1 Introduction
Climatic changes with global warming can all have a great negative impact on plant distribution and production and therefore, a great interest is devoted to the study of plant response to abiotic stresses and to their adaptive strategies. However, most of the investigations are made applying a single stress factor to plants under laboratory conditions. According to Miller et al. [1], this approach could not be adequate to fully explore plant tolerance and adaptive mechanisms. In fact, abiotic stresses often occur in combination in nature, with the different stress factors cooperating together [2], and plants activate specific stress response in the presence of multiple stresses [3]. For these reasons, the effect of different stress combinations is being more and more researched [1]. An alternative method could be the study of plants living in natural and extreme environments, like geothermal alteration fields, where different stressors naturally coexist. The few plants able to survive in these extreme environments develop specific morpho-anatomical and physiological traits [4]. Geothermal alteration fields occur in few and sometimes geographically isolated areas of the world and constitute very extreme environments frequently characterized by surface geothermal manifestations. These comprise hot emissions of water steam mixed with different gases, toxic elements and compounds (e.g. CO2, CH4, NH3, H2S, Rn, As, Hg, H3BO3, etc.) that violently and unpredictably escape from soil fractures and then condensate, affecting large areas around fumaroles [5]. Additionally, marked soil heating and acidity, low content of carbon, nitrogen and phosphorus, high concentrations of heavy metals and trace elements in soil, are common factors in these sites [5,6]. High soil temperature and soil acidity, in particular, are important environmental constraints limiting growth and productivity of plants [7], as heated and hyperacid soils affect the physiology and the growth of both shoot and root [8]. An inhibition in root growth, a decrease in hormone synthesis and transport, a reduction in the uptake of water and nutrients were observed in plants subjected to these stressors [8–10]. The need for an efficient conservation of nutrients in these particular conditions can reflect on functional characters, resulting in low specific leaf area, SLA, and high leaf dry matter content (LDMC) [11].
Constraints of geothermal alteration field can cause oxidative damage by overproduction of reactive oxygen species (ROS) [4]. Leaf injury has been associated with pronounced lipid peroxidation and decrease in antioxidative activity, particularly, in plants with roots exposed to high temperatures [12]. All these effects together with decreased antioxidant activity can result in severe senescence and damages to leaves [13]. To counteract the damaging effects of ROS, plants have evolved multiple antioxidant defence mechanisms comprising phenols, important protective molecules [14] and low molecular weight antioxidants, such as ascorbate and glutathione, which can cooperate with enzymes to protect plants from oxidative injury [15].
The Mediterranean shrub Cistus salviifolius L., eliophilous and pyrophilic species [16], is one of the few plants able to survive in the fumarolic field of Sasso Pisano (Central Western Italy), one of the most extreme geothermal fields in terms of both soil acidity and hot temperatures [17]. This plant has been demonstrated as able to adopt adaptive strategies in relation to environmental constraints [18] and therefore, it can be considered as a useful model to explore plant response to environmental stress.
Our aim was to answer the following question: which are the morpho-functional and physiological traits putatively involved in adaptation of plants to a natural multi-stressed environment, as geothermal fields?
To address this question, morphological, functional and physiological parameters were analysed in mature leaves of C. salviifolius sampled from plants grown around fumaroles (G leaves) and in mature leaves developed by the same plants after transferring into a growth chamber under controlled conditions (C leaves).
2 Materials and methods
2.1 Site description
Plants of C. salviifolius L. were collected in the geothermal alteration field near to Sasso Pisano town (Castelnuovo Val di Cecina, Pisa province, Italy), in the site of Regional Interest (RIS B12, IT 5160103) named “Monterotondo Marittimo and Sasso Pisano geothermal fields”, hosting two types of natural habitats listed in the Natura 2000 EU directive: the “Forests of Castanea sativa Miller (cod 9260)” and the “Lava fields and natural cavities (cod 8320)” [19]. The sampling site is characterised by emissions of steam vents containing H2S, CO2, boric acid and water vapour from cracks in the rocks with surface soil temperature from 50 °C to 100 °C and extremely low pH values (pH = 1.13) [17].
The considered geothermal alteration field is part of the geothermoelectric basin of Larderello, in southern Tuscany. The geological structure of the Larderello geothermal area is described in Bertini et al. [20].
The sampling site is located in the upper valley of Cornia river, at an elevation of about 550 m a.s.l. The climate is Mediterranean, with a mean annual temperature of 13.3 °C [21] and a mean annual rainfall of 1107 mm [17]. At the time of the sample collection (May 2011), the mean monthly temperature recorded was 16.3 °C (min: 10.3 °C; max: 22.5 °C) and the mean monthly rainfall was 7.0 mm (data from the wheather station of Castelnuovo Val di Cecina, Pisa available at http://www.castelnuovometeo.it/) and the monthly averaged daily mean global insulation upon a normal surface was 7780.1 Wh/m2 (data from “Atlante Italiano della radiazione solare”, edited by “ENEA, Agenzia nazionale per le nuove tecnologie, l’energia e lo sviluppo economico sostenibile”, available at http://www.solaritaly.enea.it/index.php).
