1 Introduction
Iron (Fe) is an essential nutrient for plants, but although abundant in the earth crust, it is not readily available for plants. Consequently, Fe deficiency often limits plant growth leading to several agricultural problems. Indeed, one third of the cultivated area in the world is made of calcareous soils and is considered as Fe-deficient lands [1]. Under such tricky environmental conditions, higher plants have evolved specific mechanisms for iron acquisition. Graminaceous (Poaceae) can enhance Fe uptake from sparingly soluble inorganic Fe(III) compounds through a release of natural chelators, phytosiderophores (PS). These species widely differ in their iron deficiency tolerance. In general, plant species releasing higher quantities of PS are more Fe-efficient than those releasing lower quantities [2,3]. However, this correlation with released PS quantity is not always consistent. For instance, in several maize cultivars, iron deficiency tolerance was reported not to be related to the total amounts of secreted PS, which suggests the existence of other factors that control such a tolerance [4,5]. Other important but less-studied factors may also be involved in plant responses to iron deficiency such as root/shoot ratios [6], photoassimilate distribution, tissue Fe requirements for chlorophyll synthesis [7], Fe use efficiency for growth [8,9], and difference in PS chemical properties [10] since the chemical nature of these chelators can differ from one species to another and even from a cultivar to another [3].
Most studies on Fe deficiency have been carried out on crop plants and quite little is known about Fe nutrition strategies of wild plants that allow their survival on contrasting soil types. Wild plants are able to grow under severe environmental conditions although their growth rate is slower than that of most crops. They might have specific adaptations which are lacking in cultivated plants [11]. Hordeum maritimum (Poaceae) is an annual fodder species frequent in the Mediterranean basin and the European littoral [12]. In Tunisia, this plant is often found in the salt-affected depressions, where it significantly contributes to the annual biomass production [13]. This successful survival of the species in different ecosystems largely depends on its capacity to tolerate several environmental constraints.
The aim of the present study was to compare some traits of the adaptive mechanism to iron deficiency in wild and cultivated barley. The study was based on some usual parameters such as biomass production, root/shoot ratios, chlorophyll concentrations, iron status within tissues, organic solute accumulation, and PS release rates. Furthermore, to know whether the difference in the chemical nature of root exudates has an influence on the Fe(III) mobilization capacity in the two species, an iron mobilization test was performed at different pH values using an insoluble Fe form (Fe-hydroxide).
2 Materials and methods
2.1 Plant material and growth conditions
Seeds of H. maritimum (collected from Soliman Sebkha, 30 km south of Tunis, semi-arid stage) and H. vulgare were disinfected with calcium hypochlorite and germinated at 25 °C in Petri dishes on filter paper moistened with distilled water. Five-day old seedlings were then replicated on a Fe-free nutrient solution. Four days after iron chlorosis appearance in the youngest leaves, the half of plants was transferred into a Fe-supplied (100 μM Fe) medium, whereas the remaining ones were kept iron-deficient (0 μM Fe). The treatments were applied over 10 days under controlled growth chamber conditions (16 h of light at 25 °C and 8 h of dark at 22 °C and 60–75% of relative humidity).
2.2 Determination of chlorophyll concentration
Chlorophyll concentrations were measured in the youngest fully-expanded leaf according to the method described by Arnon [14]. The measurements were performed on days 0, 2, 4, 6, 8, and 10 of treatment, with three replicates for each treatment.
2.3 Root exudates collections
Root exudates collections were realized, as chlorophyll measurements, every 48 h from day 0 to day 10 of treatment. Two hours after the beginning of the light period, rinsed roots were washed for 30 min with aerated distilled water. Then a 4-hour incubation in 500 ml of the latter was performed to collect their exudates. An antimicrobial agent, Micropur (Roth, Karlsruhe, Germany), was then added to the collected root exudates, followed by a storage at −20 °C before the determination of PS release rate and iron mobilization capacity of each species.
2.4 Determination of PS release rates
Released PS quantities were indirectly determined by calculating the amount of Fe(III) mobilized from Fe(OH)3 with a modified method of Takagi [15]. An aliquot of 2 ml Fe(OH)3 solution and 100 μl from root exudates were added to a final volume of 10 ml. The resulting mixture was shaken for 1 h at room temperature then filtrated (Blue ribbon No. 5893, Schleicher and Schüll, Dassel, Germany). Mobilized iron was determined by ferrozine test. The absorbance measurement at 562 nm was recorded and PS release rates (μmol PS g−1 root FW 4 h−1) were calculated as Fe equivalents.
