1 Introduction
The widespread use of pesticides in public health and agricultural programs has resulted in the pollution of water, air, and food that has lead to severe acute and chronic cases of human poisonings [1–6]. The World Health Organization estimates that the incidence of pesticide poisoning in developing countries has doubled between 1987 and 1997 [7]. However, the use of organophosphorus pesticides (OP) has increased considerably due to their low potency and durability as compared to organochlorine pesticides [8], in spite of their risks to human health and the environment. The OP compound malathion [S-1,2(bis ethoxycarbonyl) ethyl O,O dimethyl phosphorodithioate] is used extensively throughout the world, especially in developing countries, to control major arthropods in public health programs, animal ectoparasites, human head and body lice, household insects and to protect grain in storage [9–12]. It kills insects by contact or vapour action. OP compounds are primarily recognized for their ability to induce toxicity in mammals through the inhibition of acethylcholinesterase (AChE), leading to the accumulation of acetylcholine and subsequent activation of cholinergic muscarnic and nicotinic receptors [13]. Also, a study suggests that OP compounds may exert direct action on muscarnic and nicotinic receptors, when their concentration in the circulation exceeds micromolar levels [14]. The toxicity of OP agents may be due, at least in part, to the formation of reactive oxygen species (ROS), leading to lipid peroxidation LPO, which is generally assessed by an increase in the levels of thiobarbituric acid reactive substances (TBARS) [13,15,16]. In addition, malathion is a lipophilic substance; it may enhance LPO by directly interacting with the cellular plasma membrane [13]. It is evident that LPO is accompanied by an alteration (inhibition or activation) in the antioxidant defence system in different organs following acute, subchronic and chronic exposure to OP compounds [13,17–22]. Nevertheless, recent evidence suggests that exposure to different stress models and concentrations (acute, repeated and chronic restraint stress) have an important implication in the alteration of the antioxidant defence system [23]. The liver is the major metabolizing and the most active organ for mediating bio-activation of thiono-organophosphates [24]. It is considered among the primarily targets for malathion toxicity [25]. Thus, the aim of the present investigation was the evaluation of liver damage after subchronic exposure to malathion and the estimation of the antioxidant status using non-denaturing electrophoresis followed by activity-staining [26].
2 Materials and methods
2.1 Chemicals
Malathion (fyfanon 50 EC 500 g/l) of commercial grade was used in this study. 2-thiobarbituric acid (TBA); 2,6,-di-tert-butyl-4-methylphenol (BHT); trichloroacetic acid (TCA); Acetylthiocholine iodide; 5,5-dithio bis(2-nitrobenzoic acid)(DTNB); 3-(4,5-dimethylthiazol-2-yl)-2,5-diphenyltetrazolium bromide (MTT), phenazine methosulfate (PMS), magnesium chloride (MgCl26H2O); potassium iodide; sodium thiosulfate (Na2S2O3) were obtained from sigma-Aldrich Co (Germany).
2.2 Animals and treatment
Adult male wistar rats (170–180 g), procured from Tunisian Society of Pharmaceutical Industries, were kept in clean plastic cages and allowed to acclimatize in the laboratory environment from 7 days. The ambient temperature was () with a 12-h dark/light cycle. Balanced food and drinking water were given to the animals ad-libitum.
Animals were randomly divided into two groups of 12 animals and treated for 32 days. The first group received orally 1 ml corn oil via gavage. The second received instead 1 ml corn oil containing 100 mg Mal/kg body weight per day. The test concentration of malathion is calculated directly from commercial grade. Body weight and food intake were recorded daily throughout the experiment. All the procedure was in accord with the Guidelines for Ethical Conduct in the Care and Use of Animals. At the end of the treatment, 24 hours after the last dose of malathion [26], animals were killed by decapitation, without preliminary anaesthesia, and arteriovenous blood wss taken quickly for centrifugation (3000 g × 10 min) at 4 °C. The liver was removed by transverse abdominal incision and kept frozen at −80 °C.
2.3 Pseudocholinesterase PchE activity assay
The pseudocholinesterase activity of plasma was estimated by the method of Ellman [27], using acetylthiocholine iodide. The rate of hydrolysis of acetylthiocholine iodide is measured at 412 nm through the release of the thiol compound which, when reacted with DTNB, produces the color-forming compound TNB.
