1 Introduction
As an element greatly required for plant physiological and molecular activities, Ca2+ plays important roles in regulating plant metabolism, growth and development. Moreover, Ca2+ also participates in many physiological and biochemical reactions in plants as a common second messenger through coupling both cellular and intercellular signal transduction networks. Growing evidence shows that cytosolic Ca2+ concentrations can be induced to change by various environmental conditions and hormone signal molecules, such as wind, illumination, low temperatures, salt, alkali, gibberellins, ABA (abscisic acid). These changes of cytosolic Ca2+ concentrations occur before the physiological activities of the plants [1–15]. Therefore, it is speculated that the change of Ca2+ concentration and distribution in plant cells induced by the plant itself may be considered as the plant's responses and adaptations to the ever-changing outside environment. The distribution and translocation of cytosolic free Ca2+ serve as fundamental basis for the formation of Ca2+ signals. At present, the distribution of cytosolic Ca2+ and the change of plant cell's super-microstructures under cold stresses are investigated in depth while the distribution of cytosolic Ca2+ and its sub-cellular localization under drought stresses are less studied [16–28].
It has been demonstrated by more research that the addition of calcium to leaves could enhance water conservation capability of both plant leaves and their cellular membranes, thus effectively ameliorating plant water conditions under drought stress [29–41]. It is also considered that Ca2+ can alter the hydration degrees of membrane, and improve the cohesion of cell walls, thus enhancing the viscosity of protoplasm and cells' capacity of dehydration resistance. In addition, Ca2+ can improve the hydrophobicity of cellular membrane while lowering its permeability through connecting to the phosphates and carboxyl of phosphatides and proteins in cellular membranes, thus strengthening the stability of membranes, which shows that Ca2+ can stabilize plant cells through its direct effects as a structural basis for plant drought-resistance [42–59]. The report concerning the change of cellular super-microstructures merely focused on nucleus and chloroplast. In this paper, the change of cytosolic Ca2+ concentrations and cellular super-microstructures of maize at different drought stress stages were researched in detail, providing theoretical basis for the investigation of the Ca2+ roles in plant drought-resistance. In plant cells, it was already reported that ABA-induced cytosolic Ca2+ concentration increase participated in stomatal closure [16–24], while the temporal and spatial specificities of ABA-induced changes of cytosolic Ca2+ distribution and concentration are rarely reported. Drought is a major stress to agricultural production. Plants synthesize ABA in response to drought, triggering a signaling cascade in guard cells that results in stomatal closure, thus reducing water loss that may influence water use efficiency in plants. In addition, drought is also related to salt stress, cold stress, high temperature stress, acid stress, alkaline stress, pathological reactions, senescence, growth, development, cell circle, UV-B damage, wounding, embryogenesis, flowering, signal transduction and so on [25–47,60–73]. In this report, the changes of cytosolic Ca2+ distribution and concentration are traced and measured through the application of transmission electron microscope (TEM) technique under drought stress, attempting to further discover the role of Ca2+/CaM in the drought signal transduction and the cause of ABA-induced cytosolic Ca2+ concentration increase.
