1 Introduction
Ultraviolet radiation and air pollutants, such as sulfur dioxide (SO2) and ozone (O3) are some of the major factors affecting plant productivity by reduction in photosynthetic pigments, inhibition of physiological processes, alteration in metabolic function, and enzyme activities [1,2]. An increased uptake of SO2 can cause toxicity and reduce plant growth and productivity by interacting with different physiological processes and damaging tissues and pigments [3].
Air pollutants generate reactive oxygen species (ROS). SO2 toxicity is mainly attributed to highly reactive intermediates, such as the sulfur trioxide radical (HSO3–), the superoxide anion radical (O2–), and the hydroxyl radical (OH•), which are generated during the radical-initiated oxidation of SO2 [2,4]. Damaging effects of ROS are minimized by various antioxidant defence systems, including enzymatic and non-enzymatic mechanisms. The antioxidative defence system consists of several enzymes, such as superoxide dismutase (SOD, EC 1.15.1.1), ascorbate peroxidase (APX, EC 1.11.1.11), glutathione reductase (GR, EC 1.6.4.2), and catalase (CAT, EC 1.11.1.6), and low molecular-weight antioxidants, such as ascorbic acid, glutathione, and phenolic compounds [5,6].
Manipulation of the expression of genes coding for antioxidant enzymes in chloroplast has helped to maintain the productivity of plants under stress conditions. A number of studies have reported that overexpression of antioxidant enzymes, such as SOD, APX, and CAT, has increased oxidative stress resistance in transgenic plants [7–9]. As we previously reported, transgenic tobacco plants expressing both SOD and APX in chloroplasts provided strong protection to oxidative stress [10]. Moreover, transgenic potato, sweet potato and tall fescue plants expressing both CuZnSOD and APX under the control of an oxidative stress-inducible SWPA2 promoter in chloroplasts showed resistance against various abiotic stresses, including oxidative stress [11–13]. These results have shown the potential to engineer enhanced oxidative stress in important agronomic crop plants by introducing both CuZnSOD and APX genes into chloroplasts. SOD catalyzes the dismutation of two molecules of O2– in O2 and hydrogen peroxide (H2O2), and APX reduces H2O2 to water by utilizing ascorbate as an electron donor.
Sweet potato [Ipomoea batatas (L.) Lam.] ranks seventh in annual production among the food crops in the world. It is not only a good energy source with carbohydrate content, but is also used for starch and alcohol production. Moreover, it does not require large amounts of fertilizers and other agricultural chemicals, as well as it is known as a relatively drought-resistant crop. However, conventional breeding is limited by its sterility and cross-incompatibility [14]. Molecular breeding is a good tool to overcome such limitations. Previously we have established regeneration and transformation systems in several cultivars of sweet potato via somatic embryogenesis [15,16].
Recently, enhanced abiotic stress tolerance tests with genetic engineering by overexpression of genes in sweet potato plants evidenced that transgenic plants showed tolerance to environmental stress. For example, transgenic sweet potato plants with overexpressing the defence signal pathway or antioxidant enzymes showed improved stress resistance. Transgenic sweet potato plants overexpressing nucleoside diphosphate kinase 2 (NDPK2) showed increased tolerance to methyl viologen (MV)-mediated oxidative stress, salt and low-temperature stress by increased activities of the NDPK and H2O2-scavenging antioxidant enzymes [17]. The transcription factor zinc finger protein (SCOF-1) gene overexpressing transgenic sweet potato also showed enhanced tolerance to low-temperature stress [18]. In addition, the overexpression of the betaine aldehyde dehydrogenase (BADH) gene in sweet potato improved its tolerance to various abiotic stresses, including salt, low-temperature, and oxidative stress. The increased BADH activity and glycine betaines (GB) accumulation as a osmotic protectant in the transgenic plants under normal and multiple environmental stress conditions resulted in increased protection against cellular damage, reduced ROS generation, and increased activities of ROS-scavenging enzymes [19]. However, the enhanced tolerance of transgenic sweet potato plants with genetic engineering to air pollutants, like SO2 has not been characterized in detail as yet.
