1 Introduction
Argania spinosa L. (family Sapotaceae) is a Moroccan endemic tree; it covers there more than 800 000 km, alleviating significantly the poverty of the population nearby the argan forest [1]. This multipurpose tree is increasingly required due to its ecological interest and its socio-economic value [2–4]. The argan tree value is due more particularly to the much-appreciated oil extracted from its kernels, which gives a much-appreciated oil with medicinal and cosmetic properties [5] reflected by its high market prices and an increasing export demand [6,7]. However, despite the oil's value, the argan natural populations are threatened by destructive logging, overgrazing and climate changes [8–10]. These stressful conditions led to forest decline, causing its protection by UNESCO in 1998. Moreover, as the set up of new orchards dedicated to oil production is not so far a successful maneuver [11–13], there is an urge for further alleviation of the pressure exerted on the argan natural resources through the development of appropriate technologies that could fill the demand supply gap. The biotechnological approach offers an opportunity to use any plant tissue by growing it in vitro without causing unbalances to the natural ecosystem [14,15]. Plant tissue culture technology could be used either for propagation or to provide a cost-effective, sustainable, and environment-friendly platform for the commercial exploitation of the natural products from plants that are difficult to cultivate or endangered by overexploitation [14–17]. Along with the sustainability, plant tissue culture allows raw material continuous production independently of the season and of the environmental influences [17]. Callus induction from somatic tissues, in particular, is a widely used technology either for basic research or for industrial applications [14,15,17–20]. The antioxidant power in plant tissues is an intensely studied point covering both compounds and enzymes, which correlate positively, due to their free radical scavenging properties [21–23]. Numerous environmental stimuli, such as UV radiation and chemical pollutants, generate excessive reactive oxygen species (ROS) in the human body and skin cells, most commonly the superoxide anion radical (O2•−), the hydroxyl radical (HO•), and hydrogen peroxide (H2O2) [24]. This species produces severe damage to the cellular system integrity, including that of DNA, leading consequently to premature skin disorders, aging and cell senescence [25], which promoted research for antioxidants that could potentially reverse these changes [25,26]. Moreover, as the antioxidants bioavailability and production from the differentiated tissues was reported to be restricted by several technical bottlenecks and limiting issues [25], the dedifferentiated cells’ approach is characterized by an open chromatin state, of the same genome, making it flexible for dynamic changes in gene expression [27–29]. The well-coordinated cell-to-cell communication networking is another important feature of the shoot and apical meristems dedifferentiated cells [30–32]. Thus, the plant-dedifferentiated stem cells in culture, with their flexible and easy to manipulate gene expression, through the supplementation of stimulating factors enable reach higher antioxidant yield in manufacturing plant active ingredients [33]. The first challenging step in the cost-effectiveness of callus development process is the selection of the proper explant to cultivate and the successful sterilization way that may prevent important economical losses due to time, effort, and material waste [34,46]. Thus, the axenic culture establishment has to be standardized to achieve better survival without tissue damages [35]. In addition, the optimal growth regulators and physical conditions could influence also the process [36]. Thus, the present study aims to develop an efficient cost-effective procedure for the establishment of A. spinosa axenic callus and for promoting the antioxidant status through an abiotic and safe eliciting factor as an approach toward the sustainable production of bioactive products from the economically important argan tree.
2 Materials and methods
2.1 Plant material
The mother plant source of material was clonally propagated in the nursery through cutting from at least 100-year-old superior trees selected in the Agadir argan forest. The clonal propagation has been conducted by the Regional Center for Water and Forests of Marrakech [12,13]. Shoots about 10 to 20 cm in length with dormant axillary buds were collected from one about 6- to 7-year-old tree to discard any genotype impact. The shoots were washed with tap water supplemented by a few drops of commercial detergent and treated by 4% (w/v) methyl-thiophanate (Pelt 44) for about 10 min.
2.2 Culture media
After surface disinfection, vegetative explants that consisted of buds were excised and transferred into Petri dishes containing a callus-inducing medium that consists of a Murashige and Skoog (MS) medium [37] with half-strength MS salts supplemented with a MS vitamins (0.1%) mixture, the PGRs, 100 mg L−1 of myo-inositol, 30 g L−1 of sucrose, and 8 g L−1 of agar. The pH of the medium was adjusted to 5.8 with 1 M NaOH and HCl prior to autoclaving at 121 °C for 20 min. All cultures were stored in the dark at 25 ± 2 °C.
2.3 Standardization of the sterilization protocol
The procedure of sterilization had been divided into two phases: the outside laminar airflow phase and the inside air flow step. The shoots were firstly rinsed with tap water supplemented by a few drops of commercial detergent and followed by a treatment with methyl-thiophanate (Pelt 44) within 15 min. After three rinses with sterile deionized water, the explants were transferred to the Laminar Air Flow. In order to control contaminations, four sterilizing agents, namely, mercuric chloride (HgCl2), sodium hypochlorite (NaOCl) and H2O2, calcium hypochlorite (Ca(ClO)2), were tested for surface sterilization by varying their concentration and time of exposure. Records for contaminations, browning and non-growing cultures were taken after four weeks of incubation under dark conditions at 25 ± 2 °C.
