1 Introduction
The contamination of soil and water with lead (Pb) mostly results from human activities. Various industries, such as mining and smelting, the past and present use of lead in paints, gasoline and linings have resulted in its spreading all over the world. Lead is extremely toxic to all intermediates of food chains [1–3]. In humans, its effects have been thoroughly characterised, and include nephropathy, saturnism or colic-like abdominal pains [4]. Chemical and physical techniques to remove lead from soils have been developed [5,6]. They are expensive and applicable only to small areas. Recently, heavy metal extraction by plants has emerged as a promising, cost-effective alternative to reduce heavy metal levels in soils [7]. The first step in the implementation of phytoextraction strategies is the identification of plant species able to: (i) tolerate large quantities of heavy metals in the soil [7]; and (ii) absorb large amounts of at least one trace element. Over 400 plant species have been described as presenting both these properties [8]. A majority of them have evolved to cope with Ni [8], one of several trace elements (Cu, Ni, Zn, Cd, As, Se, Pb) representing a hazard to ecosystems. Lead tolerant and/or lead accumulating plants are rather exceptional. Scientists are therefore on the lookout for novel species with phytoremediation potentials towards this element.
Grass pea (Lathyrus sativus L.) is a ubiquitous annual leguminous crop with such an amazing capability to tolerate harsh growth conditions that it is considered one of the most promising sources of genes of interest [9]. It is poorly affected by abiotic stresses, such as water-logging and is resistant to various insects and pests [9–14]. Due to its low nutriment requirements, it can thrive in poor soils, and also in very arid environments [15]. We have previously shown that some drought tolerant tropical legume species cope with water deficit by maintaining cell homeostasis through control over autolytic processes [16–19]. Furthermore, our results strongly suggest that these characteristics could provide general tolerance to abiotic and even biotic stresses. In the present study, we hypothesized that grass pea, due to its multi-tolerance, possessed the ability to deal with stress at the cellular level, and we investigated its capacity to cope with lead in the soil solution. In particular, we report on grass pea's lead-absorption and storage capacities in root tissues and on the impact of lead on mineral homeostasis for calcium, copper and zinc.
2 Materials and methods
Lathyrus sativus (L.) seeds corresponding to an Indian local line “Raipur”, were germinated on Murashige and Skoog medium (Kalys, France) under sterile conditions. Seedlings of similar size were transferred to expanded vermiculite irrigated with 1/10 Hoagland's N°2 medium (pH 5.0) (Sigma, France). Five days later, plants were transferred to liquid growth medium (phosphate free 1/10 Hoagland's N°2 medium, pH 5.0), aerated continuously and supplemented (or not) with 0.5 mM Pb(NO3)2. When in the liquid medium, plants were cultured under controlled conditions: 12 h/12 h photoperiod with 25/23 °C day/night temperatures; 70% RH; 400 μmol photons m−2 s−1. After 96 h of exposure to lead, the roots were collected and briefly rinsed in phosphate free 1/10 Hoagland medium. For EDTA treatment, roots were soaked in 1 mM EDTA (Sigma, France) for 1 h. Six plants were used for each treatment. Root systems (approximately 100 mg each) were dried at 35 °C for seven days, and digested under pressure in sealed vessels containing suprapur nitric acid (65% m/v). Lead and calcium levels were determined by inductively coupled plasma optical emission spectroscopy (ICP-OES), using a Varian Vista MPX instrument.
Tolerance indexes (TI) for roots and shoots were calculated according to Wilkins's equation [20] where MLPb and MLC represented mean length of longest roots (or shoots) in 1/10 Hoagland's-leaded and lead-free solutions, respectively: .
Leaf relative water content was determinated according to equation of Barrs and Weatheley [21] where FW, DW and TW represented fresh, dry and turgid weight: .