2.4 Aspartate aminotransferase ASAT and alanine aminotransferase ALAT assay
The serum activities of aspartate aminotransferase (ASAT) and alanine aminotransferase (ALAT) were determined spectrophotometrically at 340 nm using established clinical chemical methods. The rate of reduction in the concentration of NADH, is directly proportional to the ASAT or ALAT activity in the sample [28]. These values are reported in units per litre (UI/l).
2.5 Lipid peroxidation
Each liver was homogenised separately in Tris Buffered Saline (TBS) pH 7.4. The homogenates were centrifuged at 4 °C at 3000 g and the supernatants were stored at 4 °C for determining lipid peroxidation. Each supernatant was mixed with the (BHT)-TCA solution, and centrifuged at 1000 g for 10 min. Malonedialdehyde (MDA) is an end product of the oxidation of polyunsaturated fatty acids and can react with TBA to produce a complex that can be determined spectrophotometrically at 530 nm. So, the supernatant was mixed with 0.5 N HCl and 120 mM TBA in 26 mM Tris, and heated at 80 °C for 15 min. After cooling, the absorbance was determined using a BIORAD UV-visible spectrophotometer. Lipid peroxidation in homogenates was assessed in terms of thiobarbituric acid reactive substances (TBARS) produced [29]. The protein content in liver homogenates was quantified by the method of Bradford [30], using bovine serum albumin as a standard.
2.6 Analysis of antioxidant enzyme activities using native PAGE
2.6.1 Non denaturing gradient gel electrophoresis
500 mg of liver were homogenized with the extract buffer [31] added with benzamidine (0.1 M) for inhibiting proteases. The homogenate was centrifuged at 4000 g for 30 mn at 4 °C, and the pellet discarded. Protein concentration was determined according to the method of Bradford [30]. The equivalent of 25 μg of each sample protein were mixed with an equal volume of sample buffer containing glycerol, 0.1 M Tris–HCl, pH 6.8, 0.1% and blue bromophenol. The mix of each sample was loading, respectively, per lane on the gel realized on 8% polyacrylamide. At the end of the electrophoretic migration, gels were incubated with staining solution to identify the enzymes' activities.
2.6.2 Catalase (CAT)
Native PAGE was realized on 8% polyacrylamide gel at 200 V and 4 °C. The gel was incubated in the first step in sodium thiosulfate solution (60 mM) with 3% H2O2 for 30 to 60 seconds. Secondly, the first solution was discarded and the gel was incubated in a second solution containing 90 mM KI and acid acetic (glacial). Catalase activity is indicated by the presence of achromatic bands [32].
2.6.3 Superoxide dismutase (SOD)
Native PAGE was realized on 10% polyacrylamide gel at 200 V and 4 °C. Enzymatic activities were developed in staining solution containing 50 mM Tris-HCl (pH, 8.5), 10 mg MTT, 6 mg PMS, 15 mg MgCl26H2O in daylight. The enzyme bands are seen as pale zones on a dark blue background [32].
2.6.4 Quantitative estimate
For the quantitative estimate we have to use an application software image J that is a special Plugin for convenient densitometry of 1D gels and also dot blots.
2.7 Statistical analysis
All data are expressed as mean ± SEM by factorial ANOVA, followed by Student test. The relationships between MDA levels and serum transaminases (ASAT and ALAT) activities were assessed by linear regression analysis. Differences between groups were considered significant when .
3 Results
3.1 Physical observation
No signs of toxicity were observed in malathion-treated animals until the end of the experiment. In addition, our results showed that malathion administration caused no significant decrease in mass gain between controls and malathion-treated animals.
3.2 Blood estimations and lipid peroxidation
Malathion at dose of 100 mg/kg/day increased significantly hepatic MDA and serum activities of aspartate aminotransferase (ASAT) and alanine aminotransferase (ALAT) and decreases PchE activity – see Table 1.
Effect of subchronic treatment with malathion on TBARS level measured in liver, on plasma PChE activity, and on transaminases activites (ASAT, ALAT) of rats
Treatment | MDA nmol/mg protein | PChE nmol/min/mg protein | ASAT UI/l | ALAT UI/l |
Control (n=12) | 5.5 ± 0.24 | 12.877 ± 0.259 | 47.25 ± 2.78 | 19.32 ± 1.25 |
Malathion (n=12) | 7.05 ± 0.24⁎⁎⁎ | 4.918 ± 1.496⁎⁎⁎ | 70.10 ± 7.40⁎ | 27.92 ± 1.55⁎⁎⁎ |
⁎ as compared with control.