2.5 Plant harvest and iron content determination
Immediately after root exudates collection, roots of intact plants were carefully washed with EDTA (10 mM) solution for 10 min under continuous aeration to remove the apoplastic iron then rinsed with distilled water. Thereafter, plants were harvested and cut into roots and shoots for fresh and dry weight determination then ground with an agate grinder for nutrient extraction. The latter was performed by a nitric/H2O2 acid digestion (4:3, v/v). Fe contents were determined by a Perkine Elmer AAnalyst 100 Atomic Absorption Spectrophotometer.
2.6 Determination of soluble sugar contents
Carbohydrate changes are of particular importance because of their direct relationship with physiological processes such as photosynthesis and respiration. For this reason, we suggested to determine the total soluble sugar contents using anthrone reagent method according to Yemm and Willis [16]. Briefly, plant material (0.25 mg) was extracted with 80% ethanol solution. The extract was incubated for 30 min at 70 °C then centrifuged at 3000g at 25 °C for 30 min. 5 ml anthrone reagent were added to the supernatant then heated in a boiling water bath for 10 min. Samples were then ice-cooled for 10 min and incubated for 20 min at room temperature (25 °C). The optical density (OD) was read at 640 nm on a spectrophotometer (LABOMED, SPECTRO UVS-2700) after a standard curve was made using glucose.
2.7 Fe use efficiency calculation
Fe use efficiency (IUE) in shoots and roots was also considered as a parameter involved in the discrimination between wild and cultivated barley. It was determined according to Makmur et al. [17]. This parameter is generally calculated on the basis of biomass production and defined as the ratio of shoot or root biomass (g) to its Fe content (μmol). It can be also calculated on the basis of chlorophyll biosynthesis as the ratio of shoot chlorophyll content (mg) to shoot Fe content (μmol).
2.8 Statistical analysis
Data were subjected to a one-way ANOVA test using STATISTICA program and means were compared according to Duncan's test at 5% level of significance.
3 Results
3.1 Biomass production and leaf area expansion
Under iron deficiency, shoot and root biomass production in both species was significantly reduced as compared to the control (Fig. 1). In H. vulgare, shoot fresh weight was more reduced than that of roots (50 and 35% less than the control, respectively). More severe reductions were observed in H. maritimum in which shoot and root biomass production was decreased by 54 and 44%, respectively. Iron deficiency induced an increase in iron use efficiency for root growth in both species (Table 1). This parameter was decreased in shoots of wild barley and increased in those of cultivated one. Leaf area expansion was reduced by iron deficiency in both species with a more pronounced effect in H. maritimum than in H. vulgare (about 75 and 50%, respectively) (Fig. 2).
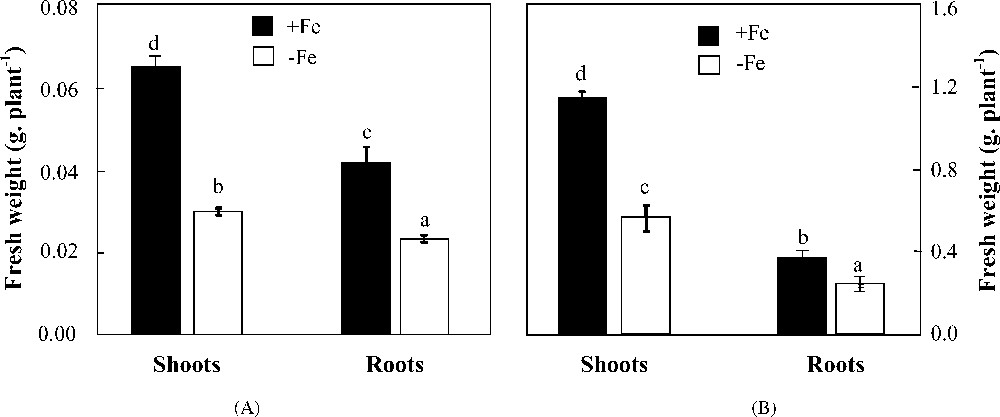
Effects of iron availability (+Fe = 100 μM and −Fe = 0 μM) in the medium on shoot and root fresh weights of H. maritimum (A) and H. vulgare (B). Bars are means of three replicates ±SE. Those with the same letters are not significantly different at p < 0.05 according to Duncan's test.
Effects of iron availability (+Fe = 100 μM and −Fe = 0 μM) on root/shoot fresh weight, soluble sugar content ratio, soluble sugar allocation (SS %), and Fe use efficiency calculated on the basis of chlorophyll biosynthesis (mg μmol−1 Fe) and shoot or root biomass production (g μmol−1 Fe) in H. maritimum and H. vulgare. Values are means of three replicates ±SE. Those with the same letters are not significantly different at p < 0.05 according to Duncan's test.