2 Materials and methods
The tested material in this experiment is the hybridized maize (Zea mays L.) cultivar called “zhengdan958”. According to [48–51], the fully-nourished seeds were selected, sterilized by 0.1% HgCl2 for 10 min and repeatedly washed by water. 24 h after seed soaking, the seeds were cultured in an incubator for germination with a constant temperature of 28 °C. When the bud length reached 1 cm, the seeds were planted into vermiculite pots. When the first true leaf was entirely extended, the seedlings were sampled. The sample leaves were quickly soaked into PEG-6000 (−0.85 MPa) for stress treatments of 30, 45, 60, and 75 min, respectively. After these treatments, cytochemical localization was implemented in the cells of sample leaves. The sample leaves were then collected for the following treatments: (1) treated by the direct addition of 50 μM ABA; (2) soaked into 2 mM EGTA solution (supplemented with 10 mM MES, 50 mM KC1, pH 6.1) for 30 min for illumination avoidance and then treated by 50 μM ABA; (3) soaked into 100 μM Verapamil solution (supplemented with 10 mM MES, 50 mM KC1, pH 6.1) for 30 min for illumination avoidance and then treated by 50 μM ABA; (4) soaked into 80 μM TFP solution (supplemented with 10 mM MES, 50 mM KC1, pH 6.1) for 30 min for illumination avoidance and then treated by 50 μM ABA. The cytochemical localization of Ca2+ was implemented as following: 2% potassium pyroantinonate solution (represented by “A solution” in the following context, pH 7.6) was formulated with potassium phosphate. A solution should be formulated as a fixed solution containing 3% glutaraldehyde. The sample leaves were treated by such reagent with a size of 1 mm × 0.3 mm × 10 mm and fixed overnight. After overnight, theses leaves were washed by A solution for 3 times with an interval of 0.5 h for each time. Then, theses samples were fixed again by osmic acid formulated with A solution for 2 h, washed 3 times by double distilled water with an interval of 0.5 h for each time, and then washed twice by double distilled water (pH 10) with an interval of 0.5 h for each time. Next, the samples were placed into ethanol with various concentration gradients, and dehydrated, embedded, ultramicrocut, and dyed with uranium acetate, respectively. After the above operations, the approximate configuration and dense electronic particles of these leaves can be observed under transmission electron microscope (TEM), thus further inferring the location of Ca2+. The Ca2+ cellular localization was determined as follows: the photographed slices were placed into EGTA (a kind of Ca2+ chelators) for their chelating with Ca2+, the above slices were photographed when the dense electronic particles disappeared, and then the Ca2+ cellular distribution was determined.
3 Results
It can be concluded from the results of TEM observation that calcium pyroantinonate precipitate particles created from cellular Ca2+ localization appeared in large quantity in vacuoles, and occurred occasionally in chloroplasts, nucleus and Golgi apparatus. It was reported that Ca2+ distributed in endoplasmic reticulum (ER) which also represents a commonly-considered cellular Ca2+ sink with large quantity, while such large amounts of Ca2+ distribution was not found in ER in this experiment. Plant cell wall represents another kind of cellular Ca2+ sink, and in this experiment, Ca2+ existence was found in cell walls. In addition, large amounts of calcium pyroantinonate precipitate particles were also observed in the intercellular gaps outside the cells (CK).