3 Results
After 96 h of exposure to lead as 0.5 mM Pb(NO3)2 in P-free modified Hoagland's liquid medium under controlled conditions, survival rate amongst grass pea plants was 100%. Plant growth, however, was affected, as shown by the tolerance indexes measured for roots and shoots (Table 1). Unsurprisingly, tolerance index (TI) values revealed that root elongation was more affected by the presence of lead than shoot elongation (Table 1). Furthermore, the roots whitish color in the control plants had evolved to a light brown in the lead-treated plants. Average root dry biomass was reduced in the lead-treated plants, as indicated by the dry biomass ratios between lead-treated and control plants (Table 1). In addition, leaves of lead-treated plants were smaller than those in the control plants, although exposure to the pollutant did not affect the number of leaves per stem (data not shown).
Tolerance indexes (TIPb) to lead and dry biomass produced by grass pea (Lathyrus sativus L.) plants grown (96 h) in 1/10 modified Hoagland's medium supplemented with 0.5 mM Pb(NO3)2 (DWPb) or not (DWC)
TIPb | DWPb/DWC | |
(%) | ||
Roots | 48.0 ± 14.4 | 443 ± 11.2 |
Shoots | 75.4 ± 9.3 | 80.1 ± 18.7 |
The water status in leaf tissues was not affected by lead since RWC values remained equivalent in both groups of plants (Table 2).
Relative water content in the leaves of grass pea (Lathyrus sativus L.) plants grown (96 h) in 1/10 modified Hoagland's medium supplemented with 0.5 mM Pb(NO3)2 (Pb) or not (C)
RWC (%) | |
C | 90.8 ± 0.3 |
Pb | 93.0 ± 0.6 |
Analyses of mineral contents by ICP-OES in the roots of control plants showed that they were lead-free (Fig. 1). On the other hand, lead-exposed plants accumulated an average of 153.0 mg of lead per g of dry root material (Fig. 1). Rinsing the root systems with an EDTA solution prior to mineralization resulted in a slight decrease in the quantity of accumulated lead in the roots (138.0 mg Pb g−1 DW) (Fig. 1). Like Pb contents, Na contents increased in the roots of Pb-treated plants (data not shown). On the other hand, the amounts of calcium, zinc and copper were reduced by 6, 2 and 3.5-fold, respectively, in response to the lead treatment (Figs. 2–4). The potassium content was also reduced (data not shown). In general, root treatment with EDTA had a slight impact on the mineral contents (Figs. 2–4).

Lead content in the roots of grass pea (Lathyrus sativus L.) plants grown (96 h) in 1/10 modified Hoagland's medium supplemented with 0.5 mM Pb(NO3)2 or not. C: control plants; Pb: leadtreated plants; Pb + EDTA: lead-treated plants rinsed with EDTA. Values are means ± (SD) (n=6).
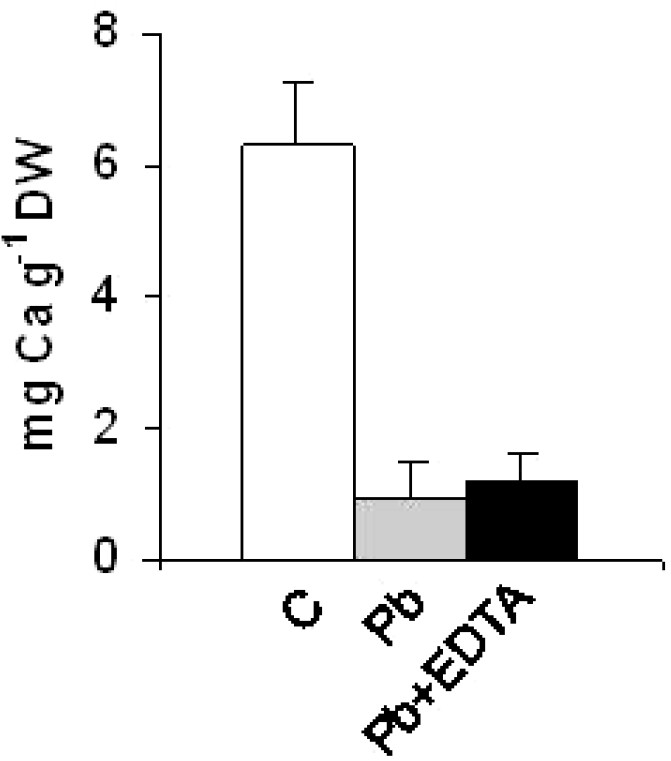
Calcium content in the roots of grass pea (Lathyrus sativus L.) plants grown (96 h) in 1/10 modified Hoagland's medium supplemented with 0.5 mM Pb(NO3)2 or not. C: control plants; Pb: leadtreated plants; Pb + EDTA: lead-treated plants rinsed with EDTA. Values are means ± (SD) (n=6).