In the sampling site, the daily temperature of the soil was recorded 30.4 °C (min: 25 °C; max: 35 °C) and soil pH was 3.8 [4].
2.2 Plant material
In May 2011, ten healthy plants of C. salviifolius, similar in size, growing near the fumaroles, were screened. The selected plants were then explanted, transferred in pots filled with perlite, after the complete removal of the native soil, and kept for 30 days inside a growth chamber (BINDER, KBW 400, Germany), under controlled conditions (12:12 h light:dark photoperiods, a day/night temperature of 25 °C, an estimated irradiation of 6510 Wh/m2). Plants were weekly watered with 1/2 × Hoagland's solution (Sigma).
Mature leaves were sampled from plants before the explants (G leaves) and from the same plants transferred to controlled conditions (C leaves) for anatomical and morpho-functional analyses (fresh material or chemically fixed) and for physiological determinations (fresh materials or fixed in liquid nitrogen and stored at –20 °C until use).
2.3 Light and scanning electron microscopy
Leaf portions were excised from G and C leaves and were fixed for 24 h in FAA fixative (10 % formaldehyde–5 % acetic acid–45 % ethanol), dehydrated in a graded ethanol series, and embedded in LR-White medium Grade (London Resin Company). Semi-thin sections (3 μm) were cut with the ultramicrotome Ultratome Nova LKB using glass knives. The sections were stained with toluidine blue O (0.05 % in 100 mM benzoate buffer at pH 4.4) for histological characterisation [22]. The sections were cleared in xylene, air dried, mounted in DPX Mountant (Sigma) and then observed with a LEITZ DIAPLAN light microscope. At least 100 histological sections for each experimental group were analysed. Images of each slide were taken using a Leica DFC 420.
For surface investigations on fresh leaf pieces, a Leica M165 C stereomicroscope fitted with a Leica DFC 420 was employed.
For scanning electron microscopy (SEM) observations, leaf portions from the two experimental groups were fixed in 3% glutaraldehyde in 100 mM sodium phosphate buffer (pH 7.4) for 24 h and then dehydrated in a graded ethanol series. After critical point drying, leaf portions were collected on stubs through a double adhesive tape and subsequently they were coated with gold, examined and captured with a JEOL/JSM-5410 scanning electron microscope.
2.4 Morpho-functional traits
Epidermal stripping of both adaxial and abaxial surfaces of fresh leaves were made to determine stomatal density (SD), expressed as the number of stomata per square millimetre leaf area [23] and hair density (HD), expressed as the number of hairs per square millimetre leaf area. For each experimental group, 20 leaves collected from the selected plants (10 individuals) were analysed. Counts were made on both surfaces of the leaves for a total of 40 counts per experimental group.
Twenty histological median cross-sections randomly collected from 10 leaves per plant accession were used for the determination of total leaf thickness (TLT), palisade parenchyma thickness (PPT), adaxial and abaxial epidermis thickness (ADE and ABE), all expressed in μm. Analyses were performed by using the open source UTHSCSA Imagetool software (available at http://ddsdx.uthscsa.edu/dig/itdesc.html).
Leaf area (LA) was the one-sided projected surface area of a fresh leaf, expressed in mm2. Specific leaf area (SLA) was the one-sided area of a fresh leaf divided by its oven dry mass (DW = dry weight), expressed in mm2/mg. Leaf dry matter content (LDMC) was the oven dry mass of a leaf (DW) divided by its water-saturated fresh mass (TW = turgid weight), expressed in mg/g. Succulence index (SI) was calculated as the ratio of the difference between the oven dry mass of a leaf and its water-saturated fresh mass to the leaf surface area, expressed in mg/cm2 [24,25]. The following formulae are reported:
For each accession, 20 leaves from 10 individuals were collected. Sample storing and processing followed the standardised methodologies detailed by Cornelissen et al. [26]. Leaf projected area was acquired with a CanoScan LiDE 90 (Canon) and determined by CompuEye, Leaf & Symptom Area software (available at http://www.ehabsoft.com/CompuEye/LeafSArea/). For calculating mean and standard error, the average SD, HD, TLT, PT, ADE, ABE, LA, SLA, LDMC and SI for each individual plant was one statistical observation.