2.4 Effect of the exogenic hormonal stimuli on callus formation
The effect of PGRs under different concentrations of 2,4-dichlorophenoxyacetic acid (2,4-D) at 0, 0.01, 0.2, 0.4, 0.5, 1, and 2 mg L−1 with naphthaleneacetic acid (NAA; at 0, 0.01, 0.2, 0.4, 1, and 2 mg L−1) and 6-benzylaminopurine (BAP; at 0, 0.01, 0.2, 0.4 and 1 mg L−1), in the following combinations: 2,4D-ANA; 2,4D-BAP and 2,4D-BAP (Table 1), was investigated in order to study their effect on callus induction. The number of developed callus was scored after four weeks of culture under dark conditions at 25 ± 2 °C. Each treatment was conducted in ten replicates and each experiment was repeated four times.
Hormonal combinations tested on callus induction in A. spinosa.
Treatment | 2,4D (mg/l) | ANA (mg/l) | BAP (mg/l) |
T1 | 0.01 | – | 0 |
T2 | 1 | – | 0 |
T3 | 1 | – | 1 |
T4 | 0.01 | – | 0.01 |
T5 | 0.5 | – | 1 |
T6 | 1 | – | 0.5 |
T7 | 0.01 | – | 1 |
T8 | 1 | – | 0.01 |
T9 | 0.4 | – | 0.4 |
T10 | 0.4 | – | 0.2 |
T11 | 0.2 | – | 0.2 |
T12 | 0.2 | – | 0.4 |
T13 | 2 | 1 | – |
T14 | 1 | 2 | – |
T15 | 1 | 1 | – |
T16 | 0.2 | 0.2 | – |
T17 | 0.4 | 0.4 | – |
T18 | 0.4 | 0.2 | – |
T19 | – | 1 | 1 |
T20 | – | 0.01 | 0.01 |
2.5 Callogenesis potential of different vegetative explants of A. spinosa
Several types of explants were tested to determine their callogenesis potential. Apical and axillary buds, leaves and stems were transferred on solid MS media supplemented with, the optimum hormone combination, 1.0 mg L−1 NAA and 1.0 mg L−1 2,4D at pH 5.8. Cultures were maintained at 25 ± 2 °C in the dark. After four weeks of culture, the percentage of callogenesis was recorded for each type of explant. Fifteen replicates at least were used for each treatment, and each treatment was repeated thrice.
2.6 Effect of UV radiation and pH on callus development
In this study, only the factor being studied was varied. The effect of the light/dark regime on the callus induction rates of the axillary buds was investigated. The media were supplemented with 1.0 mg L−1 NAA and 1.0 mg L−1 2,4D under pH of 5.8. Cultures were incubated under continuous dark, 16:8 h photoperiod, or under continuous cool-white fluorescent light (400 μmol m−2 s−1, which is equivalent to 86.96 W m−2 UV irradiation), in order to test the effect of the UV radiation on callus initiation and growth. The results were observed after four weeks of incubation at 25 ± 2 °C. At least 30 replicates were used for each treatment and every treatment was repeated thrice.
Similarly, the explants, consisting of axillary buds, were transferred onto media supplemented with the optimum hormonal requirement with different pH values (5.5, 5.6, 5.7, 5.8, 5.9, and 6). Cultures were maintained for four weeks in the dark at 25 ± 2 °C. At least 15 replicates were used for each treatment and each treatment was repeated thrice.
2.7 Effect of salt stress on A. spinosa callus proliferation
The growth of calli derived from buds under different levels of salt stress was tested in order to examine their growth performance and to determine the optimal concentration to use as a pressure without adverse effect on cells survival. One-month-old calli with a known initial weight were exposed to increasing NaCl concentrations (0, 2.5, 3, 4, 5, 7.5, 10, 15, 20 g L−1) equivalent to 0, 43, 51, 68, 85, 128, 171, 256, 342 mM, respectively. After eight weeks of incubation, their final weight was measured again. The difference in the fresh weight (FW) of the calli was recorded for each treatment. Ten replications were used per treatment, with one callus per replication.
2.8 Abiotic elicitation of A. spinosa cells
1-month-old calli were transferred to a medium containing 80 mM NaCl over three months and were subsequently subjected to antioxidative tests comparatively to non-stressed calli.
2.9 Evaluation of the antioxidant status of the callus
The antioxidative behavior of the stressed (S) and non-stressed calli (NS) was measured under (+NaCl) and without stress (–NaCl). The antioxidative potential was triggered by 80 mM NaCl for one week. Four situations were then studied: stressed calli under salt stress (S + NaCl), stressed calli under normal conditions (S–NaCl), non-stressed calli under stress (NS + NaCl), and non-stressed calli under normal conditions (NS–NaCl). H2O2 content, malondialdehyde (MDA) concentrations, PO, SOD, CAT and polyphenol oxidase (PPO) activities were undertaken.