⁎⁎⁎ as compared with control.
3.3 Relationship between serum ASAT and ALAT activities and hepatic MDA
There was a significant positive correlation between serum ASAT and ALAT activities and hepatic MDA; see, respectively, Figs. 1 and 2.
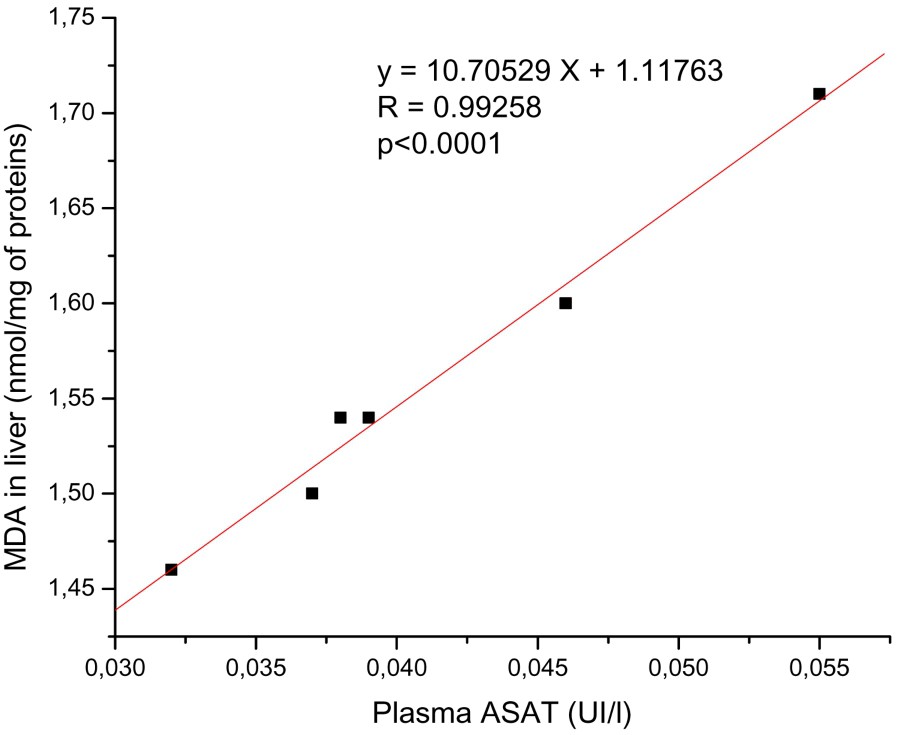
The relationships between the levels of hepatic MDA and plasma ASAT activity. There is positive correlation between hepatic MDA and plasma ASAT activity. y: Equation of the linear fit; r: correlation factor.
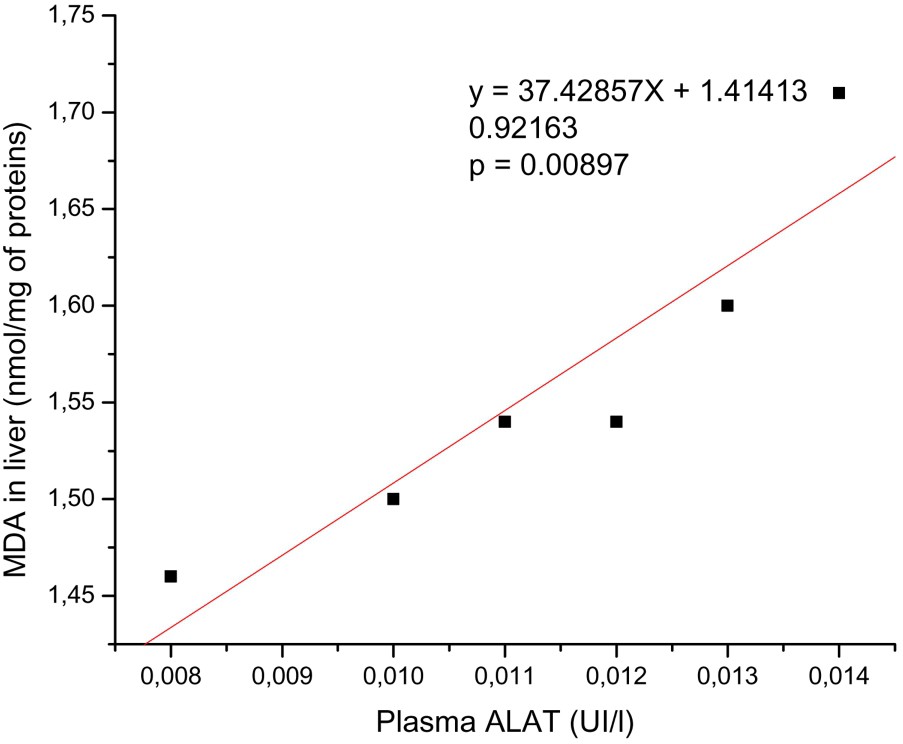
The relationship between the levels of hepatic MDA and plasma ALAT activity. There is positive correlation between hepatic MDA and plasma ASAT activity. y: Equation of the linear fit; r: correlation factor.