Treatment (μM Fe) | H. maritimum | H. vulgare | ||
100 | 0 | 100 | 0 | |
RootFW/shootFW | 0.63 ± 0.067c | 0.78 ± 0.027d | 0.32 ± 0.013a | 0.43 ± 0.035b |
RootSS/shootSS | 0.31 ± 0.025c | 0.47 ± 0.034d | 0.11 ± 0.024a | 0.202 ± 0.03b |
Chl (mg μmol−1 Fe) | 36.0 ± 1.70d | 13.0 ± 0.900c | 3.20 ± 0.180b | 1.30 ± 0.130a |
ShootFW (g μmol−1 Fe) | 5.40 ± 0.54c | 3.28 ± 0.080a | 3.80 ± 0.110b | 11.9 ± 0.740d |
RootFW (g μmol−1 Fe) | 0.96 ± 0.03a | 3.77 ± 0.130c | 1.94 ± 0.110b | 9.50 ± 1.090d |
Shoot SS (%) | 66 | 61 | 90 | 83 |
Root SS (%) | 34 | 39 | 10 | 17 |
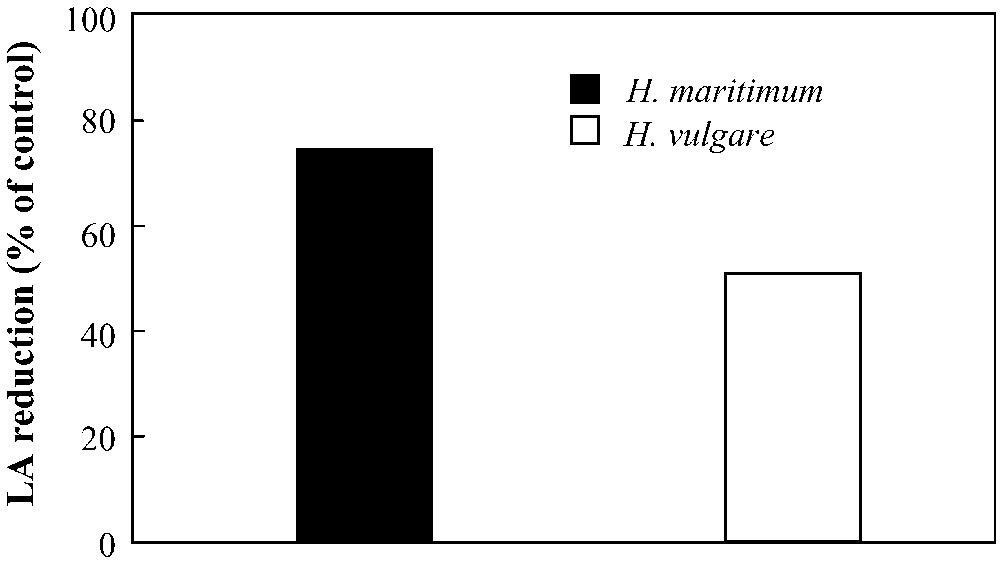
Effect of iron deficiency (+Fe = 100 μM and −Fe = 0 μM) on leaf area expansion (LA) in H. maritimum and H. vulgare.
3.2 Shoot and root soluble sugar contents
Iron deficiency altered shoot and root soluble carbohydrate concentrations in cultivated as well as in wild barley (Fig. 3). Those of shoots did not discriminate the two species since both of them showed the same decrease percentage (36%). However, a significant difference was observed in those of roots which were reduced by 12 and 21%, respectively in H. vulgare and H. maritimum. Such a difference in carbohydrate distribution within plants was measured as rootSS/shootSS ratio (Table 1). This parameter increased under iron deficiency conditions from 0.31 to 0.47 in H. maritimum and from 0.11 to 0.20 in H. vulgare, showing perfectly the same tendency of root/shoot fresh weight ratio and indicating a preferential allocation of photoassimilates towards roots in both species (Table 1).
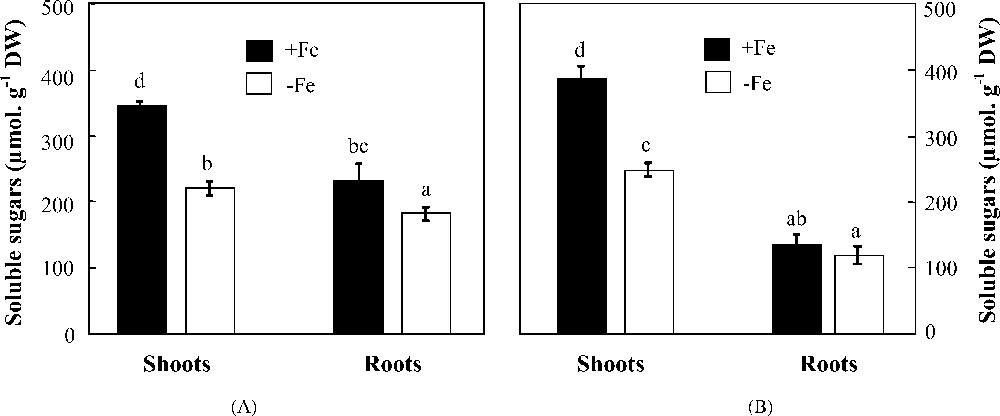
Effects of iron availability (+Fe = 100 μM and −Fe = 0 μM) in the medium on soluble sugar concentrations in shoots and roots of H. maritimum (A) and H. vulgare (B). Bars are means of three replicates ±SE. Those with the same letters are not significantly different at p < 0.05 according to Duncan's test.