In Fig. 1.1, it can be observed that 30 min after PEG stress, the free Ca2+ concentration in cytoplasm and nucleus considerably increased. The large amounts of Ca2+ precipitate particles formed in the internal sides of the plasma membrane represented remarkable marks for such cellular Ca2+ concentration elevation. These particles were relatively orderly arranged and almost formed a particle circle in the internal sides of the plasma membrane. In vacuoles, Ca2+ was localized in the outside of vacuolar membrane. After PEG stress, vacuolar Ca2+ concentration significantly decreased (Fig. 1.1a), and large amounts of Ca2+ distribution occurred in the outside of chloroplast capsules (Fig. 1.1a). Contrary to the apparent change in cellular Ca2+ concentration, no remarkable change in cellular super-microstructure was observed (Fig. 1.1b). As shown in Fig. 1.2a, 45 min after PEG stress, the cells transformed, but such cell transformation was not significant. Relatively larger Ca2+ precipitates can be observed in the outside of cell walls, and relatively huge amounts of Ca2+ precipitate particles can also be found in cytoplasm. Compared with those under 30 min PEG stress treatment, the precipitates under this treatment were disorderly and unevenly distributed, and considerably concentrated in certain parts of plant cells. Some chloroplasts expanded and became round with their enlarged internal stromal spaces and expanded granular thylakoid, while the morphological change occurred in some other chloroplasts (Fig. 1.2a). With the continuous increase of Ca2+ concentration in the nucleuses and the appearance of larger black particles, the heterochromatin in the cells conglomerated (Fig. 1.2b). With cell transformation, the cytosolic Ca2+ concentration enhanced significantly, while the Ca2+ particles had irregularity in their configurations, some of them were big and dense black particles, while some others of them presented irregular hollow formations (Fig. 1.3a). In chloroplasts, Ca2+ concentration improved dramatically, and with the further expansion of granular thylakoid membranes, the distorted parts of membranes and the lamellar structures become vague while observed, and the increase of osmic acidophilic particle numbers took place in some chloroplasts (Fig. 1.3b). Some chloroplasts conglomerated with each other, forming a “butterfly configuration” (Fig. 1.3c). With relatively large amounts of Ca2+ particles mainly concentrated in the centre of nucleus, chromatins distributed in all directions around the nucleus (Fig. 1.3d). Observing Fig. 1.4a, it can be seen that cytosolic Ca2+ assembled in large quantities in certain cellular areas, and the occurrence of membrane breakages was found in some Ca2+ precipitate-centralized cellular areas. The serious chloroplast transformation was presented, and the black block masses with high electron density appeared after the partial breakage of some chloroplasts and the exosmosis of their stroma (Fig. 1.4b). With its collapsed and sectional vague membranes, the nucleus suffered from its serious transformation (Fig. 1.4c).
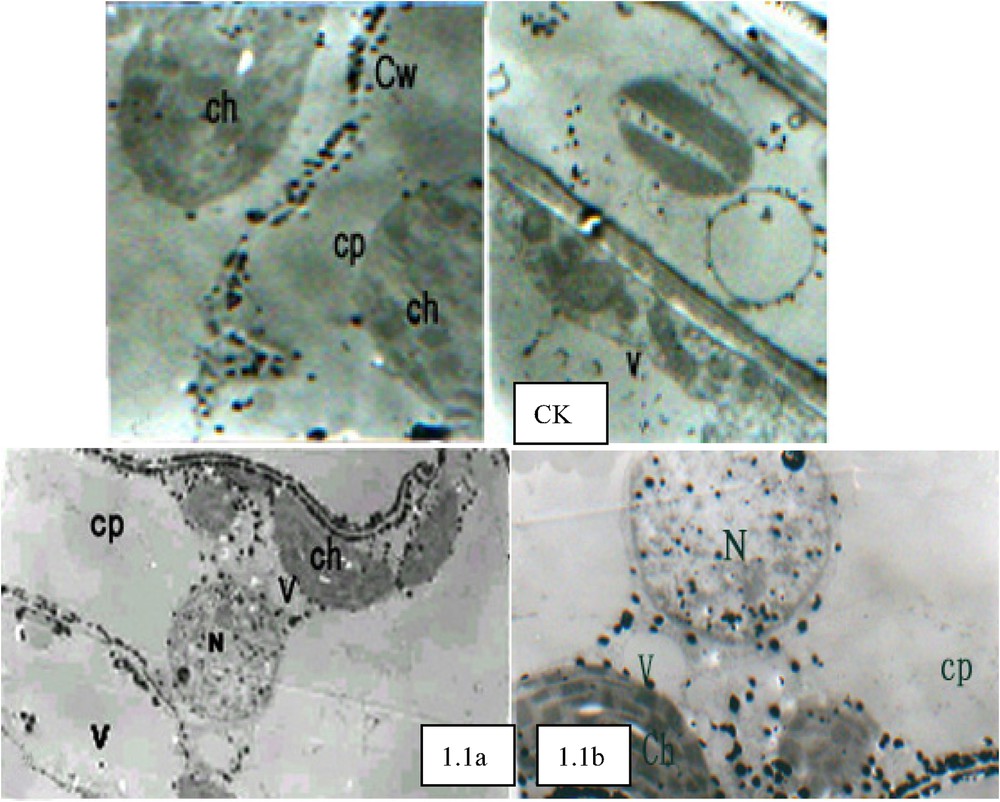