2.5 Determination of water content and of relative water content
Calculations of leaf fresh weight, dry weight and moisture content were based on weights determined before and after oven drying of leaf samples at 100 °C for 24 h. Water content percentage was estimated on the fresh weight basis. Leaf relative water content (RWC), [27, modified] was calculated from the formula:
Fresh weight was obtained by weighing the fresh leaves. The leaves were then immersed in water overnight, blotted dry and then weighed to get the turgid weight. The leaves were then dried overnight in an oven at 100 °C and reweighed to obtain the dry weight.
2.6 Pigment determination
Chlorophylls (a, b and total) and carotenoids were extracted in 80% acetone and determined according to Hassanzadeh et al. [28] and Lichtenthaler [29], respectively. Then, 100 mg of fresh leaves were homogenised and the extracts were centrifuged for 10 min at 6000 g at 4 °C. The supernatants were collected and the pellets were resuspended and extracted with 80% acetone until they resulted colourless. The collected supernatants were read using spectrophotometer at 645, 663 and 470 nm. Pigment contents were expressed as mg/g DW.
2.7 Extraction and determination of hydrogen peroxide
H2O2 content of leaves was determined according to Jana and Choudhuri [30]. Leaves (250 mg) were ground in a mortar and homogenised with 15 mL of phosphate buffer 50 mM pH 6.5. The homogenate was centrifuged at 6000 g for 25 min. To determine the H2O2 content, 3 mL of extracted solution were mixed with 1 mL of 0.1% titanium chloride in 20% (v/v) H2SO4, then, the mixture was centrifuged at 6000 g for 15 min and the supernatant absorbance at 410 nm was read. The amount of H2O2 in the extracts was calculated from a standard curve and expressed as μmol/g DW.
2.8 Lipid peroxidation
The amount of lipid peroxidation products in leaves was estimated by determining the malonyldialdehyde (MDA) content in the leaves according to Hartley-Whitaker et al. [31] with minor modifications as in Spanò et al. [32]. Powder from freeze-dried leaves material (10 mg) was mixed with TBA reagent (4 mL of 10% w/v trichloroacetic acid + 0.25% w/v thiobarbituric acid), heated (95 °C for 30 min), cooled for 15 min and centrifuged at 2000 g for 15 min. The level of MDA (155 mM/cm extinction coefficient) was measured as specific absorbance at 532 nm, by subtracting the non-specific absorbance at 600 nm [33] and expressed as nmol/g DW.
2.9 Extraction and determination of phenols
Total phenols were measured according to Arezki et al. [34]. Phenolic extracts were obtained after centrifugation of frozen leaf samples homogenised in HCl 0.1 N and left at 20 °C for 3 h. Then, 300 μL of extract were added to 1.5 mL H2O + 0.1 mL Folin–Ciocalteu reagent and left so for 3 min. After incubation at 100 °C for 1 min in the presence of 400 μL Na2CO3 (20%), samples were cooled and the absorbance was measured. The level of phenolic compounds was determined spectrophotometrically at 750 nm and calculated as equivalent of gallic acid (GAE, mg/g DW) referring to a standard curve.
2.10 Extraction and determination of ascorbate and dehydroascorbate
Ascorbate (ASA) and dehydroascorbate (DHA) extraction and determination were performed according to Kampfenkel et al. [35] with minor modifications. Briefly, the leaves (500 mg) were ground in a chilled mortar and homogenised with 3.75 mL of 5% (w/v) TCA. The homogenate was centrifuged at 12 000 g for 10 min at 4 °C and the supernatant was used for the determination at 525 nm [35]. Total ascorbate was determined after reduction of DHA to ASA by dithiothreitol and DHA level was estimated on the basis of the difference between total ascorbate and ASA value. Calculations were made on the base of a standard curve and correction was made for colour development in the blank (absence of sample). Content was expressed as mg/g DW.
2.11 Extraction and determination of glutathione
Glutathione was extracted and determined according to Gossett et al. [36]. The leaves (500 mg) were homogenised in 1.5 mL of ice-cold 6% (w/v) m-phosphoric acid (pH 2.8) containing 1 mM ethylenediaminetetraacetic acid (EDTA). The homogenate was centrifuged at 20 000 g for 15 min at 4 °C and the supernatant was collected and stored in liquid nitrogen until use. Total glutathione (GSH + GSSG) was determined by the 5,5′-dithio-bis-nitrobenzoic acid (DTNB)-glutathione reductase recycling procedure [37] and the reaction was monitored as the rate of change in absorbance at 412 nm. GSSG was determined after removal of GSH from the sample extract by 2-vinylpyridine derivatisation. GSH was detected by subtracting the amount of GSSG from total glutathione and calculations were made on the base of a standard curve. A blank was made in the absence of the extract and content was expressed as nmol/g DW.