2.9.1 H2O2 concentrations
H2O2 concentrations were determined according to Velikova et al. [38]. A fresh callus (0.1 g) was homogenized in an ice bath with 3 ml of 0.1% (w/v) trichloroacetic acid (TCA). The homogenate was centrifuged at 12,000 g for 15 min at 4 °C, and the supernatant was collected and used as the crude enzyme extract. Each 100-μl sample of extract was mixed with 300 μl of 20 mM guaicol, 2 ml of 100 mM phosphate buffer, pH 7.0, and 1 ml of 1.0 M potassium iodide. The change in absorbance at 390 nm was recorded after 1 h of incubation in the dark using a UV/Visible spectrophotometer (6305; Jenway, London, UK). H2O2 concentrations are presented in μmol H2O2 g−1 FW of callus, making reference to a series of H2O2 standards prepared under the same conditions. Measurements were made on six replicates per treatment.
2.9.2 Malondialdehyde (MDA) concentrations
Lipid peroxidation was measured in terms of MDA concentrations, estimated as thiobarbituric acid-reactive substances (TBA) [39]. Each sample of callus (100 mg) was homogenized in 2.0 ml of 0.1% (w/v) TCA. The homogenate was centrifuged at 15,000 g for 15 min, and 1.0 ml of the supernatant was added to 1.0 ml of 20% (w/v) TCA containing 0.5% (w/v) of TBA. The mixture was heated at 95 °C for 30 min, then cooled on ice. After centrifugation at 10,000 g for 10 min, the absorbance of the supernatant was measured at 532 nm using a Jenway 6305 UV/Visible spectrophotometer. MDA concentrations were calculated using a molar extinction coefficient of 155 mM−1 cm−1. Six replicate callus samples were used for each treatment. The results were expressed in mol MDA g−1 FW.
2.9.3 POD, SOD, and PPO activities
Forty milligrams of calli (n = 6 samples with three replicates per treatment) were homogenized in 2.0 ml of 0.1 M potassium phosphate buffer pH 7.8 containing 5% (w/v) polyvinyl polypyrrolidone (PVPP) and 0.2% (v/v) Triton X-100. The homogenate was centrifuged at 12,000 g for 30 min at 4 °C, and the supernatant was used as a crude enzyme extract to measure the activities of POD, SOD, and polyphenol oxidase [PPO] [40].
POD activity was assayed following the method of Rodriguez and Sanchez [41]. Each 100-μl sample of crude extract was mixed with 300 μl of 20 mM guaiacol and 2.0 ml of 0.1 M potassium phosphate buffer, pH 6.0. The reaction was started by the addition of 200 μl of 0.3% (v/v) H2O2, and the formation of tetraguaicol was followed by measuring the absorbance at 470 nm using a Jenway 6305 UV/Visible spectrophotometer.
To measure PPO activity, each 1.1-ml reaction mixture contained 500 μl of 1.6% (w/v) catechol in 0.1 M phosphate buffer (pH 6.0), 250 μl of distilled water, 200 μl of 0.1 M phosphate buffer (pH 6.0), and 100 μl of crude callus extract [42]. The absorbance was recorded at 410 nm using a spectrophotometer.
The specific enzyme activities of POD and PPO were expressed in μmol of guaiacol or μmol of catechol mg−1 total soluble protein (TSP) min−1 (see below), using extinction coefficients of 26.6 mM−1 cm−1, and 22.6 mM−1·cm−1 for guaiacol and catechol, respectively, with six replicates per treatment.
The total SOD activity was assayed according to Beauchamp and Fridovich [43], based on the ability of SOD to inhibit the reduction of nitroblue tetrazolium chloride (NBT) by following the change in absorbance at 560 nm in a spectrophotometer. Each 2.0-ml reaction mixture consisted of 50 μl of 50 mM phosphate buffer, pH 7.8 containing 0.1 mM ethylenediaminetetraacetic acid (EDTA), 25 μl of 33 μM NBT, 50 μl of 10 mML-methionine, 25 μl of 4 μM riboflavin, and 50 μl of enzyme extract. The riboflavin was added last, and the test tubes were shaken and placed under fluorescent lamps at a light intensity of 50 mmol photons m−2 s−1. Identical solutions were kept in the dark and served as blanks. One unit of SOD activity was defined as the amount of enzyme that inhibited 50% of the reduction of NBT under the assay conditions. The experiment was conducted with six replicates per treatment. The specific SOD activity was expressed in units SOD mg−1 TSP min−1.
2.9.4 CAT activity
CAT activity was assayed by the method of Gong et al. [44]. A fresh callus (100 mg) was homogenized in 3.0 ml of 100 mM Tris-HCl buffer, pH 8.5, containing 2 mM EDTA and 5% (w/v) PVPP. The homogenate was centrifuged at 16,000 g for 14 min at 4 °C and the supernatant was used to measure CAT activity.