Zinc content in the roots of grass pea (Lathyrus sativus L.) plants grown (96 h) in 1/10 modified Hoagland's medium supplemented with 0.5 mM Pb(NO3)2 or not. C: control plants; Pb: leadtreated plants; Pb + EDTA: lead-treated plants rinsed with EDTA. Values are means ± (SD) (n=6).
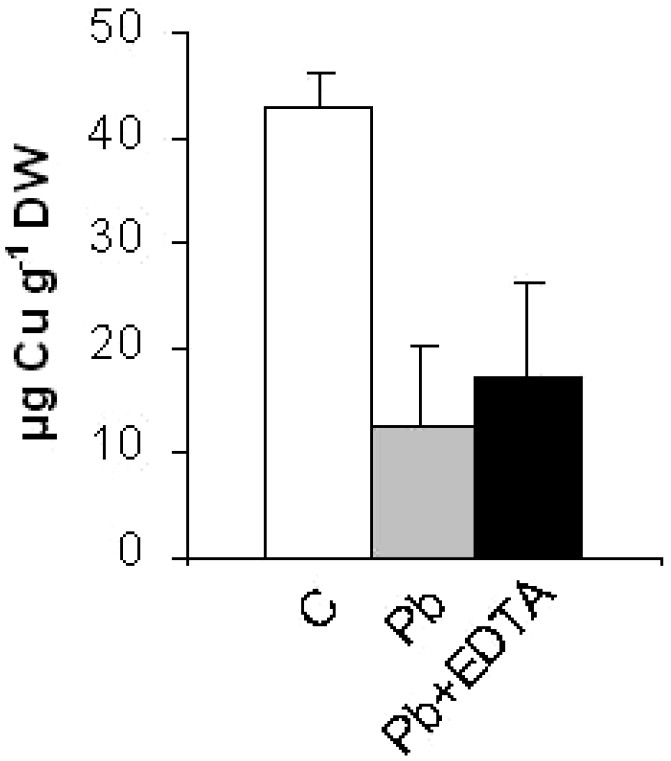
Copper content in the roots of grass pea (Lathyrus sativus L.) plants grown (96 h) in 1/10 modified Hoagland's medium supplemented with 0.5 mM Pb(NO3)2 or not. C: control plants; Pb: leadtreated plants; Pb + EDTA: lead-treated plants rinsed with EDTA. Values are means ± (SD) (n=6).
4 Discussion
Precise control over the amounts of Pb ions available to plants growing in a soil is technically difficult because lead bio-availability depends highly on soil characteristics [22]. The grass pea plants (Lathyrus sativus L.) were therefore exposed to a modified phosphate-free Hoagland's liquid medium supplemented with 0.5 mM Pb(NO3)2 for 96 h. Their capacity to cope with the pollutant was analyzed by comparing lead-exposed plants to control plants exposed to the Pb-free medium.
The 100% survival rate observed at the end of the lead treatment and the absence of visual damage to the leaves suggested that grass pea plants presented an elevated tolerance to Pb ions, under our experimental conditions. Morphological differences between lead-treated and control plants concerned their size. Lead reduced grass pea plant growth rates in the roots and shoots and limited overall biomass production. This appeared to be a general response to lead that was previously observed in Vicia faba, Pisum sativum and Phaseolus vulgaris [3,23–25]. Since hydration levels (RWC%) in leaf tissues were slightly higher in lead-treated than in control plants at the end of the treatment, it is most likely that lead-induced stomatal closure developed over the course of the experiment and that atmospheric carbon fixing activities were compromised as a consequence.