The cellular Ca2+ distribution under PEG stress. CK (×5000), large amounts of calcium pyroantinonate precipitate particles can be observed in the intercellular gaps outside the cells. 1.1. PEG stress for 30 min (×6000): (a) Vacuolar Ca2+ concentration significantly decreased compared with CK. (b) Cellular super-microstructure remained unremarkable change compared with CK. 1.2. PEG stress for 45 min (×5000): (a) The cells were not significantly transformed. Relatively larger Ca2+ precipitates can be observed in the outsides of cell walls, and relatively huge amounts of Ca2+ precipitate particles can also be found in cytoplasm. Compared with 30 min PEG stress treatment (1.1), the precipitates under this treatment were disorderly and unevenly distributed, and considerably concentrated in certain parts of plant cells. (b) With the continuous elevation of Ca2+ concentration in the nucleuses and the occurrence of relatively large black particles, heterochromatin conglomerated. 1.3. PEG stress for 60 min (×6000): (a) With cell transformation, cytosolic Ca2+ concentration enhanced significantly, while the Ca2+ particles had irregularity in their configurations. (b) In chloroplasts, Ca2+ concentration improved dramatically. (c) Some chloroplasts conglomerated with each other, forming a “butterfly configuration”. (d) With relatively large amounts of Ca2+ particles mainly concentrated in the centre of nucleus, chromatins distributed in all directions around the nucleus. 1.4. PEG stress for 75 min (×8000): (a) Cytosolic Ca2+ assembled in large quantities in certain cellular areas, and the occurrence of membrane breakages was found in some Ca2+ precipitate-centralized cellular areas. (b) Chloroplast transformed seriously, and the black block masses with high electron density appeared after the partial breakage of some chloroplasts and the exosmosis of their stroma. (c) Nucleus transformed seriously with its collapse membranes. Ch: chloroplast; Cp: cytoplasm; Cw: cell wall; N: nucleus; V: vacuole.
According to Fig. 2.1, it can be known that cellular after the ABA treatment to seedling leaves, cellular Ca2+ distribution changed significantly. The concentrations of Ca2+ precipitates decreased both in vacuoles and outside cells, while huge amounts of Ca2+ precipitate particles can be observed in the internal sides of membranes with the dramatic enhancement of cytosolic Ca2+ concentration. Compared with ABA treatment, EGTA and ABA jointed treatment had quite different effects on the changes of cellular Ca2+ distribution in seedling leaves. From Fig. 2.3, it can be observed that cellular Ca2+ concentration increased slightly, while Ca2+ outside cells can not be seen because of the elimination of it caused by EGTA chelating (Fig. 2.2). After Verapamil (an inhibitor to Ca2+ channels) and ABA jointed treatment, the cellular Ca2+ accession from outside into inside was inhibited, because Verapamil interrupted the Ca2+ channels in the membranes, thus leading to the obviously-observed large quantities of Ca2+ distribution in both intercellular gaps and cell walls. While the cytosolic Ca2+ concentration did not increase significantly (Fig. 2.3). TFP (an inhibitor to CAM) affected the cellular Ca2+ accession from outside into inside to some extent. Many Ca2+ precipitates can be seen in intercellular gaps, while the trend of cytosolic Ca2+ concentration elevation was not significant than such trend observed under ABA treatment (Fig. 2.4). After EGTA treatment, the precipitates in the slice were eliminated. The original sectors for Ca2+ distribution such as intercellular gaps, chloroplasts, vacuoles, and plasma membranes presented transparent electron areas similar as the original precipitate structures, demonstrating a real reflection of Ca2+ localization in the slice (Fig. 2.5).