CAT activity was measured by following the loss of H2O2. Each sample of crude extract (250 μl) was added to 2.0 ml of 50 mM Tris-HCl buffer, pH 6.8, containing 5 mM H2O2. The reaction was stopped after 10 min by adding 0.25 ml of 20% (v/v) titanium tetrachloric acid (TiCl4) at 20 °C. A blank was prepared by adding 0.25 ml of 20% (v/v) TiCl4 at the start, to stop all CAT activity. CAT activity was determined by comparing the absorbance at 415 nm against a standard curve of H2O2. Six replicates were used for each treatment. One replicate represented one callus. CAT activity was expressed in μmol H2O2 mg−1 TSP min−1.
2.9.5 Protein analysis
Total soluble protein (TSP) concentrations in each crude callus extract were determined according to Bradford [45] using bovine serum albumin (BSA) as the standard. Values were used to express the specific activities of the various anti-oxidant enzymes.
2.10 Statistical analysis
Data were analyzed by the analysis of variance (ANOVA) using SPSS software (Version 10.0; Norusis 1986). Means were compared using the Tukey's Post hoc test. Statistical analyses were considered significant at P ≤ 0.05.
3 Results
3.1 Standardization of the sterilization protocol
After observing the explants for their growth and contaminations, our results indicated that the use of mercuric chloride (HgCl2) insures 100% of decontamination with adverse effect on the explant survival, even when used at low doses. HgCl2 seems to be phytotoxic for the A. spinosa tissues (Fig. 1). However, among the other sterilizing agents tested, the sodium hypochlorite provided the best results with maximum healthy shoots and good survival rates. Nonetheless, at high concentrations, above 6% during 20 min, we noted an undesirable effect on the quality of the explants. Hydrogen peroxide and calcium hypochlorite were inefficient as they led to incoherent results relatively to sodium hypochlorite (Fig. 1). Therefore, for surface disinfection, the sodium hypochlorite was elected as the best disinfectant agent of explants (Fig. 1). Thus, the disinfection protocol consists in soaking the explants for 20 min in a solution of sodium hypochlorite at 6% (v/v) supplemented by 0.1 mL of Tween 80 with continuous shaking. The explants were rinsed subsequently three times with sterile deionized water.

Effect of different sterilization agents under different concentrations and varying times of exposure on the percentage of infected plants of A. spinosa explants cultured on an MS medium supplemented with 1.0 mg L−1 of ANA and 1.0 mg L−1 of 2,4D. Values (means ± SD) with distinct letters are significantly different at 5% (Tukey's test). Fifteen explants at least were tested per experiment, and each experiment was repeated thrice.
3.2 Effect of the hormonal stimuli on callus formation
The influence of growth regulators on callus development was investigated using different combinations of auxins and cytokinins (Table 1). Callus initiation of A. spinosa occurred within 20 days. The callogenic response under different hormonal balances varied from 10 to 95% (Fig. 2). The maximum response (95%) was achieved by the simultaneous addition of 1.0 mg L−1 NAA and 1.0 mg L−1 2,4D. The synergetic combination 2,4D and BAP, at 0.01 and 1.0 mg L−1, respectively, was also favorable to promote callus induction. The use of both combinations is efficient for promoting callus formation. Therefore, for further experiments, we selected randomly between the two hormonal combinations. The lowest amount of callus induction (10%) was obtained under high BAP concentration combined with low auxin levels (Fig. 2). 2,4D and NAA seem to be the most effective hormones for callus induction of A. spinosa from vegetative explants.
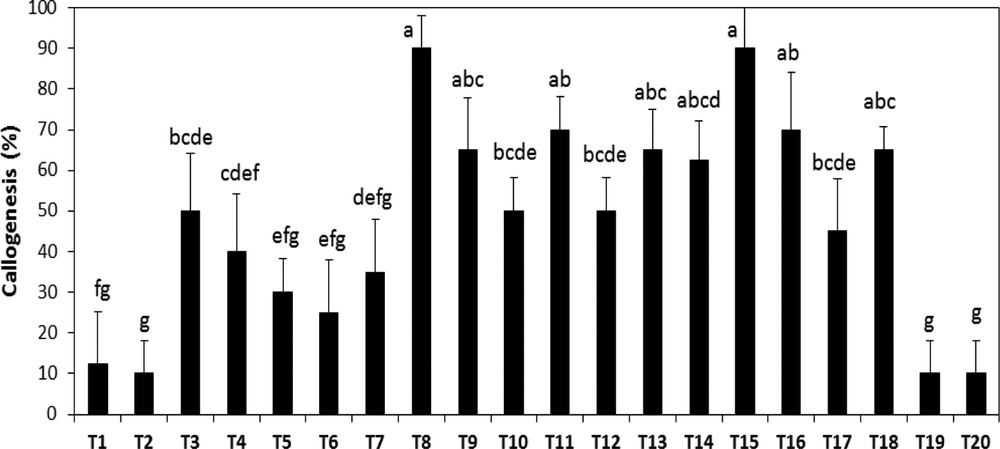
Effect of different hormonal combinations on callus formation from bud explants of A. spinosa. Results were scored after four weeks of culture. Values (means ± SD) with distinct letters are significantly different at 5% (Tukey's test). Each treatment was conducted in at least ten replicates, and each experiment was repeated four times. (T: combination treatment).