In our previous reports, transgenic sweet potato plants overexpressing both CuZnSOD and APX under the control of an oxidative stress-inducible SWPA2 promoter in chloroplasts (referred to as SSA plants) had increased protection against chilling and methyl viologen-mediated oxidative stress [12]. In this study, we evaluate the SSA plants for protection against an oxidative stress caused by exposure to 500 ppb of SO2. In addition, changes in the activity of SOD and APX were investigated in SSA transgenic plants exposed to SO2.
2 Materials and methods
2.1 Plant materials
Transgenic sweet potato plants (I. batatas Lam.) expressing the CuZnSOD and APX genes in chloroplast under the control of an oxidative stress-inducible SWPA2 promoter (SSA plants) were generated by particle bombardment. In our previous study [12], the introduced genes in transgenic plants were confirmed by Southern blot analysis. These SSA transgenic plants were cultured on MS [20] medium containing 100 mg/L of kanamycin, 3% sucrose and 0.4% gelrite and maintained at 26 °C under a 16:8 h (light/dark) photoperiod with light supplied at a light intensity of 70 μmol/m2/s by fluorescent tubes. And then, the plants were grown in 10-cm diameter pots containing commercial mineral-mixed soil, in a greenhouse at approximately 28:22 °C (day/night) with daily watering, and 6-week-old plants were used for SO2 treatment. SSA transgenic plants having no differences in morphology compared with non-transgenic (NT) plants were used in this study.
2.2 Exposure to SO2 conditions on whole plants
Plants were fumigated with 500 ppb of SO2 for 8 h/day (between 9 am and 5 pm) in a plant-growth chamber at 26 °C, 60% relative humidity and 16-h photoperiod at 320 μmol/m2/s light from fluorescent tubes. The experimental conditions are described in Lee et al. [21]. The plants were cultured in the chamber for one day prior to SO2 treatment, and chamber SO2 concentrations were monitored just above the crop canopy during treatment using a SO2 analyzer (Model 319, Kimoto, Japan). Non-fumigated control plants were maintained in a separate growth chamber under similar environmental conditions for the same duration as the fumigated plants.
2.3 Determination of PSII photosynthetic efficiency and total chlorophyll content
Chlorophyll fluorescence measurements were performed on the same leaf after SO2 treatment using a portable plant efficiency analyzer (Handy PEA, Hansatech, England). Leaf clips for dark adaptation were placed on the adaxial side of the leaves 30 min before measurement at an excitation irradiance set at 2000 μmol/m2/s. Minimum fluorescence (F0) and maximal fluorescence (Fm) were measured, from which variable fluorescence (Fv) was calculated. Chlorophyll content after SO2 stress was measured by a portable chlorophyll meter (SPAD-502, Konica Minolta, Tokyo, Japan) from intact fully expanded fifth leaves at the top of individual plants.
2.4 RT-PCR analysis
A RT-PCR kit (RevertAid, Fermentas, USA) was used to identify mRNAs coding for CuZnSOD and APX investigated in this study. Reverse transcription of 2-μg aliquots of total RNA was carried out as recommended by the manufacturer. Actin mRNA served as an internal control. Gene-specific primers for PCR were designed according to cDNAs. The APX primer set (5′-ATGGGAAAATCTTACCCAACTGTTA-3′, 5′-TTAGGCTTCAGCAAATCCAAGCTC-3′) amplifies a 753-bp product; the CuZnSOD primer pair (5′-ATGGTGAAGGCTGAAGCTGTTCTT-3′, 5′-CTATCCTCGCAAACCAATACCG-3′) generates a 459-bp product [12].
2.5 Determination of total protein contents and SOD and APX enzyme activities
The leaves of sweet potato were homogenized on ice with a mortar in a 0.1 M potassium phosphate buffer (pH 7.0). Protein concentration was determined according to the Bradford [22] method using Bio-Rad protein assay reagent. SOD activity was measured according to the method of McCord and Fridovich [23] using xanthine, xanthine oxidase, and cytochrome c. One unit of SOD was defined as the amount of enzyme that inhibits the rate of ferric-cytochrome c reduction by 50%. APX activity was assayed according to the method described by Nakano and Asada [24] using ascorbic acid as a substrate. The oxidation of ascorbate was initiated by H2O2, and the decrease at 290 nm for 1.5 min was monitored. One unit of APX was defined as the amount of enzyme oxidizing 1 μM of ascorbate.