2.12 Enzyme extraction and assays
Acetonic powders were made according to Saari et al. [38] with modifications as in Bartoli et al. [4]. Briefly, the leaves were homogenised in ice-cold acetone. Homogenates were filtered through a Buckner filter with Macherey–Nagel MN 618 filter paper under vacuum and washings were repeated until the powder resulted colourless. After the complete removal of acetone under vacuum, acetonic powders were extracted in 100 mM potassium phosphate buffer pH 7.5 containing 1 mM EDTA, and 1% (w/v) polyvinylpyrrolidone (PVP-40) as in Spanò et al. [39]. All the extractions were performed at 4 °C. The homogenate was then centrifuged at 15 000 g for 20 min. For ascorbate peroxidase, 2 mM ascorbate was added to the extraction medium. For glutathione reductase, the supernatant was desalted on a Sephadex G-25 column. Supernatants were collected and stored in liquid nitrogen until their use for enzymatic assays.
Ascorbate peroxidase (APX) activity was measured according to Nakano and Asada [40] with modifications. Enzyme activity was assayed from the decrease in absorbance at 290 nm (extinction coefficient 2.8 mM/cm) as ascorbate was oxidised. The reaction mixture contained 100 mm potassium phosphate pH 7.5, 0.5 mm ascorbate and enzyme extract (25 μg protein/mL). The reaction was started by adding 0.2 mM H2O2. Correction was made for the low, non-enzymatic oxidation of ascorbate by hydrogen peroxide (blank).
Dehydroascorbate reductase (DHAR) activity was determined as described by Nakano and Asada [40]. The activity of DHAR was determined by monitoring the glutathione-dependent reduction of dehydroascorbate. Enzymatic extract contained 12.5 μg protein/mL. The activity was determined by measuring the increase in absorbance at 265 nm for 3 min. Specific activity was calculated from the 14 mM/cm extinction coefficient. A correction for the non-enzymatic reduction of DHA by GSH was carried out in the absence of the enzyme sample (blank).
Glutathione reductase (GR) activity was determined as described by Rao et al. [41], following the oxidation of NADPH at 340 nm (extinction coefficient 6.2 mM/cm). Enzymatic extract contained 25 μg protein/mL. A correction for the non-enzymatic reduction of GSSG was carried out in the absence of the enzyme sample (blank).
Ascorbate oxidase (AO) activity was measured as described by Moser and Kanellis [42]. The activity was determined by monitoring ASA oxidation following the decrease in absorbance at 265 nm for 3 min. Enzymatic extract contained 50 μg protein/mL. Specific activity was calculated from the 14 mM/cm extinction coefficient.
Glutathione peroxidase (GPX) activity was determined according to Navari-Izzo et al. [43], following the oxidation of NADPH at 340 nm (extinction coefficient 6.2 mM/cm). Enzymatic extract contained 25 μg protein/mL.
Catalase (CAT) activity was determined as described by Aebi [44]. Enzymatic extract contained 100 μg protein/mL. A blank containing only the enzymatic solution was made. Specific activity was calculated from the 23.5 mM/cm/extinction coefficient.
All enzymatic activities were determined at 25 °C and expressed as U/g protein. Protein measurement was performed according to Bradford [45], using BSA as standard.
2.13 Statistical analysis
Morpho-functional and physiological leaf traits were compared using the non-parametric test of Wilcoxon–Mann–Whitney. All the statistical tests were performed using the software R 2.14.0 (R Development Core Team. 2011. R: A language and environment for statistical computing. R Foundation for Statistical Computing. Vienna, Austria. http://cran.r-project.org/).
3 Results
3.1 Leaf anatomy and functional traits
A crimped and partially rolled lamina, forming several abaxial crypts (Fig. 1a, c, e), was observed in G leaves that also displayed a significantly greater thickness than the C leaves, which were characterised by large, thin and flat lamina (Fig. 1b, d, f and Table 1). Stellate trichomes occurred in adaxial surfaces of both G and C leaves (Fig. 1c, d), while dendritic trichomes, significantly more abundant in G leaves, occurred in leaf abaxial surface (Fig. 1e, f and Table 1). Leaves of C. salviifolius were amphistomatic (Fig. 2a–d) and G leaves, characterised by stomata of heterogeneous size (Fig. 2b), showed the greatest adaxial and abaxial stomatal densities (Table 1). Additionally, these leaves showed significantly higher values of LDMC and SI than C ones that, on the contrary, exhibited the greatest values of both LA and SLA (Table 1).