3.3 Callogenesis potential of different explants of A. spinosa
The study shows that the induction of callus could be obtained from several types of explants. A mass of friable and yellowish callus was rapidly produced for all the types of explants tested. However, the different explants showed variable growth responses of the callus. For leaves, callus formation has begun from the midrib and slowly developed throughout all the leave (Fig. 3). However, for meristems, callus formation started by an increase in the volume of the explants before proliferation of the undifferentiated cells. In fact, the explant type had a significant effect on the success of the callogenesis of A. spinosa. Callus induction rates varied depending on the type of explant. Among the different types of explants tested, the axillary buds were the most responsive, with the highest callus induction potential (Fig. 4).

Morphology of one-month-old callus of A. spinosa raised from steam (a), nodes (b) and leaves (c) cultured on Murashige and Skoog medium supplemented with 1.0 mg L−1 of NAA and 1.0 mg L−1 of 2,4D.
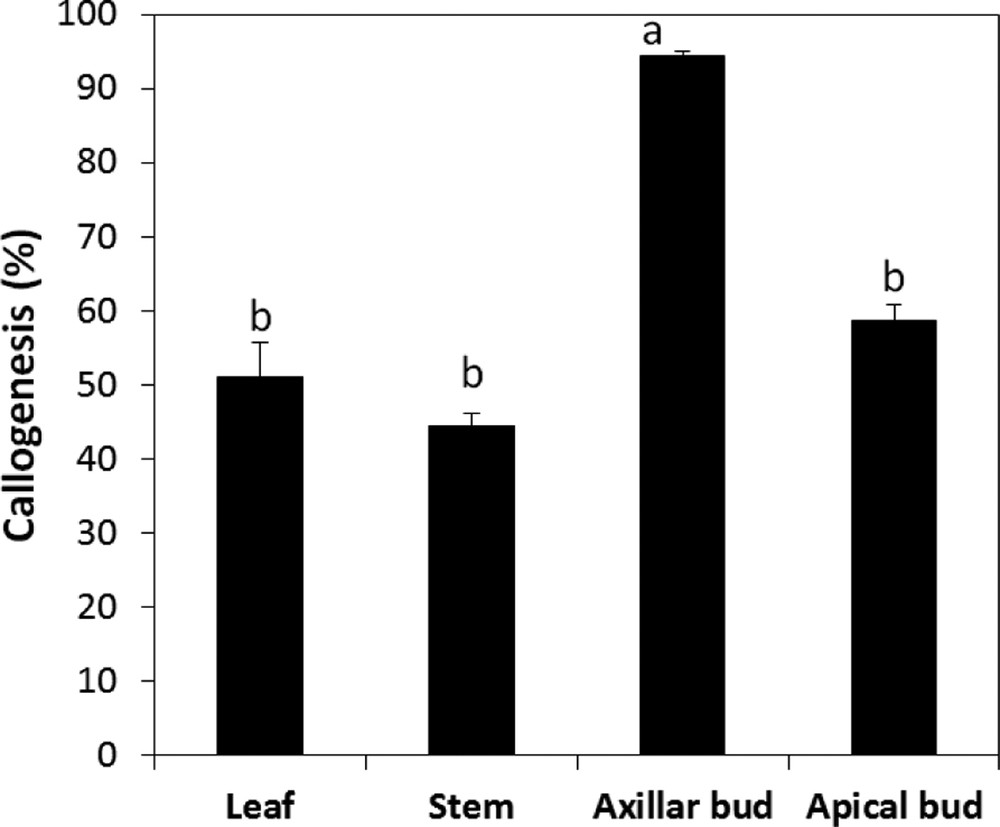
Callogenesis rate of different type of explants of A. spinosa cultured in an MS medium supplemented with 1.0 mg L−1 of NAA and 1.0 mg L−1 of 2,4D. The results were scored after four weeks of culture. Values (means ± SD) with distinct letters are significantly different at 5% (Tukey's test). Each experiment consisted of at least 13 explants and was repeated thrice.
3.4 Effect of UV radiation and pH on callus development
According to the results obtained, we noticed that callus initiation of A. spinosa is very sensitive to the changes in the incubation conditions. It was observed that callus induction occurred earlier and with the best rates under dark when compared to a 16:8-h photoperiod and continuous light regimes (Fig. 5). Therefore, the light has been found to severely hamper callus development and induce browning. Our results also revealed that the callogenic response of A. spinosa explants has been affected by the pH of the medium. Indeed, we recorded significant differences (P ≤ 0.05) in callogenesis rates as a function of pH; for instance, the maximum growth of calli was observed at pH 5.8 (Fig. 6).