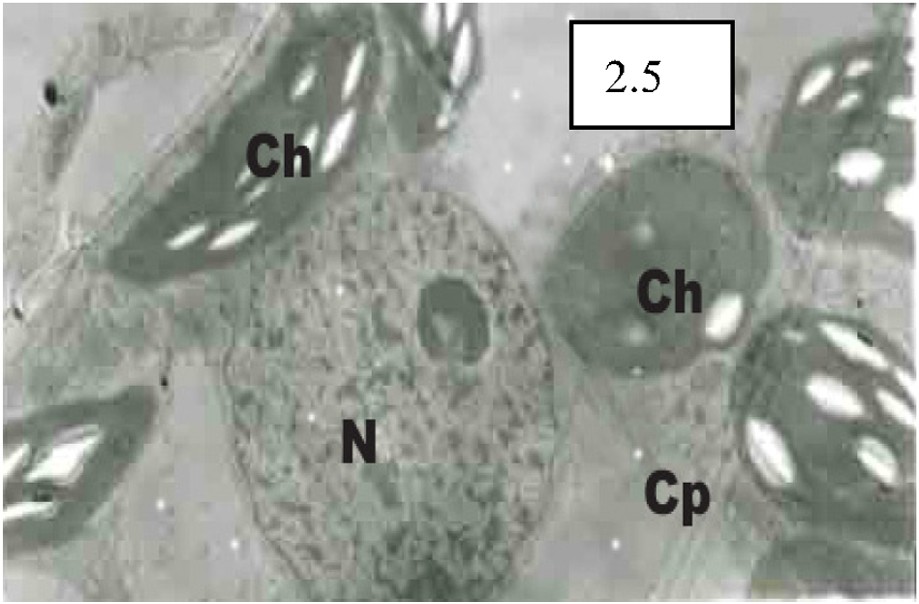
The cellular Ca2+ distribution under ABA treatment, different jointed treatments and EGTA treatment. CK (×5000), large amounts of calcium pyroantinonate precipitate particles can be observed in the intercellular gaps outside the cells. 2.1. ABA treatment (×6000): Cellular Ca2+ distribution changed significantly compared with CK. 2.2. EGTA and ABA jointed treatment (×6000): Ca2+ outside cells can not be seen because of the elimination of it caused by EGTA chelating. 2.3. Verapamil and ABA jointed treatment (×6000): Large quantities of Ca2+ distribution in both intercellular gaps and cell walls can be obviously observed although cytosolic Ca2+ concentration did not increase significantly. 2.4. TPF and ABA jointed treatment (×8000): TFP affected the cellular Ca2+ accession from outside into inside to some extent, and many Ca2+ precipitates can be seen in intercellular gaps, while the trend of cytosolic Ca2+ concentration elevation was not significant than such trend observed under ABA treatment (2.1). 2.5. EGTA treatment (×10 000): The precipitates in the slice were eliminated. The original sectors for Ca2+ distribution such as intercellular gaps, chloroplasts, vacuoles, and plasma membranes presented transparent electron areas similar as the original precipitate structures. Ch: chloroplast; Cp: cytoplasm; Cw: cell wall; N: nucleus; V: vacuole.
4 Discussion
The relationship between the nucleus of wheat seedling and Ca2+ under drought stress was examined with the cytochemical method of improved calcium antimonate precipitation. The results showed that there was a small concentration of Ca2+ in nucleus, the shape of the nucleus liked a ball and the chromatin was scattered distributed. The longer the duration of drought stress the higher the free Ca2+ concentration in nucleus and the more serious the ultrastructure of the nucleus, meanwhile, the membrane of the nucleus wrinkled and the chromatin seriously agglutinated, the shape of the nucleus was abnormal till its final disintegration [51–53]. It can be directly observed that under drought stress, the Ca2+ distributions and the cellular microstructures all changed in seedling leaves. At the early stage of drought stress, the nucleus and the chloroplast played important roles in regulating cellular Ca2+ concentrations, and the sectional distributions of Ca2+ in plant cells also provided vital roles in regulating the physiological activities regarding plant drought-resistance. Ca2+ participated in the processes of drought-stress and ABA-induced drought signal transduction as a pivotal medium. ABA could induce cytosolic Ca2+ concentration elevations in the mesophyll cells and guard cells of maize seedling leaves, such elevations originated mainly from extracellular Ca2+ sinks and partially from cellular Ca2+ sinks such as vacuoles. Extracellular CaM could participate in the process of drought signal transduction as an important medium through inducing the changes of cellular Ca2+ concentrations. It is also indicated and demonstrated in this experiment that under normal conditions, cellular free Ca2+ concentrations actually remain very low, and vacuole serves as a main Ca2+ sink for higher plants. Identical to the results of chemical measurement, this experiment also proved that there were relatively large quantities of Ca2+ distributions in cell walls and among intercellular gaps.