2.6 Native poly-acrylamide gel electrophoresis (PAGE)
Native PAGE of SOD and POX were performed on a 7.5% gel at 120 V at 4 °C [25]. For SOD activity staining, the gel was incubated in the dark for 30 min in a staining buffer [50 mM potassium phosphate buffer, pH 7.8, 0.026 mM riboflavin, 0.25 mM nitro blue tetrazolium (NBT), 0.2% TEMED] and then exposed to a light box until the SOD activity bands became visible. The SOD isozymes were differentiated by incubating the gel for 20 min in 50 mM potassium phosphate buffer (pH 7.8) containing either 3 mM KCN or 5 mM H2O2 before staining for activity. CuZnSODs were inhibited by KCN and H2O2. FeSODs were resistant to KCN, but were inactivated by H2O2. MnSODs were resistant to both inhibitors [25]. APX isozymes were detected by equilibrating the gels for 30 min in a 50 mM sodium phosphate/2 mM ascorbate buffer solution (pH 7.0), and then in a 50 mM sodium phosphate/4 mM ascorbate/2 mM H2O2 buffer solution (pH 7.0) for 20 min. After washing with a 50 mM sodium phosphate buffer solution (pH 7.0) for 1 min, the gels were submerged in a 50 mM sodium phosphate/28 mM TEMED/1.25 mM NBT buffer solution (pH 7.8) for 10 min at room temperature [26]. Native PAGE band intensity was quantified by Bio-1d software program (Viber Lourmat, Germany).
2.7 Statistical analyses
Data were analyzed by one-way analysis of variance (ANOVA). The subsequent multiple comparisons were examined based on the least significant difference (LSD) and Duncan's multiple range test. All statistical analyses were performed using the Statistical Package for the Social Sciences (SPSS 12), and statistical significance was set at P < 0.05 and P < 0.01.
3 Results
3.1 Enhanced tolerance of SSA transgenic plants during exposure to SO2
To evaluate SO2-induced oxidative stress tolerance in the whole plant level, 6-week-old NT and SSA transgenic plants were exposed to 500 ppb of SO2 for 8 h per day for 5 days. SO2 stress caused visible damage in the leaves of both NT plants and SSA plants, but younger leaves were non-symptomatic in all lines. However, the damage in the leaves of non-transgenic (NT) plants was more severe than in those of SSA transgenic plants (Fig. 1A). Five days of SO2 stress led to a significant difference between the SSA transgenic and NT plants in photosynthetic efficiency (Fv/Fm). The Fv/Fm activity of NT plants severely decreased by 46% on the 5th day after the treatment, whereas the activity of SSA plants remained at the high level of 93% under the same conditions (Fig. 1B). Moreover, to investigate the recovery of Fv/Fm activity, NT and SSA plants exposed to SO2 were grown in the growth chamber under normal conditions (25 °C, 350 μmol/m2/s). After 5 days of recovery, the Fv/Fm activity decreased by 63% in the NT plants, but remained unaltered in SSA plants (Fig. 1B). There were no differences in Fv/Fm activity between untreated NT plants and SSA plants (data not shown). These results suggested that photosynthesis of SSA plants was less affected during SO2 treatments and in recovery conditions than that of NT plants.

(Color online.) Enhanced SO2 tolerance in SSA transgenic sweet potato plants. A. Visible damage in the leaves of NT and SSA sweet potato plants exposed to 500 ppb of SO2 for 8 h per day for 5 days. NT: non-transgenic plants; SSA: transgenic plants expressing both of CuZnSOD and APX in chloroplasts under the control of an oxidative stress-inducible SWPA2 promoter. B. Changes in photosynthetic efficiency (Fv/Fm) in leaves of NT and SSA sweet potato plants after five days of SO2 treatment. Fv/Fm values in the third leaf from the top of both plants were measured after dark adaptation for 30 min. Data are means ± SE of three independent measurements. Masquer
(Color online.) Enhanced SO2 tolerance in SSA transgenic sweet potato plants. A. Visible damage in the leaves of NT and SSA sweet potato plants exposed to 500 ppb of SO2 for 8 h per day for 5 days. NT: non-transgenic plants; SSA: transgenic ... Lire la suite
To investigate the effect of SO2 in leaves of different ages, the plants were exposed to 500 ppb of SO2 for 5 days. In NT plants, the Fv/Fm activity decreased to 40, 53, 53, 60 and 88% of that of control NT plants, counting from the oldest leaf after the treatment. The most serious damage in leaves exposed to SO2 was in the oldest leaf. In contrast, the activity of SSA plants varied little according to the age of the leaves (Fig. 2A). The chlorophyll content was also evaluated in the central portion of leaves with different ages from plants exposed to SO2. The chlorophyll content decreased by 78, 61, 72, 47 and 31%, counting from the oldest one after the treatment in NT plants, whereas that of SSA plants remained high, 97%, in the third leaves under the same conditions (Fig. 2B). It is clear that SSA plants showed enhanced tolerance to oxidative stress generated by SO2 in these conditions.