Callogenesis rate of A. spinosa axillary buds cultured in an MS medium supplemented with 1.0 mg L−1 of NAA and 1.0 mg L−1 of 2,4D under different light/dark regimes. The results were scored after four weeks of culture. Values (means ± SD) with distinct letters are significantly different at 5% (Tukey's test). Each experiment consisted of at least 13 explants and was repeated thrice.

Callogenesis rate of A. spinosa axillary buds cultured in an MS medium supplemented with 1.0 mg L−1 of NAA and 1.0 mg L−1 of 2,4D under different pH conditions. The results were scored after four weeks of culture. Values (means ± SD) with distinct letters are significantly different at 5% (Tukey's test). Each experiment consisted of at least 13 explants and was repeated thrice.
3.5 Effect of salt stress on the survival capacity of calli
The difference between the fresh weights of 1-month-old calli before and after 5 weeks of exposure to progressively elevated concentrations of NaCl has been investigated (Fig. 7). The results of this study show that concentrations over 128 mM had noticeably inhibited cultures from growing, while a concentration of 80 mM caused half the maximal reduction effect. This concentration was used as the stressful pressure.
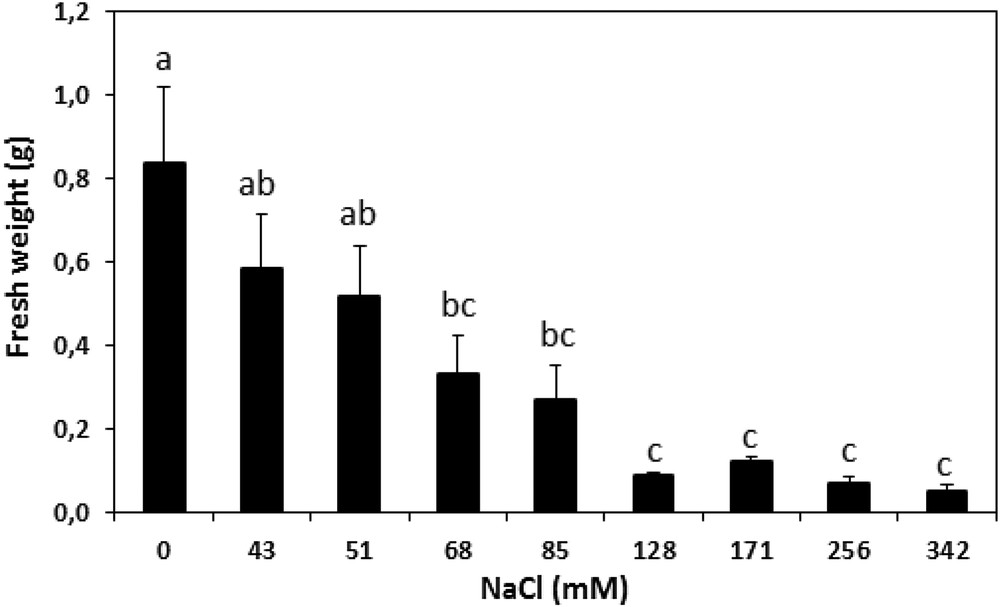
Fresh weight changes (in g) in A. spinosa calli before and after five weeks of exposure to various concentrations of NaCl. Each value is the mean of ten replicates. The vertical bars represent standard errors of the means. Values with the same lower-case letters are not significantly different according to Tukey's multiple range test at P ≤ 0.05.
3.6 Effect of salt stress on H2O2 and MDA concentrations
Fig. 8 shows the effect of stressful conditions on the MDA level and the H2O2 content in stressed and non-stressed calli of A. spinosa. Stressed calli revealed higher concentrations of H2O2 than non-stressed cells, with contents of 17. 37 and 9.93 μmol g−1 FW, respectively. However, the MDA contents were not significantly influenced (P < 0.05; Fig. 8), with only 62. 58 mol MDA g−1 FW recorded in the stressed calli compared to a value of 49.35 mol MDA g−1 FW measured in non-stressed calli under stressed conditions.

Hydrogen peroxide (H2O2) and malondialdehyde (MDA) concentrations in NaCl-stressed calli exposed to salt stress (S + NaCl), NaCl-stressed calli not exposed to salt stress (S–NaCl), in non-stressed calli exposed to salt stress (NS + NaCl), and in non-stressed calli not exposed to salt stress (NS–NaCl). The vertical bars in each panel for each mean value (n = 5) are standard errors. Means with the same letter are not significantly different according to Tukey's multiple range test at P ≤ 0.05.