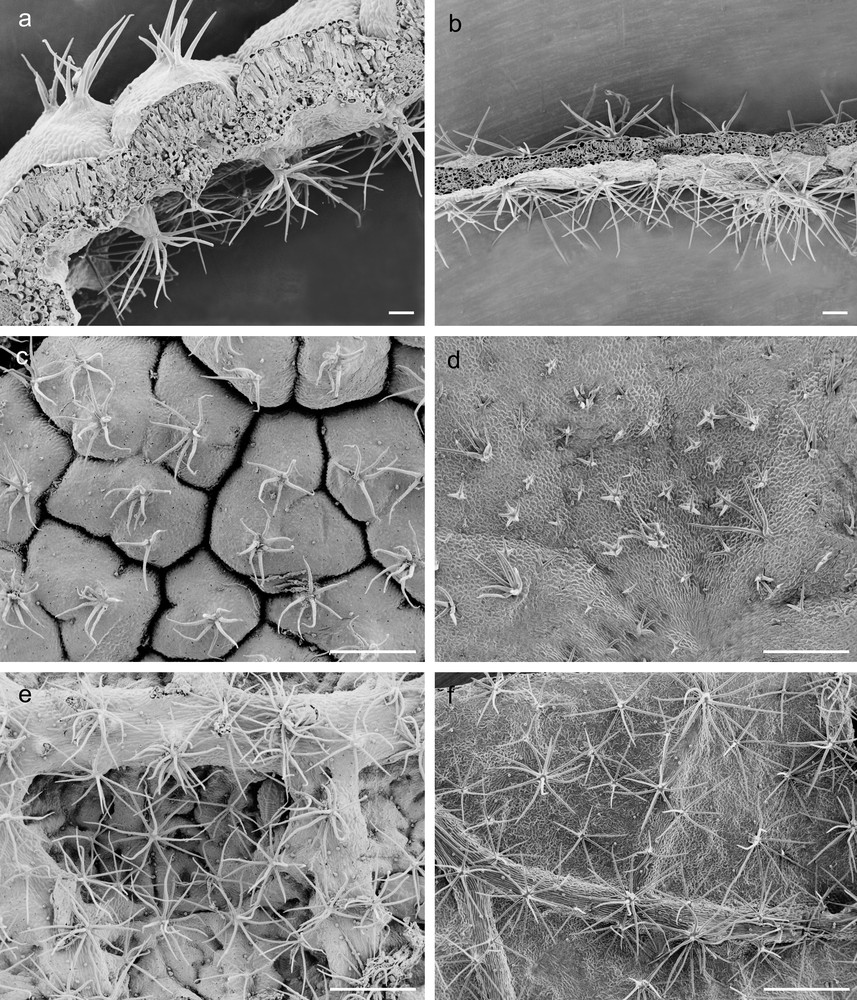
Scanning electron micrographs of G and C leaves of Cistus salviifolius. Cross-medial sections of G (a) and C (b) leaves (scale bars = 100 μm); adaxial and abaxial epidermis of G (c, e) and C (d, f) leaves (scale bars = 500 μm).
Morpho-functional traits of leaves from plants living in the geothermal field (G leaves) and from plants transferred into the laboratory (C leaves).
Morpho-functional traits | G leaves | C leaves |
Adaxial SD (stomata/mm2) | 210 ± 14a | 170 ± 26a |
Abaxial SD (stomata/mm2) | 455 ± 18a | 220 ± 13b |
Total SD (stomata/mm2) | 333 ± 10a | 195 ± 19b |
Adaxial HD (hairs/mm2) | 5.7 ± 0.3a | 4.3 ± 0.2b |
Abaxial HD (hairs/mm2) | 6.9 ± 0.2a | 6.5 ± 0.2a |
Total HD (hairs/mm2) | 6.3 ± 0.2a | 5.4 ± 0.1b |
TLT (μm) | 410.66 ± 13.90a | 180.98 ± 4.90b |
PPT (μm) | 197.74 ± 4.40a | 73.44 ± 1.93b |
ADE (μm) | 21.58 ± 0.44a | 19.78 ± 0.40a |
ABE (μm) | 15.91 ± 0.60a | 16.72 ± 0.36a |
LA (mm2) | 293 ± 20b | 635 ± 49a |
SLA (mm2/mg) | 2.6 ± 0.1b | 8.8 ± 0.4a |
LDMC (%) | 34.5 ± 0.9a | 15.4 ± 0.5b |
SI (mg/cm2) | 25.2 ± 0.4a | 9.9 ± 0.5b |
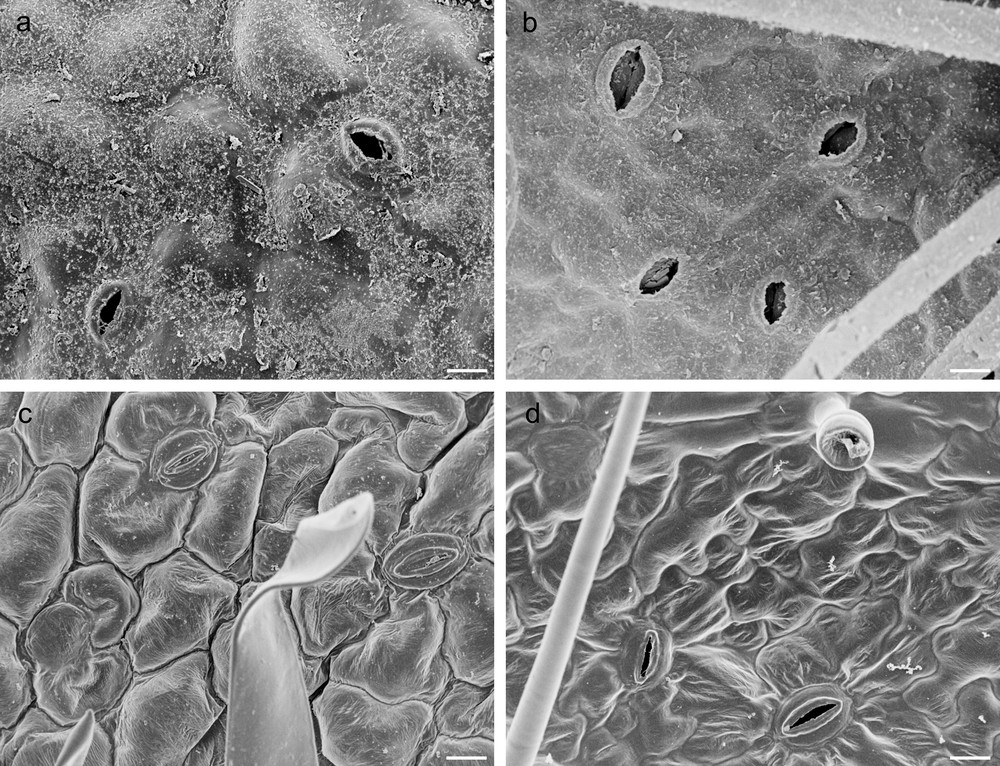
Scanning electron micrographs of G and C leaves of Cistus salviifolius. Adaxial and abaxial epidermis in G (a, b) and in C (c, d) leaves (scale bars: a, b, c, d = 50 μm).