Effect of SO2 on photosynthetic efficiency (Fv/Fm) and total chlorophyll in the SSA transgenic sweet potato plants. A. Photosynthetic efficiency, and (B) total chlorophyll content in different leaf ages of NT and SSA sweet potato plants exposed to 500 ppb of SO2 for 5 days. The samples were collected after 5 days of SO2 treatment. The first leaf (1) is the oldest leaf and 5 is the new one. Total chlorophyll contents means units in the 6-mm leaf area. Data are means ± SE of three independent measurements. Masquer
Effect of SO2 on photosynthetic efficiency (Fv/Fm) and total chlorophyll in the SSA transgenic sweet potato plants. A. Photosynthetic efficiency, and (B) total chlorophyll content in different leaf ages of NT and SSA sweet potato plants exposed to 500 ppb of ... Lire la suite
3.2 Changes in expression and activity of SOD and APX after exposure to SO2
To confirm the expression of the introduced CuZnSOD and APX genes in SSA plants under SO2 treatment, we investigated the accumulation of CuZnSOD and APX mRNAs after the plants were exposed to 500 ppb of SO2 for 8 h per day for 5 days. The CuZnSOD and APX genes were detected in SSA plants, but not in NT plants under SO2 stress conditions (Fig. 3).

RT-PCR analysis of the expression of introduced CuZnSOD and APX genes in leaves of NT and SSA sweet potato plants exposed to 500 ppb of SO2. Total RNA was extracted from leaves 5 days after exposure to SO2. Actin was used as a control for equal loading.
To determine the contribution of the antioxidant enzyme level to the protection against SO2-induced oxidative stress, we compared the activities of SOD and APX enzymes between NT and SSA plants. Fig. 4 shows the activities of SOD and APX in sweet potato plants exposed to 500 ppb of SO2 for 8 h per day for five days. Specific SOD activity in NT plants increased after SO2 stress treatment, whereas that in SSA plants did not show any increase under the same conditions. There was no significant difference in the SOD activity level between NT and SSA plants under these stress conditions (Fig. 4A). In the case of APX, activity showed little difference between the NT and SSA plants under no stress conditions. However, the specific APX activity in NT plants increased three times under stress condition, while that of SSA plants dramatically increased five times compared to the case with no stress conditions. In addition, the specific APX activity of SSA plants was about two times higher than that of NT plants after both had been exposed to SO2 for 5 days. Isoenzyme patterns of SOD and APX in native gel assay were similar to the changes in these enzymes’ activities shown in Fig. 4A (Fig. 4B). In sweet potato plants, the CuZnSOD and MnSOD isoforms were detected in normal and stress conditions. However, the FeSOD isoform was not detected in any conditions. These results suggest that CuZnSOD and APX gene expression under SO2 stress contributed more in SSA plants.