3.7 Evaluation of the antioxidant status of callus
Our results (Fig. 9) showed that stressed calli responded differently to the stress compared to non-stressed calli. In fact, under stress, the calli exhibit an increase in the antioxidant activity involved in the oxidative metabolism. The induction was significant (P ≤ 0.05) for the catalase and peroxidase activities, which reached 86.38 μmol H2O2 and 38.19 μmol guaiacol mg−1 TSP−1 min−1, respectively, when compared to their controls, which reached only 0.18 μmol H2O2 and 3.79 μmol guaiacol mg−1 TSP min−1, for CAT and PO, respectively (Fig. 9). After one week of stress, the stressed calli revealed also high and statistically significant (P ≤ 0.05) SOD activity (158.71 units mg TSP−1 min−1) compared to 86.43 units mg TSP−1 min−1 in non-stressed cells. However, in both stressed and non-stressed calli, the activity of PPO was similar (P ≤ 0.05; Fig. 9).
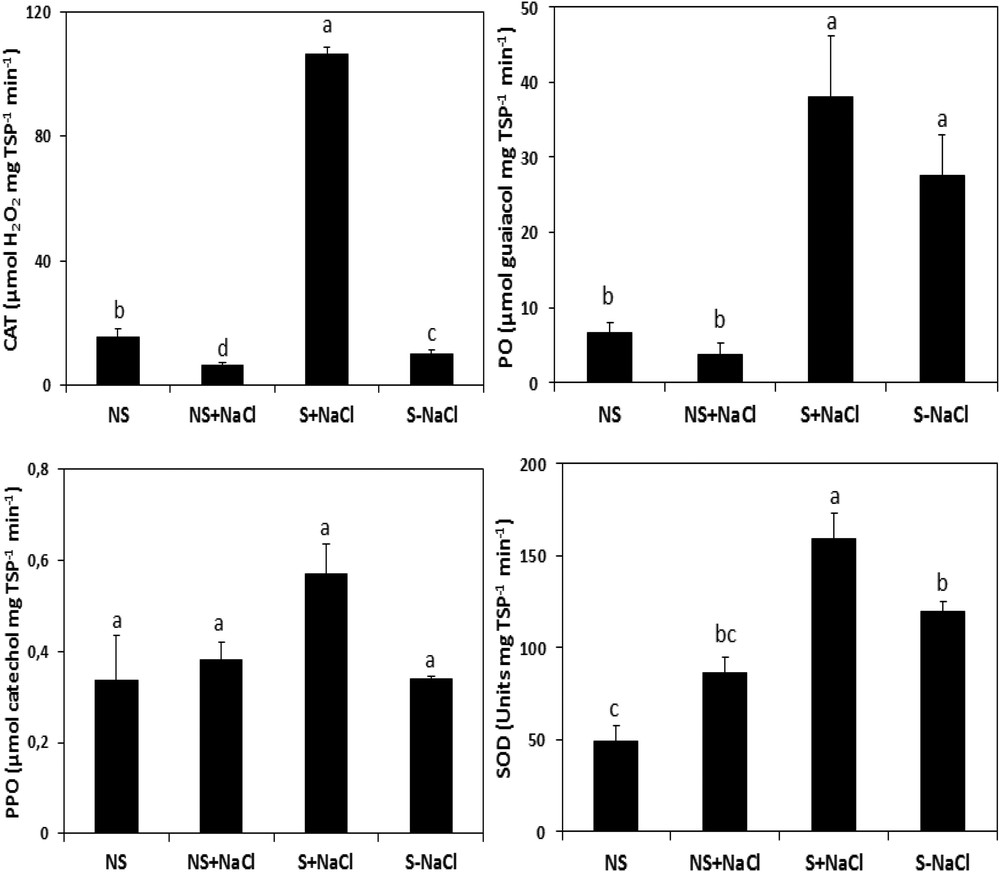
Catalase (CAT), peroxidase (PO), polyphenol oxidase (PPO), and superoxide dismutase (SOD) activities in NaCl-stressed calli exposed to salt stress (S + NaCl), NaCl-stressed calli not exposed to salt stress (S–NaCl), in non-stressed calli exposed to salt stress (NS + NaCl), and in non-stressed calli not exposed to salt stress (NS–NaCl). The vertical bars are standard errors of the mean values (n = 5). Means with the same letter are not significantly different according to Tukey's multiple range test at P ≤ 0.05.
4 Discussion
Because of their sessile nature, plants have adopted a unique plasticity in response to external stimuli enabling whole-body regeneration comparatively to human cells restricted to tissue repair from damage [46]. This so-called totipotency concept is a widely applied process either for plant propagation or for artificial production of bioactive cell extracts and/or compounds. Hormone exogenous application is artificially used to induce the dedifferentiation of plant somatic cells for the production of several economically important products [15–17,20,47]. The extracts from dedifferentiated stem cells are claimed in particular for their richness in antioxidants that may contribute to beneficial protection of human skin cells through the maintaining of their healthy epigenetic profile and the regulation of the length of the telomeres [46,48,49]. Argan tree is highly claimed for its content in valuable compounds, which makes it a new candidate as a source of regenerative skin extracts similarly to apple [17] and tomato [20]. This minimalist system provides as well a coordinated cell-to-cell communication relatively to the complexity of the whole plant [20,31,50,51]. However, callus production could be a critical step in many tissue culture processes involving the reprogramming of a differentiated cell with a determined fate [52]. Economic losses could as well reduce the effectiveness of the process due to contaminations, in particular when glasshouse-forced cuttings or field-grown-tree-derived explants are used. Surface sterilizing should be optimized to insure the cost effectiveness of the system. In this experiment, the maximum of healthy explants were obtained after 20 min immersion in 6% (v/v) sodium hypochlorite, which corresponded approximately to 80% of healthy explants without any adverse effect. Other reports claimed, to the contrary, the effectiveness of HgCl2 [53–56].