3.3 Chlorophyll concentrations
Chlorophyll concentrations in young leaves were started to be followed up on day 0 of treatment (which corresponds to day 4 after iron chlorosis appearance) (Fig. 4). Iron supply on that day gradually alleviated the chlorophyll status of young leaves in both species. The comparison between them led to find out some differences in their behavior towards iron deficiency stress. Indeed, H. maritimum exhibited slower yellowing rate (decrease in leaf chlorophyll concentration under iron deficiency) and slower “regreening” rate (increase in leaf chlorophyll concentration after iron supply) over the first 4 days of iron supply as compared to H. vulgare although the latter exhibited much higher biomass production and leaf expansion. Regardless of the stress, iron use efficiency for chlorophyll biosynthesis was higher in wild barley than in cultivated one. Under iron starvation, this parameter was reduced in both species (Table 1).
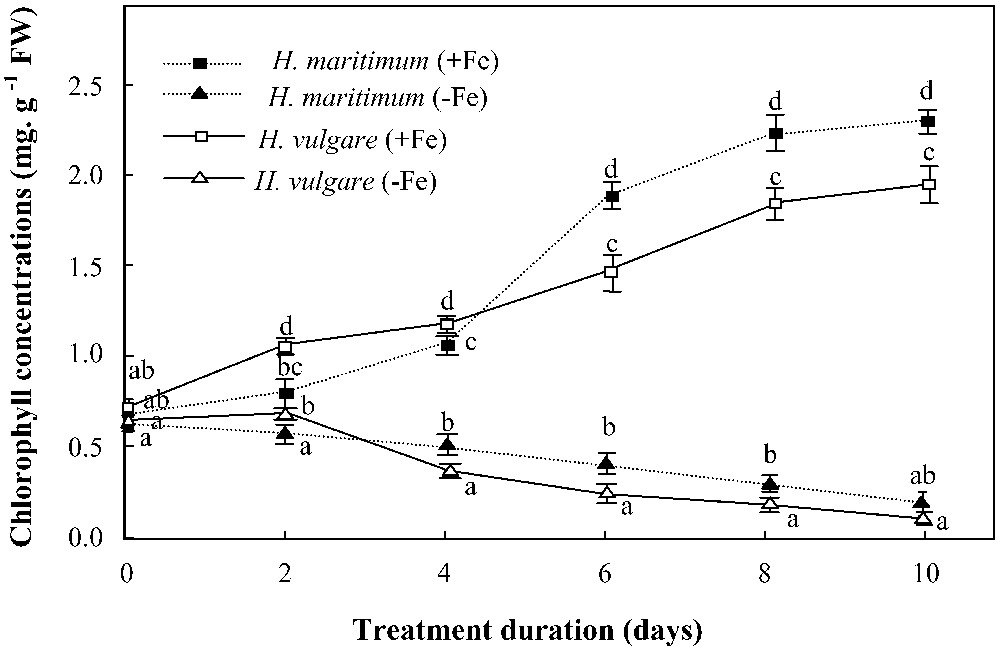
Effects of iron availability (+Fe = 100 μM and −Fe = 0 μM) in the medium on chlorophyll concentrations in young leaves of H. maritimum and H. vulgare. Bars are means of three replicates ±SE. Those of each date with the same letters are not significantly different at p < 0.05 according to Duncan's test.
3.4 Seed, shoot, and root iron status
Table 2 illustrates seed Fe concentrations and contents of two species. In terms of concentration, H. maritimum exhibited higher values as compared to H. vulgare, but in terms of content, the situation was inversed. These manifestations should be related to the difference between seed sizes.
Iron concentrations and contents in seeds of cultivated and wild barley collected from the native field (north–west Tunisia) and Soliman Sebkha (30 km south of Tunisia, semi-arid stage), respectively. Means of three replicates ±SE. Means followed by the same letters are not significantly different at p < 0.05 according to Duncan's test.