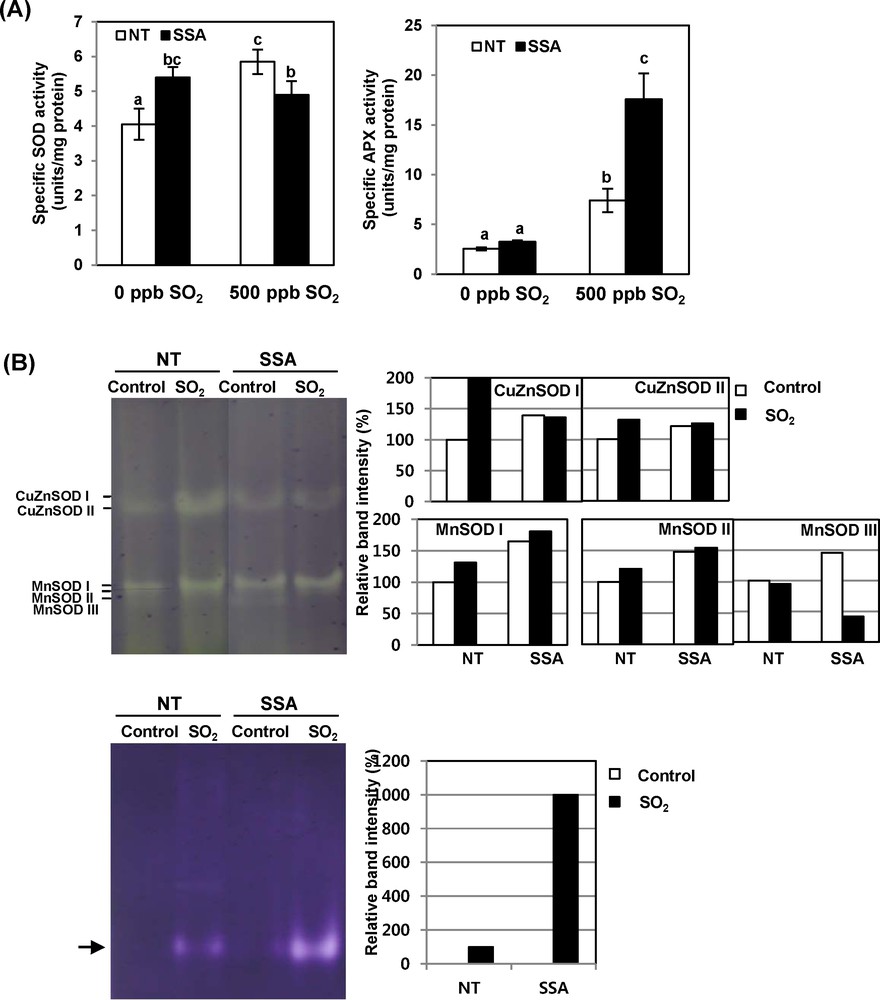
(Color online.) Enzyme activity of SOD and APX in NT and SSA sweet potato plants exposed to 500 ppb of SO2 for 5 days. A. Changes in enzyme activities of SOD and APX in sweet potato plants during exposure to SO2. Data are means ± SE of three replicates. B. Native gel analysis of SOD and APX isozymes during exposure to SO2. SOD activity was detected with a negative staining solution using NBT after electrophoresis. Seventy micrograms of total protein concentrated from leaves were loaded in each line. APX activity was detected with a negative staining solution using NBT after electrophoresis. Eighty micrograms of total protein concentrated from leaves were loaded in each line. Masquer
(Color online.) Enzyme activity of SOD and APX in NT and SSA sweet potato plants exposed to 500 ppb of SO2 for 5 days. A. Changes in enzyme activities of SOD and APX in sweet potato plants during exposure to ... Lire la suite
4 Discussion
We had previously successfully developed transgenic sweet potato plants expressing both CuZnSOD and APX in chloroplasts under the control of an oxidative stress-inducible SWPA2 promoter (SSA plants) and characterized under MV-mediated oxidative stress and chilling conditions [12]. In this study, expressions of CuZnSOD and APX in sweet potato caused the activation of ROS detoxification under exposure to SO2, thereby conferring increased tolerance to SO2-induced oxidative stress.