The thickness of adaxial and abaxial epidermis did not differ significantly between G and C leaves (Table 1), but epidermal cells of G leaves were covered by an abundant and roughened cuticle, mainly on the adaxial side, and showed thick walls with abundant pectins, (Fig. 1a, c, e; Fig. 2a, b; Fig. 3a, c, d). G leaves were, moreover, characterized by a densely packed and multilayered palisade parenchyma, consisting of highly elongated cells with few and small chloroplasts, and by a spongy parenchyma with small intercellular spaces (Fig. 3a). On the contrary, C leaves showed smooth cuticle (Fig. 2c, d), thin-walled epidermal cells (Fig. 3b, e, f) and a relaxed mesophyll, consisting of a bi-layered palisade parenchyma, characterised by short cells mainly in the inner layer, and a spongy parenchyma with very large intercellular spaces (Fig. 3b). Both tissues were characterised in C leaves by many and large chloroplasts (Fig. 3b).
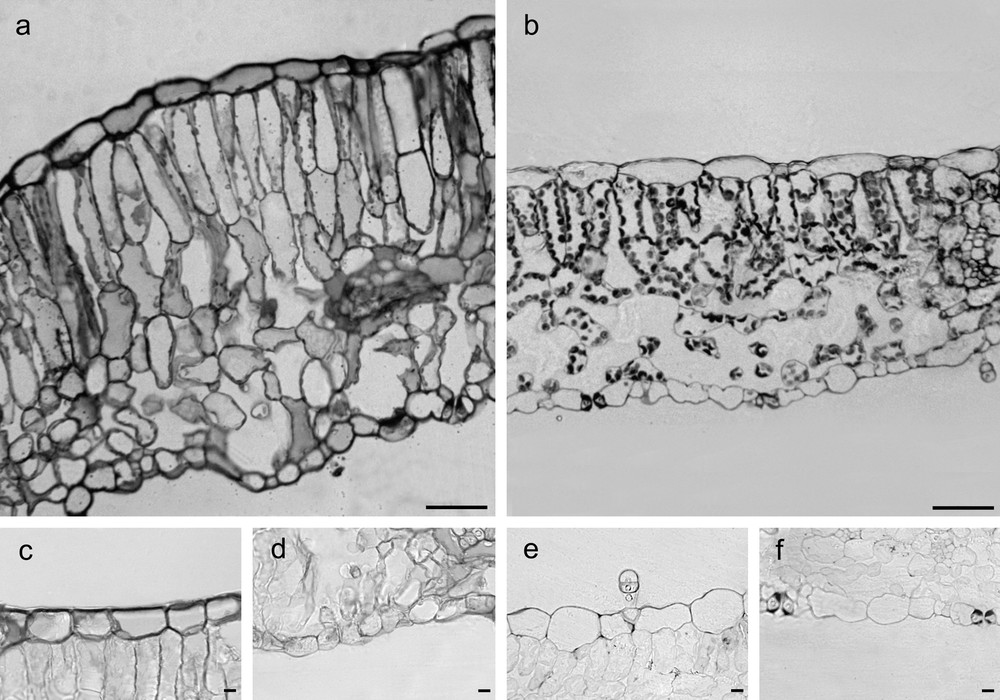
Cross-sections of G (a) and C (b) leaves of Cistus salviifolius (toluidine blue O staining, scale bars = 50 μm). Cross-sections showing pectic substances in cell walls from G (c, d) and C (e, f) leaves. (ruthenium red staining, scale bars = 10 μm).