Fe concentration (μg/mg seed DW) | Fe content (μg/seed) | |
H. maritimum | 0.13 ± 0.020b | 0.39 ± 0.079c |
H. vulgare | 0.03 ± 0.001a | 1.13 ± 0.055d |
In Fe-sufficient plants, Fe concentrations were higher in wild than in cultivated barley, especially in roots (17 versus 11 μmol g−1 DW) (Fig. 5, A and B). Under iron deficiency, a noticeable difference in shoot Fe concentrations discriminated H. maritimum and H. vulgare; while the former maintained a constant Fe concentration, the latter showed a 66% decrease as compared to the control. Root Fe concentrations were also reduced under iron starvation conditions by 81 and 87%, respectively in the two species. Quantitatively speaking, Fe contents of both organs (μmol plant−1) deceased under iron deficiency in H. maritimum as well as in H. vulgare (Fig. 5, C and D). Nevertheless, obvious differences were found when comparing root and shoot iron contents in each species. Indeed, while in H. vulgare, both organs showed perfectly the same tendency of iron content reduction, in H. maritimum, roots exhibited a more pronounced decline than shoots. As a result, iron distribution within H. maritimum deficient plants was changed, whereas it remained practically unmodified within those of H. vulgare. Expressed as percentage of the total Fe uptake, shoot amount increased under Fe deficiency conditions from 20 to 53% and from 60 to 65%, respectively in wild and cultivated barley (Fig. 5, C and D).
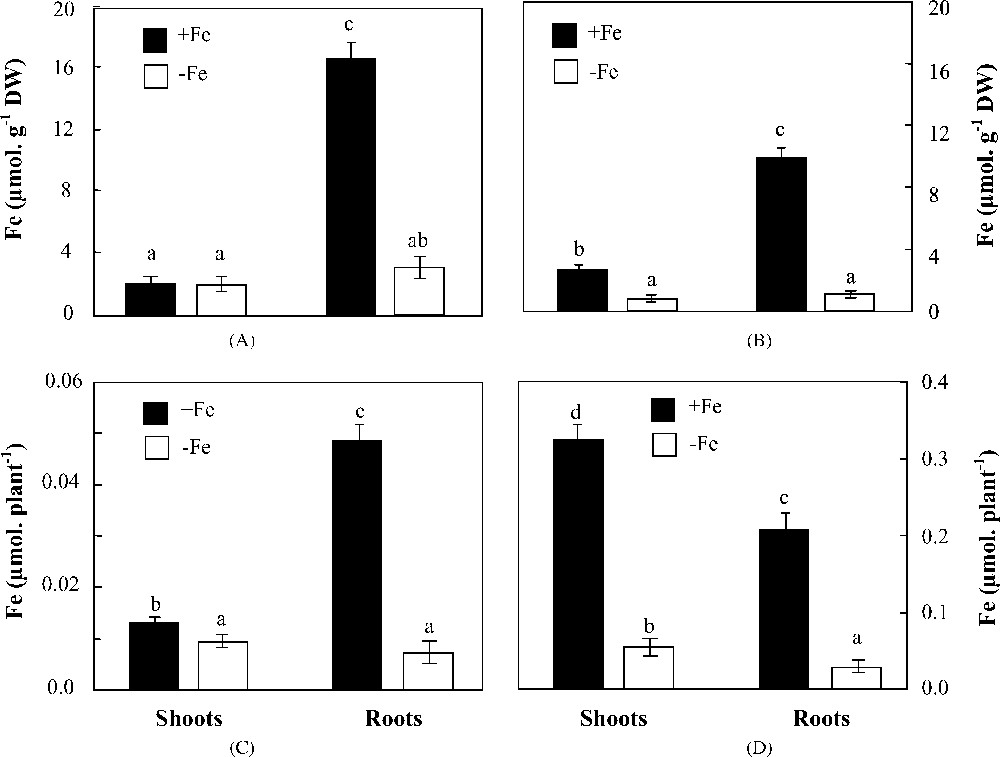
Effects of iron availability (+Fe = 100 μM and −Fe = 0 μM) in the medium on Fe concentrations and quantities in shoots and roots of H. maritimum (A and C) and H. vulgare (B and D). Bars are means of three replicates ±SE. Those with the same letters are not significantly different at p < 0.05 according to Duncan's test.
3.5 Phytosiderophore release rates
Under iron deficiency, PS secretion was early stimulated in both species (Fig. 6) and its rates reached at least 10 times that of the control, especially on day 8. As a whole, both curves showed the same form, but that of H. vulgare was constantly higher than that of H. maritimum. The difference between PS release rates was significantly high. Thus, one gram of H. vulgare roots released approximately 2-fold the amount of PS released by the same biomass of H. maritimum roots over 4 h.