It is known that ROS are implicated in a variety of environmental stresses in plants and the chloroplast appears to be a main location affected by the conditions of stress in plant cells. The protection of plants against ROS is achieved by partial suppression of ROS production and scavenging of previously produced ROS. The experiments using transgenic plants expressing foreign genes for antioxidant enzymes have shown that a variety of genes are used in several plant species [27–30]. In particular, direct modification of the expression of SOD or APX in chloroplasts has been assigned a key role in the protection of plants against various oxidative stresses [7,9]. Kwon et al. [10] previously reported that transgenic tobacco plants expressing both CuZnSOD and APX in chloroplasts under the control of CaMV 35S promoter provided strong protection against MV-mediated oxidative stress. Transgenic tobacco benefited from a synergistic effect of expression of the two enzymes with regard to protection against oxidative stresses. It appears that the simultaneous scavenging by CuZnSOD of O2– and H2O2, which can otherwise deactivate CuZnSOD and APX, is important for the maintenance of plant productivity under harsh conditions. Recently, we demonstrated that transgenic potato, sweet potato plants expressing both CuZnSOD and APX under the control of an oxidative stress-inducible SWPA2 promoter in chloroplasts showed enhanced tolerance against MV-mediated oxidative stress and temperature stress [11,12]. Lee et al. [13] also reported that SSA transgenic tall fescue plants exhibited enhanced tolerance against MV, H2O2, Cd, Cu as treatment conditions. SO2 produces many free radicals such as O2–, OH–, and H2O2 when it is converted from HSO3– or SO32– into SO42– in plants [31]. Madamanchi et al. [32] suggested that SO2-tolerant species or strains have more efficient antioxidant systems than sensitive ones. As expected, in this study, SSA transgenic sweet potato plants showed a lower decrease in photosynthetic efficiency and chlorophyll content than NT plants under SO2-induced oxidative stress (Figs. 1 and 2). It seems likely that a high expression of the introduced CuZnSOD and APX genes may result in the maintenance of the photosynthetic capacity under SO2-induced oxidative stress conditions.
It is known that a powerful expression system with an appropriate promoter is an important requisite for efficient expression system in plant cells, such as a stress-inducible promoter [33]. Thus, the development of stress-inducible promoters that control precisely the expression of target defence genes under particular stress conditions is very important for developing transgenic plants with enhanced tolerance to various stresses. We have previously isolated an oxidative stress-inducible SWPA2 promoter from cell cultures of sweet potato and characterized its function in transgenic tobacco plants in terms of environmental stresses, including oxidative stress [34]. In addition, the swpa2 genes from sweet potato cell culture were strongly induced following SO2, O3, and UV treatments. [35]. In this study, transgenic sweet potato overexpressing both CuZnSOD and APX under the control of SWPA2 promoter showed enhanced tolerance to SO2-induced oxidative stress (Figs. 1 and 2). These results suggest that the SWPA2 promoter could be very useful for the development of transgenic plants with enhanced tolerance to multiple environmental stresses, including air pollutants.
In this study, the SOD and APX activity of the SSA plants exhibited slightly higher levels than that of NT plant under normal conditions (Fig. 4). Thus, SWPA2 promoter should be expressed in conditions with and without SO2 treatment. Actually, CuZnSOD band showed a similar pattern in SSA plants in both conditions. However, NT plants exhibited a SO2-induced increase in SOD activity. Therefore, we suggested that non-fumigated control conditions in the growth chamber might affect sweet potato plants for induction by the SWPA2 promoter. Thus, SOD expression and activity in SSA plants maintained higher levels than NT plants under normal growth chamber conditions, but SO2-induced SOD activity showed a higher level in NT plants than in SSA plants. When considering the APX activity in SSA plants during SO2 treatments, the difference between SSA and NT became even less remarkable in conditions without treatment (Fig. 4). It is possible that the SO2 resistance phenotype in the SSA plants may be a consequence of increased APX activity by SO2-induced oxidative stress.
In conclusion, transgenic sweet potato plants overexpressing both CuZnSOD and APX in the chloroplast under the control of a stress-inducible SWPA2 promoter possess enhanced tolerance to environmental stress, including MV-mediated oxidative stress and chilling stress as well as to an air pollution stress such as that induced by SO2. Further characterization of the SSA plants is under investigation in field conditions for the yields of tuber. In addition, SSA plants may be useful for breeding materials with new characteristics as well as for sustainable growth on marginal lands.
Acknowledgements
This work was supported by the research group promotion program (2014-03-015) of Gyeongsang National University, grants from the Next-Generation BioGreen 21 Program (SSAC, grants: PJ01106401), Rural Development Administration, Korea, Cooperative Research Program for Agriculture Science & Technology Development (Project title: National Agricultural Genome Program, Project No. PJ01033902) and the KRIBB Initiate Program.