3.2 Leaf water content, RWC and pigments
While water content (Table 2) was significantly higher in C leaves than in G ones, there was no significant difference in RWC (Table 2) between the two materials. The content of total chlorophyll and of carotenoids (Table 2) were about four-fold higher in C leaves than in G ones that showed also a higher Chla/Chlb ratio.
Physiological characteristics of leaves from plants living in the geothermal field (G leaves) and from plants transferred into the laboratory (C leaves).
Parameter | G leaves | C leaves |
H2O content (%) | 71.47 ± 0.53b | 88.00 ± 0.12a |
RWC (%) | 78 ± 3.05a | 85 ± 2.06a |
Total chlorophyll (mg/g DW) | 3.95 ± 0.058b | 15.88 ± 0.70a |
Chlorophyll a/chlorophyll b | 3.45 ± 0.11a | 2.79 ± 0.07b |
Carotenoids (mg/g DW) | 0.89 ± 0.03b | 3.47 ± 0.18a |
Carotenoids/total chlorophyll | 0.22 ± 0.01a | 0.22 ± 0.01a |
3.3 Oxidative stress and antioxidant response
ROS production was monitored by measuring the concentration of hydrogen peroxide (Table 3). The content of this signalling molecule was significantly higher in G leaves than in C leaves, showing a much stronger stress condition in the first ones. The measurement of lipid peroxidation (Table 3) was detected as MDA nmol/g DW: damage was approximately 3.4 times higher in G leaves than in C ones.
Oxidative stress and antioxidant response in leaves from plants living in the geothermal field (G leaves) and from plants transferred into the laboratory (C leaves).
G leaves | C leaves | |
Hydrogen peroxide (μmol/g DW) | 1020.84 ± 1.48a | 86.83 ± 1.13b |
TBARS (nmol/g DW) | 513.67 ± 14.31a | 150.33 ± 1.67b |
Phenols (mg GAE/g DW) | 26.83 ± 0.38a | 7.16 ± 0.98b |
Total ascorbate (mg/g DW) | 16.36 ± 0.34a | 2.98 ± 0.03b |
ASA/DHA | 6.72 ± 0.98a | 2.53 ± 0.20b |
Total glutathione (nmol/g DW) | 270.07 ± 8.64b | 353.83 ± 15.53a |
GSH/GSSG | 9.25 ± 1.15a | 1.01 ± 0.37b |
APX (U/g protein) | 204.10 ± 10.20a | 145.00 ± 3.00b |
DHAR (U/g protein) | 599.95 ± 42.65a | 600.00 ± 17.15a |
GR (U/g protein) | 11.35 ± 0.50a | 14.00 ± 1.00a |
GPX (U/g protein) | 645.17 ± 45.17a | 606.35 ± 19.35a |
AO (U/g protein) | 17.00 ± 0.00a | 20.00 ± 2.45b |
CAT (U/g protein) | 37.45 ± 0.00a | 17.02 ± 0.01b |
The antioxidant level was assessed by monitoring the variation of phenols and ascorbate/glutathione cycle metabolites (Table 3). Phenol content, measured as gallic acid equivalents, was significantly higher in G than in C leaves. The same also occurred for ascorbate pool, due mainly to the higher content of the reduced form of this low molecular weight antioxidant. In accordance, ASA/DHA ratio was significantly higher in G than in C leaves. Anyway, the content of total glutathione was significantly higher in C than in G leaves that, on the other hand, showed a significantly higher GSH/GSSG ratio.
Results of enzymatic assays are shown in Table 3. The activity of APX was higher in G than in C leaves. The other enzymatic activities were not significantly different between the two materials with the exception of the low CAT activity that was about two-fold higher in G leaves than in C ones.