3.4 Hepatic antioxidant enzyme activities
In native PAGE, our results showed a slight increase in antioxidant enzymes activities CAT and SOD, respectively (16%) (Fig. 3(a), (b)) and (11%) (Fig. 4(a), (b)).
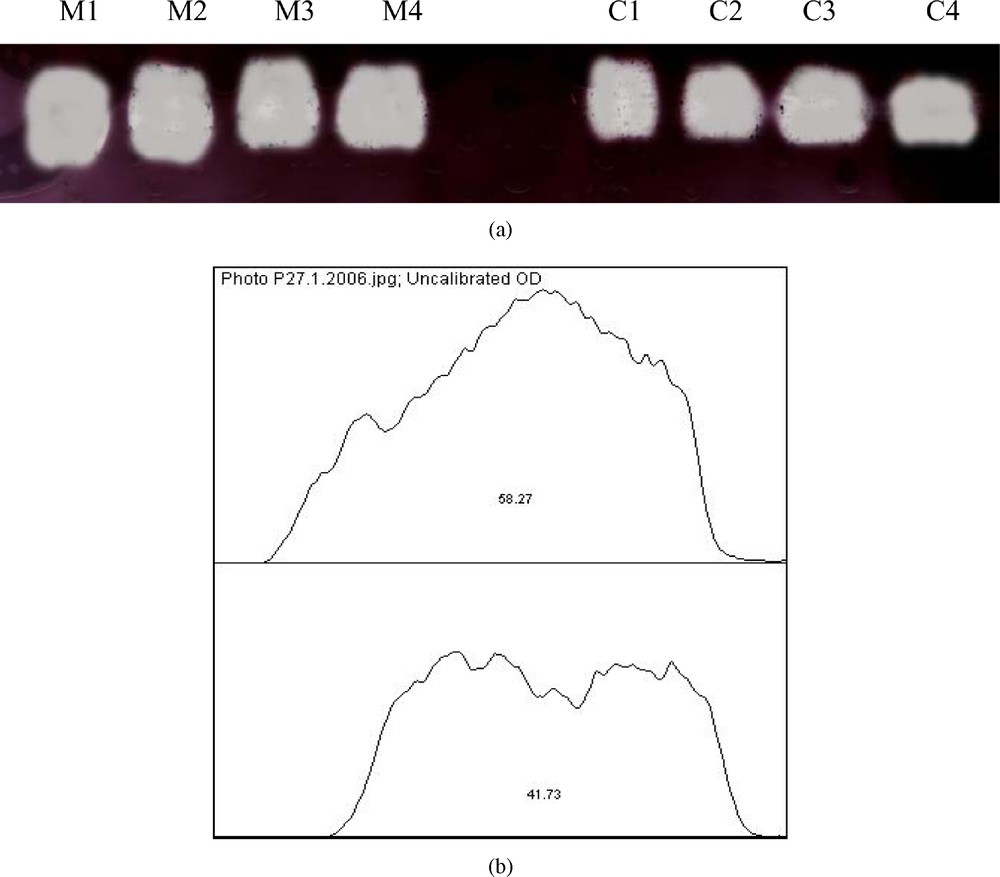
(a) Effect of malathion on hepatic catalase (CAT) activity using native PAGE (n=4); (b) quantitative estimation of hepatic (CAT) activity. The increase of CAT activity in treated rats (M) was about 16% compared to control rats (C).