It has been proved by a growing number of researches that the sectional distribution of Ca2+ in cells can be changed through altering the transportation of Ca2+ carriers in membranes and the status of cellular Ca2+ storage bodies [5–9,21–28,45–49,53–56], which can be stimulated by many exogenous and endogenous elements such as light, low and high temperatures, gravity, oxygen shortage, salt stress, hormone and gene-regulated physiological changes, thus further inducing a series of physiological and biochemical reactions in cells, and finally manifesting a change for the status of plant development and metabolism [22]. Therefore, the knowledge of the principles for plant signal transduction can be enriched and proved directly through determining the changes of cellular Ca2+ concentrations and understanding the spatial-temporal rules for such changes after cells' stimulus reception. Meanwhile, such researches can also provide some certain theoretical instructions to regulate plant development and metabolism.
In this experiment, it can be seen that membrane Ca2+ orderly distributed, and Ca2+ concentrations decreased dramatically in vacuoles and intercellular gaps 30 min after PEG stress. Therefore, it can be observed that the cytosolic Ca2+ partially originated from cells' outside and partially came from vacuoles (cellular Ca2+ sinks). With PEG stress continued, the cytosolic Ca2+ concentrations also elevated increasingly, and the cells transformed. 75 min after PEG stress, membrane breakage occurred in the sections where Ca2+ concentrated in large quantities. Numerous reports indicated that at the early stage of cold-stress, Ca2+ concentrations increased significantly in cytoplasm, but the concentrations of Ca2+ precipitates decreased in vacuoles and intercellular gaps. Therefore, it was speculated that under the stimulus of cold stress, Ca2+ in vacuoles and intercellular gaps can access into cytoplasm through Ca2+ transport channels in the membranes and other pathways as a second messenger, initiating and controlling relevant gene transcriptions and regulations aiming at cold stress resistance, thus leading to a series of physiological and biochemical reactions such as the occurrence of cold-tolerance relevant proteins, the enhancement of some enzymes' (including catalase, ATPase and so on) activities, and finally improving plant capacities of cold-resistance [50,65–73]. It can be speculated in this experiment that Ca2+ presents similar mechanism in plant drought and cold resistances, because the changes of Ca2+ distribution were identical both at the early stage of drought stress and under cold stress. It was also reported that Ca2+ applies such mechanism to enhance plant drought resistance as following: on one hand, Ca2+ can strengthen the stability of plant cellular structure through its direct effects; on the other hand, Ca2+ can also affect cellular protective systems, damage their functions and maintain the metabolic balance of such bio-radicals as active oxygen through its indirect effects [52]. Such report was also supported by the changes of Ca2+ distribution concluded in this experiment. The cytosolic Ca2+ concentrations and distributions can play active roles in regulating outside environments if they change in optimal extents. While if such changes overpass certain extents, they'll bring destructions and disturbs (such as the changes of cytoskeleton and membrane structure) to normal cellular structures and functions, altering membrane permeability and finally leading to the imbalance of cellular metabolism and the occurrence of disorders [39]. It was also found that the direct addition of Ca2+ carriers (such as A23187) or other elements to cell plant lines can lead to the elevation of cellular Ca2+ concentrations or the destruction of Ca2+ stable imbalance both inside and outside cells, thus further inducing cell death, demonstrating that there exist certain relationships between Ca2+ and cell death. In this experiment, with the advancement of drought stress, cytosolic Ca2+ concentrations continuously enhanced, and cells continuously transformed and finally disintegrated, which indicated that at the early stage of drought stress, the drought-resistance of cells enhanced with the elevation of cytosolic Ca2+ concentrations, while the increasing enhancement of Ca2+ concentrations may serve as an induction element for cell death during drought stress [67–73].
