur-oxidizing symbionts (see Table 1 and Fig. 1), it can be suggested that thiotrophic symbiosis was present in the latest common ancestor of bathymodiolines. It could have represented one of the key adaptations favouring the diversification of the group, also explaining why today's bathymodiolines are restricted to reduced environments.
The occurrence of extracellular bacteria in the gills of sunken wood mussels was considered significant because ectosymbiosis probably represents the earliest type of association on the evolutionary route to endosymbiosis, supporting the hypothesis of a progressive process of symbiont internalization leading to true endosymbiosis found in vent and seep mussel species. Host phylogeny presented in Fig. 1, however, shows that there is no evident shift from ecto- to endosymbiosis. This is either not the case in published symbiont phylogenies [29]. Extracellular bacteria are related but not paraphyletic to endosymbionts, indicating that localization is not a driving force of the evolution of symbionts. The ambiguous localization of symbionts in B. sp. JdF is problematic, and detailed studies about symbiont localization in deep-branching mussel species such as Benthomodiolus lignicola would be needed to figure out whether ‘ectosymbiosis’ could be ancestral in bathymodiolines. At present, it appears that certain species, mostly small mussels which do not form a coherent group, display extracellular sulphur-oxidizing bacteria. Whether bacterial localization depends upon host species or a habitat could be tested if mussel species colonizing several types of habitats (vents, seeps, organic falls) were discovered.
The topology of the thiotrophic symbiont tree is significantly distinct from that of the host tree (AU test using CONSEL,
Mussel-associated methanotrophs belong to a gammaproteobacterial clade which, to date, only includes mussel symbionts, suggesting a single common origin of methanotrophic symbionts which would imply that the ability to establish symbiosis appeared once in this group of bacteria [70]. Their closest relatives are free-living Type I methanotrophs, as could be expected from symbiont morphology displaying typical internal stacked membranes (see Fig. 3E) [71]. All symbiont 16S rRNA sequences are closely related, displaying a maximum divergence of 3.2%. As for thiotrophs, B. azoricus and B. puteoserpentis share a single 16S rRNA methanotroph phylotype, and methanotrophs associated with B. cf. boomerang and B. heckerae are closest relatives [36,45]. Apart from these two clades, topologies of host and methanotroph trees do not display common well-supported nodes (AU test:
Few elements are available that would explain this lack of co-speciation, because the question of how methanotrophs are acquired is not resolved. For example, a puzzling observation is that B. brooksi and “B.” childressi co-occur within the same mussel beds at Alaminos Canyon (GoM) but display clearly distinct methanotroph phylotypes [45]. Overall, symbioses involving methanotrophs are present in less than half of the mussel species, and are spread over the host tree. From the point of view of the host, these symbioses could reflect opportunistic associations rather than real evolutionary trends. Indeed, methanotrophic symbioses are found in mussel species exposed to moderate-to-high methane concentrations, in particular at cold seeps. In this regard, the absence of methanotrophic symbioses in environments such as organic falls could be due to the absence of significant methane sources in these areas. The possibility to establish opportunistic associations could also explain the presence of additional symbionts besides sulphur- and methane-oxidizers [11,45]. It may well be that a diversity of bacteria which are able to establish associations with metazoans occur in the environment.
5 Conclusion and future directions
More information is becoming available regarding the diversity, role and functioning of mussel symbiosis. Symbiosis seems to be obligatory for the host, but thiotrophic symbionts, at least, are environmentally acquired and both sulphur- and methane-oxidizers do not co-speciate with their hosts. Our understanding of the evolution of mussels and their symbionts is however still fragmentary. It is limited by the need for expensive cruises, and biased towards focused research areas such as the mid Atlantic, the eastern and western Pacific, and the Gulf of Mexico. Mussel as well as symbiont phylogenies are not well resolved and more marker genes and species are needed. Similarly, the true diversity of bacterial symbionts is probably underestimated.
How important and integrated is the interaction for the host and its associated bacteria, and how it is influenced by the environment remain to be determined. Although suspected due to indications of environmental acquisition of symbionts, the existence of free-living forms of symbionts is not yet proven, and how hosts and symbionts recognize each other and establish the interaction is not elucidated. Finally, how symbiosis shapes the evolution of mussel hosts and their associated bacteria is not known. For example, symbiosis was recently shown to have led to genome reduction in the maternally inherited thiotrophic symbiont of Calyptogena magnifica [72]. Genomics are thus the next big step forward needed to better understand mussel symbioses.
Acknowledgements
The material from the Philippines was obtained during the PANGLAO 2005 cruise aboard M/V DA-BFAR while mussel OGS45 from the Vanuatu was obtained during the SantoBOA 2006 cruise (chief scientists: B. Richer de Forges, S. Samadi) and we thank Dr P. Bouchet, L. Labe, and H. Le Guyader the co-principal investigators, for their invitation. The technical part was supported by the ANR “deep oases”. TEM images were obtained at the “Service de Microscopie Électronique de l'IFR 83-Biologie Intégrative CNRS (Paris, France)”. J. Lorion is funded by a grant from the graduate school Diversité du Vivant (UPMC).