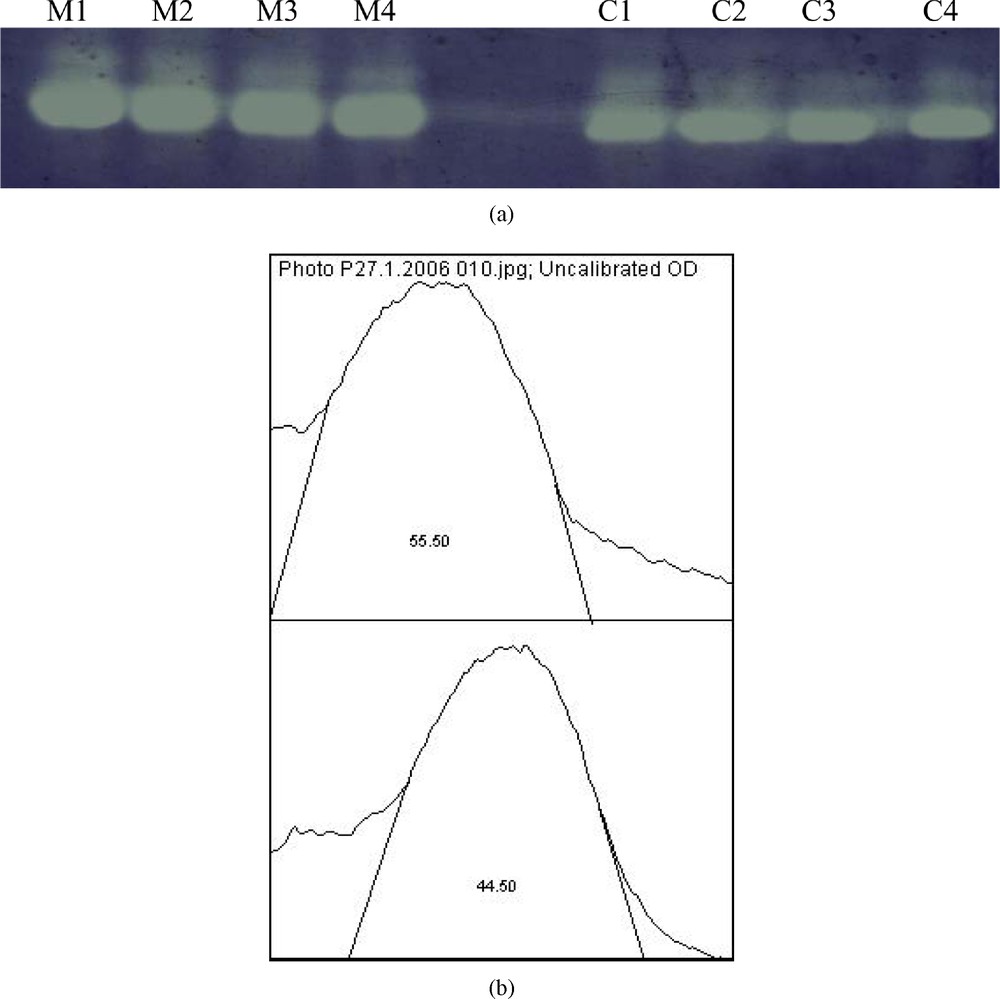
(a) Effect of malathion on hepatic superoxide dismutase (SOD) activity using native PAGE (n=4); quantitative estimation of hepatic (SOD) activity. The increase of SOD activity in treated rats (M) was about 11% compared to control rats (C).