Mineral analyses showed that lead-treated plants contained six times less Ca compared with control plants. Due to the importance of Ca in many aspects of plant cell biology [26], tolerance to low Ca conditions could therefore represent an important aspect of tolerance to lead in grass pea, as is the case in other higher plant species [27,28]. Reduced calcium contents in lead-exposed plants has previously been observed in other species, such as rye, maize, tomato and mustard varieties [27] and could result from the inhibition of Ca transporters by toxic lead ions [28–32] and/or replacement of Ca ions with Pb ions due to the high affinity of the latter for Ca binding-sites on biological structures [26,33–35]. In ground dried husks of Lathyrus sativus plants, efficient replacement of Ca by Cd at the surface of the biomass has been reported [36]. However, in the present experiment, simple replacement of Ca with Pb was not sufficient to account for the large quantities of lead found in the roots. Several studies have shown the poor molecular-specificity of some Ca transporters [26]. It is therefore possible that the more abundant Pb ions competed with Ca ions [28,32] and were preferentially transported in and out of grass pea root tissues.
To a lesser extent, the negative impact of lead on Ca content was also observed for other essential nutrients (Zn, Cu, K). Like for Ca, a reduction in Zn, Cu and K contents has previously been observed in response to lead exposure in Cucumis sativus and Zea mays plants, as a result of a possible blockage of the transporter proteins by the pollutant [22,37,38]. On the other hand, the lead-treated grass pea plants accumulated more Na than the controls. Whether Na was actively absorbed to compensate for the loss in K, as was suggested in the case of Pisum sativum roots exposed to lead [39], remains to be elucidated. Despite the important mineral unbalance induced by lead in their roots, grass pea plants remained visually intact, a response to be expected from a plant known to thrive in poor quality soils [9].
Lead-treated grass pea plants contained an average of 153 mg Pb g−1 DW in their roots. Rinsing the root systems with EDTA prior to mineral analysis barely impacted lead content. Desorption solutions including chelators of divalent cations (EDTA, HEDTA) have been used previously on lead-treated root systems to differentiate between exchangeable and non-exchangeable Pb ions [25]. In corn and ragweed, short-term Pb uptake (30 min) mostly consisted of exchangeable Pb [25]. Conversely, long-term Pb uptake, as was studied in the present experiment, mostly involved non-exchangeable Pb. The efficient immobilization of Pb ions on grass pea roots could represent a new and interesting feature for the development of phytoremediation strategies requiring that large amounts of the contaminant remain tightly bound to plant tissues.
Comparing lead accumulation capacities of plant species is not easy because experimental conditions differ significantly. To best assess grass pea lead accumulation capacity, mostly results from studies on plants grown in hydroponic conditions have been considered. After a short exposure to lead (three days with 500 μM of Pb(NO3)2), 138 mg Pb g−1 DW were measured in the roots of Brassica juncea plants [40]. Roots of Pisum sativum exposed to 1 mM of Pb(NO3)2 for four days accumulated up to 50 mg Pb g−1 DW [23] and 21 mg Pb g−1 DW were found in the roots of Helianthus annuus plants exposed to 125 μM of Pb(NO3)2 for six days [41]. Longer exposure (12 days) to 20 μM of Pb(NO3)2 led to an accumulation of 28.7 mg Pb g−1 DW in Thlaspi rotundifolium roots [25]. Under our experimental conditions (0.5 mM of Pb(NO3)2 for 96 h), grass pea plants absorbed 153 mg Pb g−1 DW in their root systems. It appears that grass pea presents lead accumulation capacities that are equivalent to those of efficient root accumulators like Brassicae juncea and Thlaspi rotundifolium. Combined with the fact that lead was tightly retained by root tissues, these accumulation data clearly demonstrate that the grass pea has an interesting potential, as an efficient lead phytoextracting species in rhizofiltration setups [3]. Studies are now in progress to better understand the mechanisms involved in lead absorption by the grass pea.
Acknowledgements
The authors thank Dr. F. Lambein of Laboratory of Physiological Chemistry at the University of Ghent for kindly providing them with grass pea seeds.