It was found in wheat seedling that under normal conditions, the chloroplast presented elliptical shapes with orderly-laminated granules and small amounts of Ca2+ distributions. With the advancement of drought stress, Ca2+ concentrations in chloroplasts increased, and chloroplasts transformed and finally disintegrated [52]. In another report, Zhong [51] also found the changes of chloroplast super-microstructures while observing the cellular super-microstructures in drought-stressed sugar-cane leave cells, which further clarified Wang's results. As important places for photosynthesis, chloroplasts can unavoidably produce such active oxygen as superoxide radicals during the process of photosynthesis. Under normal physiological conditions, the production and elimination of these radicals are balanced, bringing no damage to plants. While under drought stress, such balance can be destroyed, thus leading to the peroxidation of membrane lipids, causing membrane leakage and the destruction of cellular super-microstructures. In this experiment, the Ca2+ concentrations in chloroplasts increased because of chloroplasts' effects on cytosolic Ca2+ concentration regulation as Ca2+ pumps, and the increased Ca2+ concentrations in chloroplasts are also beneficial for the stabilization of their membranes. However, with the advancement of drought stress, chloroplasts' structures were destroyed, especially that the transformation of their granular laminas may make great contributions to cell death. The membrane structures in chloroplast granular laminas play pivotal roles in luminous energy absorption, transmission and conversion. So, the damage of such structures may lead to metabolic disorders and finally result in cell death [27–35,57–62].
At the early stage of drought stress, despite of the elevation of Ca2+ concentrations in nucleus, the structures of nucleus remained stable, which demonstrated the roles nucleus played in cytosolic Ca2+ concentration regulation. However, with the continuous elevation of Ca2+ concentration, chromatins conglomerated and nucleus membranes disintegrated. Through conducting the research of the effects of Ca2+ on the reconstruction of non-cellular nucleus systems, Zhao and Zhang [52] found that the addition of EGTA to Ca2+-contented samples led to the elimination of chromatin conglomeration in striping sperms, while without such EGTA addition, the chromatins were highly-conglomerated in Ca2+-contented striping sperms. Therefore, it can be speculated that high Ca2+ concentrations may play inhibited roles in the elimination of chromatin conglomeration. Such speculation can be expressly proved through observing the TEM slices produced in this experiment. Zhao's experiment results were also proved by Wang's reports (Wang and Zhang [50]) regarding the researches of the relationships between Ca2+ and the changes of nucleus super-microstructures in wheat seedling leaves under drought stress, which is similar to the results observed in this experiment.