4 Discussion
The results of this study indicate that malathion in subchronic exposure at a dose of 100 mg/kg per day decreases significantly PchE activity. In addition, malathion exposure increases significantly the hepatic TBARS level of malathion-exposed rats. These results are somewhat similar to those found in the liver of rats exposed to malathion acutely [13] or subchronically [21]. Elevated levels of TBARS in liver can be an indicator of increased lipid peroxidation (LPO) in hepatocyte membranes that suggests the participation of free-radical induced oxidative cell injury in mediating the toxicity of (OPs) pesticides. On the other hand, our study shows a significant increase in serum activities of ASAT and ALAT considered like markers enzymes of hepatocyte cytolysis. Indeed, ASAT and ALAT are two hepatic enzymes which will be released in blood in the event of cellular destruction proportionally to the intensity of the cellular aggression [33]. The recorded increase of ASAT and ALAT levels may be due to increased LPO. Indeed, we have seen a high positive correlation between serum rates of ASAT and ALAT and hepatic MDA level. The formation of MDA in liver is a sign of lipid membrane degradation that involving the deterioration of cellular integrity and the release of these enzymes in blood (see Figs. 3 and 4). In the same way, Nemcsok [34] has noted a tissular necrosis indicated by the rise in the serum activities respectively of the ASAT and the ALAT following the exposure of fish (carp) to an OP compound. Additionally, histological examinations of the hepatic tissues of malathion-exposed rats revealed degenerative changes in the liver in the form of parenchymatous degeneration. The areas of cytoplasm were observed to be deprived of normal cellular organellae, with an increased number of lipid vacuoles. These changes confirm the focal degeneration of the cytoplasm [35]. Among the possible explanations for this cytolysis, is the leakage of hepatocyte's biomembrane, causing efflux of K+, influx of Na+ and concomitant influx of water into the cell that can cause cytotoxic edema [13]; it is well elucidated as one of the most important effects of LPO. Indeed, OP pesticides are known to affect the activity of membrane-bound ATPases [36–38]. Also, the lipophilicity of organophosphorus insecticides favors their incorporation in membranes [39,40]. The incorporated compounds elicit physical and chemical perturbations and presumably a dysfunction of the membranes in their phospholipids bilayer structure. Therefore, the primary effects of insecticides may result from physicochemical changes at the level of membrane lipid structure and organization [39].
On the other hand, some studies have reported, in acute malathion exposure [13], a decrease in detoxifying enzymes like glutathione S-transferases (GST), which produce less toxic forms by conjugation [41] and carboxylesterases, responsible for the rapid degradation of malathion and malaoxon in mammals [42,43]. Thus, it is obvious that this leads to insufficient detoxification of malathion in the liver. Indeed, it is interesting to note that Blasiak et al. [44] reported that malathion has an acute cytotoxic effect leading to cellular death in human lymphocytes, without causing damage to the DNA; but its active metabolites, malaoxon and isomalathion act on DNA, breaking its chains. Each mole of undetoxified xenobiotic or its metabolites can potentially produce multiple moles of active oxygen species in redox-cycling. Thus, we can suggest an accumulation of active oxygen species that can exceed the organism's capacity to neutralize them and consequently, may produce cytotoxic effects. This result might be due to time and concentration-dependent mechanisms. Indeed, Gultekin et al. [45] confirmed in vitro that MDA values increased with increasing organophosphorus compound concentration and incubation period, but SOD and CAT activities decreased with increasing its concentration and incubation period. In the same way Rosety et al. [46] have demonstrated a time course variation of antioxidant enzyme activities in fish exposed to a sublethal concentration of malathion for 24, 48, 72 and 96 h. They reported that superoxide dismutase (SOD), catalase (CAT) and glutathione peroxidase (GPX) activities were altered significantly from 24 h onward. Supporting this idea, we can suggest that hepatic SOD and CAT activities pass by two phases during the subchronic treatment with malathion. Firstly, a gradual increase, reaching a maximum, considered like an initial adaptive response to increased oxidative stress. Secondly, a gradual decrease when free radicals concentrations exceed their capacity to neutralize them. Related to our work (subchronic protocol and high dose) we can suppose that enzymes in our zymograms were in the second phase of enzymatic activity. This explanatory assumption needs to be elucidated in vivo by the investment of the kinetics of antioxidant enzymes activities and their mRNAs after oral exposure to malathion in subchronic treatment. Generally, the accumulation of free radicals can involve cytotoxicity and enormous damage to DNA, as well causing an alteration in the expression of genes including those of the antioxidant system.
In conclusion, our evaluation shows that malathion in subchronic exposure disrupted the cellular integrity of hepatocytes; thus more attention is needed to limit the use of OP insecticides, particularly malathion, which is contained in most agricultural pesticides used in the developing countries, as much as possible and reduce their entrance into the human food cycle via environmental pollution.