4 Discussion
The comparative analysis of morpho-functional and physiological characters plastically expressed in leaves of C. salviifolius developed in a geothermal habitat or under controlled conditions, has made possible to highlight some key traits involved in the adaptive strategy of this plant to a complex multi-stress habitat, such as the geothermal ecosystem. Some parameters were differentially regulated in G and C leaves and seemed to be strictly correlated with stress conditions and with the ability of plant to withstand environmental constraints.
As observed in the leaves of some plants living in drought conditions [46,47], the lower values of LA of G leaves in comparison with C leaves, the crimping and the enrolling of the lamina, that reduces the size of G leaves, could help in reducing water loss. The water storage ability of tissues from G leaves could be further improved by the thick and compact mesophyll [48,49] and by pectic substances occurring in epidermal cell walls. This was confirmed by the lower values of SLA and the higher values of LDMC and SI of G leaves, typical of plants subjected to drought stress [26]. The higher number of stomata recorded in G leaves, apparently in contradiction with the need to preserve water balance, has been however detected in other plants living in the geothermal field, in strict proximity to fumaroles [4]. In the peculiar conditions of this environment, in which plants are subjected to sudden hot steam emissions, the ability to cool leaves through increase in transpiration seems to be a priority over the need to control water balance. However, this balance was not compromised in G leaves as indicated by the value of RWC, useful indicator of hydric state of plants [50], not significantly different between G and C leaves. In fact, in plants living in geothermal habitat, an excessive loss of water can be counteracted by the presence of well-cutinized and thick-walled epidermis, stomatal encryption and high trichome density. In addition, the higher number of trichomes may be a particularly effective filter, protecting the underlying tissues against deposition on the stomatal pores of particles present in fumarolic steam [51]. Besides, the greater wall thickness and cutinisation of epidermal cells, the higher densities of both stomata and trichomes, the higher value of LDMC and the lower value of SLA in these leaves indicate high investments in leaf “defence”, particularly, structural ones [26]. Some of these features might be an aspect of a common mechanism of stress response in plants, the “stress-induced morphogenic response” that, among other components, comprises changes in cell differentiation status [52,53]; in stress conditions, some epidermal cells are induced to differentiate in stomata or trichomes.
Despite these strategies, physiological parameters showed a stress condition in G leaves.
Pigment content was significantly lower in G than in C leaves, as reported in literature for plants suffering abiotic stress [54]. In accordance, the more stressed G leaves evidenced less and smaller chloroplasts than C leaves, as shown in plants subjected to different environmental stressors [55,56], suggesting a central role of chloroplasts in stress sensing processes. Interestingly both Chla/Chlb and carotenoids/total Chl ratios were not typical of stressed plants [57].
Extreme environmental conditions typical of geothermal fields are known as inducing oxidative stress in plants [4]. In accordance, oxidative stress was recorded in G leaves that showed, in comparison with C leaves, higher levels of hydrogen peroxide and of lipid peroxidation, indicative of membrane damage.
The enhanced ROS generation during abiotic stress may act as an elicitor of common stress response in plants [58] that have developed a complex protective system, including antioxidants and enzymes. G leaves had a good antioxidant capacity in terms of ascorbate and phenols. Glutathione pool was, however, significantly higher in C than in G leaves but higher GSH/GSSG and ASA/DHA ratios, evidenced a higher reducing power in G leaves than in C ones. This is in accordance with literature as many studies underline that a high ratio of GSH/GSSG and/or ASA/DHA may be a crucial element for efficient protection against increased levels of ROS in condition of abiotic stress [59]. A good activity of the main antioxidant enzymes was also detected in G leaves that showed higher APX and CAT activities in comparison with C leaves. APX and CAT were helped in their protective role by GPX and so management of hydrogen peroxide was due to the combined action of these enzymes. In Calluna vulgaris, living in the same geothermal field, catalase seemed to play a minor role in the control of hydrogen peroxide [4] and so, the antioxidant machinery and management of ROS may be significantly different in plants living in the same extreme environment. The activity of other enzymes object of study had similar values in G and C leaves.
In conclusion, leaves of C. salviifolius living in geothermal field showed a combination of peculiar morpho-anatomical, functional and physiological traits. The comparison with leaves developed by the same plants after transferring to controlled laboratory conditions has allowed us to highlight traits putatively involved in plant adaptation. A fine modulation of morphological traits and minor adjustments of antioxidant machinery with a higher reducing power could help C. salviifolius to survive in the restrictive environment of the geothermal alteration field. Based on its ability to live in extreme habitats, C. salviifolius could be a useful model to improve our knowledge on plant adaptability.
Disclosure of interest
The authors declare that they have no conflicts of interest concerning this article.