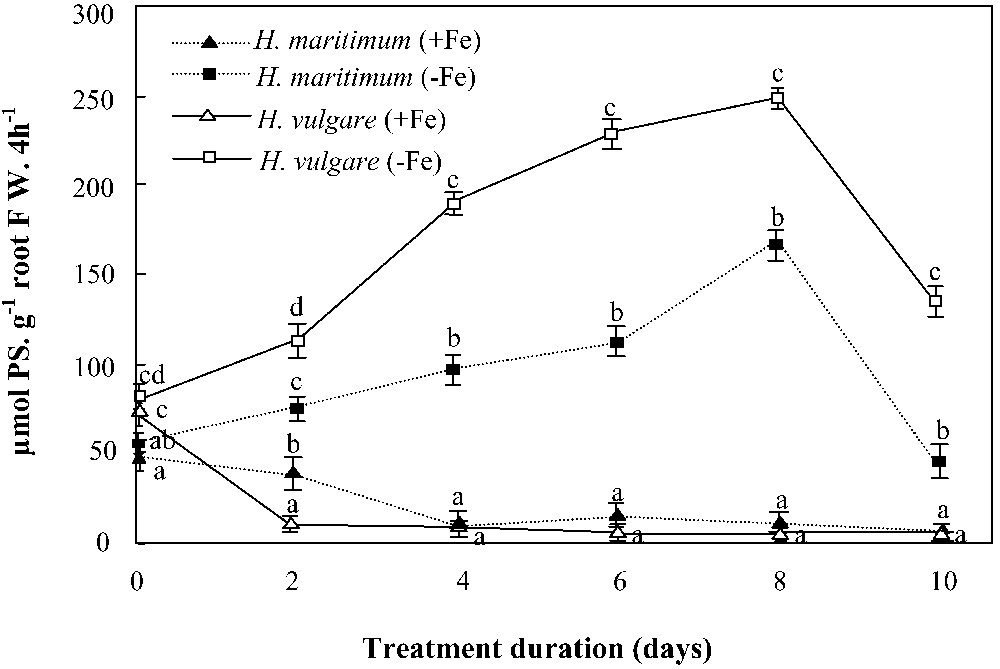
Effects of iron availability (+Fe = 100 μM and −Fe = 0 μM) in the nutrient solution on phytosiderophores (PS) release rates of H. maritimum and H. vulgare. Bars are means of three replicates ±SE. Those of each date followed by the same letters are not significantly different at p < 0.05 according to Duncan's test.
Iron addition to the nutrient solution gradually inhibited PS release in both species, but its effect was faster in H. vulgare than in H. maritimum (Fig. 6). In the former, PS release was stopped on the second day after iron supply, whereas, in the latter, the 2 days of iron feeding (100 μM Fe) were not enough to stop the signal of PS biosynthesis. This was concomitant with the difference in the rates of “regreening” of young leaves between the two species, expressed as chlorophyll contents (Fig. 4).
3.6 pH effect on iron mobilization capacity
The amounts of mobilized Fe3+ by 1 ml root washing of each species (collected by the same way during 4 h as described above) at a pH range of 5.6–8.6 are represented in Fig. 7. Distilled water was considered as blank. Over the entire pH range, the blank resulted in a non-significant mobilization of Fe3+. 1 ml of root washing collected from H. vulgare was able to mobilize about 3-fold the amount of Fe3+ mobilized by an equal volume of root washing collected from H. maritimum. This result strongly supports that of PS release rates which revealed higher values in H. vulgare than in H. maritimum (Fig. 6). Their mobilization capacities significantly decreased with the increasing pH (Fig. 7). However, the reduction as percentage of mobilized Fe3+ at pH 8.6 was higher in H. vulgare than in H. maritimum. Indeed, raising the pH value from 5.6 to 8.6 decreased the Fe mobilization by 5–30% and 12–50% in H. maritimum and H. vulgare, respectively (Fig. 7).
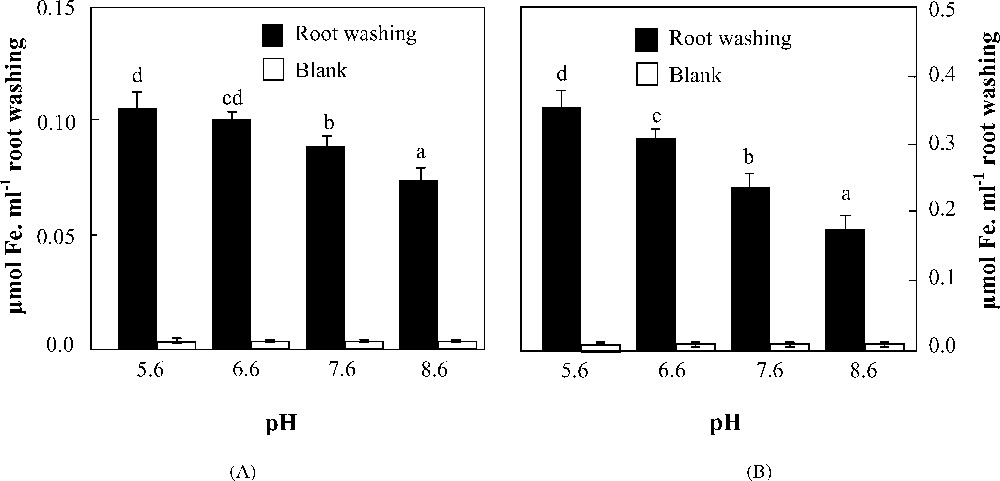
Effects of pH on Fe3+ mobilization from Fe(OH)3 by 1 ml root washing collected from H. maritimum (A) and H. vulgare (B). Bars are means of three replicates ±SE. Those with the same letters are not significantly different at p < 0.05 according to Duncan's test.