It was showed that although various stresses can all lead to the increase of cytosolic Ca2+ concentrations, they have different origins for Ca2+ concentration increase. In the heat-shock reactions of tobacco, the increase of cytosolic Ca2+ concentrations originated from two Ca2+ sinks inside and outside the cells, respectively. While in the process of low temperature-induced alfalfa cold acclimatization, such increase originated from Ca2+ sinks outside the cells, and Ca2+ can be released from cellular Ca2+ sinks even through touch and wind. In this experiment, after the implementation of EGTA, Verapamil and TFP pretreatments, although ABA could induce the elevation of cytosolic Ca2+ concentrations, the extents for such elevation were significantly lower than such extents under only-ABA induction without pretreatments. Therefore, it can be directly concluded that ABA-induced elevation of cytosolic Ca2+ concentrations originated mainly from Ca2+ sinks outside sells and partially from cellular Ca2+ sinks. Ca2+ signals can be created directly from Ca2+ sinks outside cells and released from cellular Ca2+ sinks, or it can also be jointly produced by both types of Ca2+ sinks. Currently, pharmacological experiments are mainly conducted for the investigation of the origin of Ca2+ concentration elevation due to technological limitations. It has been clarified that the changes of cytosolic Ca2+ concentrations mainly resulted from Ca2+ released from cellular Ca2+ sinks in such aspects of experiments as pollen tube growth [24], oxygen shortage [19], cold-shock [30] and so on. While as to the researches regarding plant defensive reactions, almost all the results manifested that Ca2+ outside cells played paramount roles as showed in the experiments applying such plant materials as soybean, carrot, Petroselinum sativum and tobacco. In these experiments, Ca2+ concentrations lowered, leading to the partial inhibition of phytoalexin synthesis [56]. However, the elevation of Ca2+ concentrations induced by cold stimulation, drought stress, oxygen shortage and salt stress mainly originated from the joint effects of extracellular Ca2+ influx and release of Ca2+ from cellular Ca2+ sinks [41–46]. Through TEM, it can also be observed in this experiment that the elevation of cytosolic Ca2+ concentrations could be induced by 50 μM ABA treatments to seedling leaf cells, while if extracellular Ca2+ influx was blocked by other reagents, the elevation of cytosolic Ca2+ concentrations could also be inhibited to some extents, showing that ABA-induced cytosolic Ca2+ concentrations elevation mainly originated from extracellular Ca2+. Contrary to the results of TEM observation, under digital con-focus microscopic observation, ABA-induced Ca2+ concentrations were not affected by any element 30 min after 100 μM Verapamil pretreatments. The following two possibilities could lead to such results: (1) different Ca2+ channels in the membranes have different sensitivities to Verapamil; or (2) ABA-induced Ca2+ concentrations elevation could originated from the release of Ca2+ from cellular Ca2+ sinks, which can be demonstrated by a 2 mM EGTA pretreatment experiment in maize cuticles. The results of such experiment showed that ABA-induced Ca2+ concentrations elevation could be inhibited by EGTA, indicating that such elevation might mainly originate from extracellular Ca2+ influx [1–5,31–36].
As a small protein molecule with a molecular weight of 17 kDa, CaM is universally-existed in plants. In this experiment, ABA-induced cytosolic Ca2+ concentrations elevation was inhibited by TFP, which represents an inhibitor to CaM and its analogues, showing that CaM participated in ABA signal transduction. Then, how could TFP inhibit ABA-induced cytosolic Ca2+ concentrations elevation through antagonizing CaM? As widely existing in plant cells as well as cell walls, cellular CaM serves as the most important Ca2+ receptor protein for initiating downstream Ca2+ signal events. In this experiment, through applying TFP, only the downstream Ca2+ signal events could be affected, while the changes of Ca2+ concentrations could not be blocked. Therefore, the effects of TFP on the pathway of ABA signal transduction could only be implemented through antagonizing CaM in cell walls. It was also proved in the experiment applying broad bean cuticle cells that Ca2+ concentrations could be elevated by extracellular CaM, and therefore CaM was considered to have the functions of signal molecules as extracellular signal elements, which could cause the changes of Ca2+ concentrations [66–73]. In this experiment, it was also showed that ABA could induce and transmit the signals of Ca2+ concentrations elevation through extracellular CaM.The total report here clearly provided a direct evidence for calcium ion as an important signal. However, the related molecular mechanism for ABA's correlation with extracellular CaM in inducing the changes of Ca2+ concentrations remains to be further studied and clarified at the next step.
Acknowledgements
The research in this article is jointly supported by the Natural Science Foundation of Hebei Province (C2007000994), China, National Science & Technology Supporting Project (2007BAD69B01), the Cooperative & Instructive Foundation of State Key Laboratory of Soil Erosion and Dryland Farming on the Loess Plateau (10501-HZ) and the Open Foundation of State Key Laboratory of Soil Erosion and Dryland Farming on the Loess Plateau (10501-194). Thanks are also extended to two reviewers for their concrete suggestions and comments.