Any in vitro response depends on the hormonal balance modulation along with the ability of the tissues to respond to the external stimuli [57]. Although the cell culture of argan was widely reported to be difficult [11], good dedifferentiation rates were obtained using only half-strength of MS mineral salts. Argan callus developed accordingly to the hormones type and concentration, in particular that of auxins (Fig. 2). Auxin-signaling, in promoting cell cycle reentry and reacquisition of cell proliferative competence, is a very elucidated pathway [47]. This makes it the key regulator for the induction of cellular dedifferentiation [58,59]. 2,4D, in particular, was the most reported active auxin used for this purpose [55,60,61] with the previously reported antagonist effect of cytokinins [62,63]. 2,4D combined with low BAP concentrations was favorable to the acquisition of the cell mitotic power, leading to argan callus formation. The axillary buds were found to be strongly callogenic over the other tested explants, which could be due to the pool of innately undifferentiated stem cell lines residing within the meristems, which are reported to support this proliferative activity under exogenic activation [31,47]. Several studies reported that the meristematic stem cells activity contribute powerfully to callus formation [31], while other reports claimed other vegetative parts with more callogenic potential such as leaves [20,61]. The results of this study evidence also darkness as a key parameter for the appropriate induction of healthy calli, which prevents the possible use of UV radiations as an eliciting factor and which could be explained by auxin photosensitivity [64]. Moreover, callus induction was maintained within the pH range of 5.6 to 5.8 equally to the consistent standards [36]. The results of this study show a negative correlation between callus growth and salt constraint severity. Based on the growth performance of the calli, the half growth restriction is correlated to the promotion of the cells’ oxidant power. This growth obstruction was combined with an important increase in ROS, namely H2O2 content, leading cause of the oxidative stress. The results show also a subsequent attenuation of the H2O2 impact in the stressed callus after mobilization of the antioxidant activity, in particular SOD, CAT, and PO activities (Fig. 9), leading to ROS removal by the induced antioxidant power under abiotic and safe elicitation. Moreover, the PPO activity (Fig. 9), associated with phenolic state regulation, seemed to be stable and statistically insignificant, revealing a healthy tissue status insured by the antioxidant defense system exhibited by the cells dedifferentiated under stress. The antioxidant compounds and enzymes’ positive correlation attests also to the important antioxidant power triggered within the stressed tissues [23]. MDA low contents (Fig. 8) refer as well to the established equilibrium between ROS and the antioxidant mechanisms mobilized [65]. To sum up, the results are highlighting the important impact of stress as an elicitation approach. Although 80 mM of NaCl obstruct biomass production by 50% (Fig. 7), the damage was overcompensated by the important promotion of the antioxidant power, which exceeded two times, relatively to the control cells, in particular CAT and SOD activities. NaCl did exhibit a stronger eliciting power compared to polyethylene glycol, in particular the induction of CAT and SOD activities [66], which could be explained by the ionic stress and toxicity, in addition to the osmotic pressure in comparison with polyethylene glycol, which induces only an osmotic constraint.
With the restriction of the use of animal, human, and even the genetically modified plants in cosmetics, researchers are desperately seeking for new innovative ingredients in plant cells [46,48]. Plant dedifferentiated cells represents a safe and sustainable source to achieve efficient production of active compounds in particular. Thus, this protocol will make possible the intensive production of argan cells to benefit from their highly claimed active compounds universally and in areas out of their native habitat, independently of the season and of other environmental restraints. We expect to expand the results of this work to the further establishment of suspension culture and crude extract recuperation out of the cells for their valorization. Further elicitation and precursor feeding are also outlined in our perspectives to elaborate a powerful system for antioxidant production, harvesting, and delivering of high yield of bioactive compounds for cosmetic application.
5 Conclusion
The plant cell culture technology is an alternative to overcome manufacturing problems reported with plant-differentiated cells. The cell-culture approach is also a good alternative in terms of sustainability for any endangered plant species. In this work, we succeeded in establishing in vitro callogenesis of A. spinosa efficiently with good rates (90%). It was possible also to highlight the impact of abiotic stress on to boosting of the antioxidant power of extracts of argan cells in vivo. With the increased awareness for sustainable development, the cost effectiveness of the here-described protocol could be considered with suspension culture establishment and processing to provide successfully argan natural extracts or bioactive compounds for the cosmetic industry with further manipulating of either their genetic and epigenetic status.
Acknowledgements
This work was supported by the Hassan II Academy of Science and Technology and the Ministry of Higher Education and Scientific Research (Morocco).