4 Discussion
4.1 Photoassimilates and biomass production
A noticeable iron-deficiency-induced decline in biomass production was observed in both shoots and roots of the two species. Indeed, Fe absence in the medium (direct iron deficiency) could not be tolerated even though the plants exhibited marked responses to enhance its availability for their roots. RootFW/shootFW ratios were increased under −Fe conditions, going up from 0.32 to 0.43 in H. vulgare and from 0.63 to 0.78 in H. maritimum (Table 1). These results are in agreement with those of Crowley et al. [6] who found that iron starvation altered shoot/root ratios in different barley cultivars and suggested this parameter as a criterion for iron-efficient plant selection. Van der Werf and Nagel [18] proved that when plants are grown at sufficient nutrient availability, they generally invest more biomass in shoots than roots, whereas at low nutrient availability, the opposite occurs. In comparison with H. vulgare and independently of iron concentration in the medium, H. maritimum exhibited higher root/shoot fresh weight ratios, which could be considered as a characteristic of this wild species often found in stressful natural biotopes [13–19]. The variations in Fe use efficiency for growth may support such a result since it was increased by 3 to 5 times in all organs of treated plants except H. maritimum shoots in which it was decreased by 39% (Table 1).
A decrease in soluble sugar concentrations was observed in Fe-deficient plants of both barley species. Similar decrease was previously reported in Heterosigma akashiwo [20]. Since iron is an essential component of several FeS proteins involved in chloroplast electron transfer chain [21], one may speculate that this decrease in soluble sugar concentrations in Fe-deficient plants could be explained by an alteration in chloroplasts. In this context, Andaluz et al. [22] found that chloroplast proteome composition was modified in response to iron deficiency; the amount of proteins from electron transfer complexes decreased, whereas that of proteins involved in carbon fixation increased. Another reason but less important is that sugar glycolysis increases via the activation of PEPC (phosphoenolpyruvate carboxylase) which is stimulated by iron deficiency [23,24] in order to feed the tricarboxylic acid cycle [25,26]. Besides, Espen et al. [27] and Rabotti et al. [28] found high carbohydrate catabolism under iron deficiency stress and attributed it to the activation of several glycolytic enzymes. Interestingly, organ growth responses were concomitant with a modification in soluble sugar distribution within the plant. Indeed, the sugar contents allocated towards the roots were increased in iron-deficient plants, especially in wild barley (Table 1), favoring root expansion. These results suggest that iron deficiency response requires an overall increase in carbon import to the roots. Besides, Crowley et al. [6] found that barley cultivars allocate significantly more carbon towards roots under moderate iron deficiency, which decreased shoot/root ratios. Similar results were found by Thimm et al. [29] in Arabidopsis thaliana and the authors suggested the induction of genes encoding products involved in carbon (sugars) mobilization and export from shoots to roots via phloem.
4.2 Chlorophyll concentrations and iron status
According to Shen et al. [30], the seed Fe content may play a significant role in the early establishment of iron chlorosis symptoms, especially in iron-deficient medium. Studying wheat cultivars, these authors found that seedlings obtained from seeds with low Fe contents showed earlier iron chlorosis as compared to those obtained from seeds with high Fe contents. In our case, this can be applicable only before starting treatments (up to day 0 of treatment). But, after this date, it seems that the seed iron content was exhausted in the two species, and therefore, iron chlorosis symptoms began to be rather dependent on leaf expansion. Indeed, during the pretreatment period, H. vulgare exhibited higher seed Fe content (Table 2) but also higher leaf expansion than H. maritimum (Fig. 2). This compensation between the two factors could partially explain why iron chlorosis symptoms (Fig. 4) appeared with the same degree of severity in both species up to day 0 of treatment (which corresponds to day 4 after iron chlorosis appearance). However, along the period of treatment, the rate of chlorophyll reduction was higher in H. vulgare than in H. maritimum, indicating a difference in the pigment dilution rates which was directly related to the dissimilarity in growth rates (leaf expansion) between the two species. According to Abadìa et al. [31], iron chlorosis is due to chlorophyll dilution when leaves continue to grow at a normal rate under iron deficiency conditions.
Plant Fe content (μg plant−1) was severely deceased by iron deficiency in H. maritimum as well as in H. vulgare. Consequently, a redistribution of this micronutrient within the plant was observed in wild barley. Its roots, which showed much higher Fe concentrations than shoots in iron-fed plants, exported a part of their Fe content to the photosynthetic organs in Fe-deficient plants. Similar results were recently found in chickpea varieties [8]. This suggests that iron deficiency tolerance is extremely related to the aptitude of plants to guarantee an adequate iron alimentation of their leaves [32]. Moreover, a higher leaf expansion rate was found in H. vulgare than in H. maritimum. This led to a pronounced dilution of the micronutrient within leaf tissues [33] in the former. Such a phenomenon did not occur in the latter. Thus, Fe concentration in shoots of H. vulgare was decreased by 66%, whereas that of H. maritimum was practically not modified. In addition, the higher values of Fe use efficiency for chlorophyll biosynthesis (Table 1) found in control and treated plants of H. maritimum as compared to those of H. vulgare, indicates that iron requirement for chlorophyll synthesis is lower in the former than in the latter. This physiological parameter was often used as a trait of resistance to iron chlorosis [8,9].
4.3 Phytosiderophore release and iron mobilization capacity
PS release was strongly correlated with iron status within the plant: the presence of iron in tissues inhibited the secretion of these natural chelators and vice-versa (Fig. 6). Thus, the absence of a modification in iron concentrations of H. maritimum shoots (Fig. 5) and their strong reduction in those of H. vulgare under iron deficiency conditions could explain, at least partially, the difference of PS release capacity between the two species. As it was suggested for the control of iron homeostasis in strategy I plants [34], the iron status of the plant is monitored by a sensor localized in the leaves. Under iron deficiency, this sensor triggers the synthesis and/or transport of a phloem mobile signal molecules to roots, where it induces PS biosynthesis and secretion. Although shoot iron concentration in Fe-deficient H. maritimum plants was equal to that of the control, PS exudation was not totally inhibited. Indeed, Schmidt [34] and Schmidt and Steinbach [35] suggested the presence of a second iron sensor localized in root cells that modulates the signal received from the shoots according to the presence of iron in the apoplast.
In principle, chlorosis resistance closely correlates with quantitative differences in Fe acquisition, which is associated with several ways among which the amount of released PS [2,3], root morphology adaptation [6], Fe–PS uptake mechanism across the plasma membrane [4], and the PS backbone which reflects Fe mobilization capacity [10]. Although H. maritimum produced very low levels of PS as compared to H. vulgare, it was found to grow under very severe environmental conditions. This suggests that H. maritimum has other traits which make it able to tolerate such constraining conditions. The rate of PS release could be a useful index for screening Graminaceous for their Fe deficiency tolerance, but it is not always regular. Indeed, in the maize mutant ys1, Von Wirén et al. [4] showed that, although it produced PS, it did not utilize Fe–PS complexes due to a defect in their transport mechanism across the plasma membrane.
Generally, Fe chlorosis that occurs in plants grown on calcareous soils results from two combined effects: (1) bicarbonate buffer effect and (2) iron low availability. Thus, Fe acquisition under such conditions depends not only on the amount of Fe-chelators released from the plant root system but also on their chemical nature. Results of Fe(III) mobilization test (Fig. 7) showed that H. maritimum iron mobilization capacity was less sensitive to alkalinity than that of H. vulgare. This difference may reflect the differential chemical nature of root exudates components of both species. According to Römheld and Marschner [3], the chemical nature of iron chelators can differ between species and even between cultivars. As reported by Von Wirén et al. [10], PS structure may influence iron acquisition in three principal ways: susceptibility to microbial degradation, affinity for the transport process across the root cell plasma membrane, or Fe(III) chelating proprieties. Previous studies neglect the influence of the two first ways [4,10,36]. The most efficient way which is still considered as a possible limiting factor causing discrimination among different PS species is Fe(III) chelating properties as affinity constants and pH effect on Fe(III) chelating capacity. Further biochemical studies are needed to identify the differences in the chemical nature of root exudates which can be responsible for the differences in Fe(III) mobilization capacities between the two species.
5 Conclusion
From these results, we can conclude that H. maritimum can probably survive at low iron supply using iron metabolism efficiency strategy, which is manifested by a flexibility of organ growth, a fine regulation of photoassimilate distribution between organs, an iron remobilization from roots and its transport to shoots, and a better Fe use efficiency for growth and chlorophyll biosynthesis. In addition, the less sensitivity of its Fe(III) mobilization capacity to alkalinity may guarantee a normal development on alkaline soils. However, H. vulgare tolerance to iron deficiency is especially based on its high phytosiderophore release capacity. Therefore, the correlation between iron deficiency tolerance and the quantity of released PS is not always consistent and each species (H. maritimum and H. vulgare) has its own strategy to tolerate iron deficiency.