1 Introduction
As defined by Lwoff [1], viruses are obligate parasites that use the host cell machinery to produce their progeny. Consequently, viruses have to adapt the different steps of their infection cycle (e.g. translation of proteins, genome replication, cell-to-cell movement, and host-to-host transmission) to the characteristics of their hosts. Plant viruses have to deal with plant-specific features that restrict virus transmission to other plant hosts, such as:
- • the immobility of plants, leading to limited possibilities for virus transfer among hosts via direct plant-plant contact;
- • a solid and virus-impermeable wall made of cellulose, hemicellulose, and pectin that surrounds all cells and limits both entry and exit of viruses.
While the tunnel-like connections known as plasmodesmata [2] can be used by viruses to traffic to adjacent cells within the plant, no plasmodesmata open to the outside, excluding direct escape of the virus from the infected plant via this route.
Viruses have evolved different strategies to circumvent this cell-wall barrier. In one such strategy, some plant viruses accumulate in plant organs used for producing progeny (e.g. seeds, tubers and bulbs). According to current knowledge on viral transmission, 6% of the described plant viral species are transmitted directly through seeds [3–5], and there are some rare reports of virus transmission through pollen [6]. However, plant viruses most often use external “tool(s)” to actively break the cell wall, thus allowing escape of virus particles. For some viruses, mechanically injured tissues from infected plants can be transferred to non-infected plant by human or animal activity, or winds. Although this strategy appears to be very efficient for some virus species (e.g. Potato virus X and Tobacco mosaic virus), it is not a common means of transmission for the vast majority of plant viruses. Indeed, most plant viruses use a partner, known as a “vector”, for efficient transmission to new hosts. A vector is a mobile organism able to gain access to the plant cell cytoplasm by breaking through the cell wall and membrane using their feeding organs, and that moves frequently among plants. Root nematodes, parasitic fungi and plant-feeding arthropods, particularly insects, have been described as efficient vectors in plant-to-plant transmission of viruses.
As stated elsewhere in this volume, aphids have long been the subject of intense research because:
- • they cause large economic losses (see Dedryver et al. [7]);
- • they have adopted a complex life cycle with alternating asexual and sexual phases (see Simon et al. [8]);
- • they show remarkable phenotypic plasticity (see [8,9]).
Another important aspect addressed here is the fact that aphids are certainly by far the most frequent and efficient vectors of plant viruses. They transmit hundreds of plant pathogens, mostly viruses, and this property has engendered much research on aphid population dynamics and its role in viral epidemiology (see [7,10]), and also on aphid feeding behavior coupled with dissection of the anatomy and ultrastructure of aphid stylets and digestive tracts. Aphids mouthparts are remarkably adapted to their feeding habits, and the thin and flexible stylet bundle is perfectly able to pass intercellularly without damaging plant tissues [11,12]. Prior to feeding from the phloem of a host plant, aphids have the extraordinary capacity to sample cell content during brief intracellular probes into epidermal and/or mesophyll cells without killing them [12,13]. Once the plant has been sampled and accepted by the aphid, the stylets are introduced deeply under the epidermis until they reach the sieve elements. During the steps of the feeding process, aphids produce different salivas with different compositions and functions (for a detailed review, see [14]). Remarkably, aphids can acquire viruses at all steps of this feeding process, early during intracellular probes within the cytoplasm of epidermal or mesophyll cells, as well as later in the vascular system. In a similar manner, the virus can be inoculated both in superficial and in deeper tissues during the feeding process. Thus, aphids are organisms that can acquire and inoculate any viral taxon within plants, whatever their tissue specificity.
Depending on the aphid species and viruses studied, scientists observed the existence of different patterns of aphid-virus interactions. Using the tools-at-hand more than half a century ago, the first approaches to distinguishing between the different modes of virus-vector interaction were made by measuring the time lapse required for virus acquisition from an infected plant, and inoculation to a new healthy host, as well as the time the virus was retained as an infectious unit within the vector (Table 1). Based on the results obtained, three transmission modes were defined:
- • the non-persistent mode [15], with viruses acquired within seconds and retained for only a few minutes by their vectors;
- • the semi-persistent mode [16], with viruses acquired within minutes to hours and retained for several hours;
- • the persistent mode [15], with viruses that require minutes to hours for acquisition and that can be retained for very long periods, often until the vector dies.
Different modes of plant virus transmission by insects with piercing-sucking mouthparts (adapted from [155]).
Transmission modesa | Circulative | Non-circulative | ||
Propagative | Non-propagative | Non-persistent | Semi-persistent | |
Acquisition timeb | Minutes to hours | Minutes to hours | Seconds to minutes | Seconds to hours |
Retention timec | Days to months | Days to months | Seconds to minutes | Minutes to hours |
Inoculation timed | Minutes to hours | Minutes to hours | Seconds to minutes | Seconds to hours |
Association with vectorse | Internal | Internal | External | External |
Replication in vectors | Yes | No | No | No |
Requirement of a HC | No | No | Yes and no | Yes and no |
a These modes of transmission are widely accepted for virus transmission by piercing-sucking insects. As discussed in the text, they sometimes also apply to other types of vectors.
b Time required for a vector to efficiently acquire virus particles upon feeding on an infected plant.
c Time during which the virus remains infectious within its vector, after acquisition.
d Time required for a vector to efficiently inoculate infectious virus particles to a new healthy plant.
e Internal means that the virus enters the inner body of its vector, passing through cellular barriers. External means that the virus binds the cuticle of the vector and never passes through cellular barriers.
Additional investigations (including insights provided by electron microscopy) distinguished viruses remaining on the outside of the vectors (mouthparts or foregut) from those traversing the gut epithelium and ultimately colonizing salivary glands. Kennedy et al. [17] and Harris [18] accordingly proposed a new classification of viruses, where the term non-circulative grouped the earlier non- and semi-persistent categories, and the term circulative replaced persistent, and included both virus species that replicate in insect cells (propagative), and those that do not (non-propagative) (Table 1). This article will describe the characteristics of each of these transmission modes (schematized in Fig. 1), present the state-of-the-art of each process, and propose some future prospects by highlighting the most urgent question(s) to be investigated.
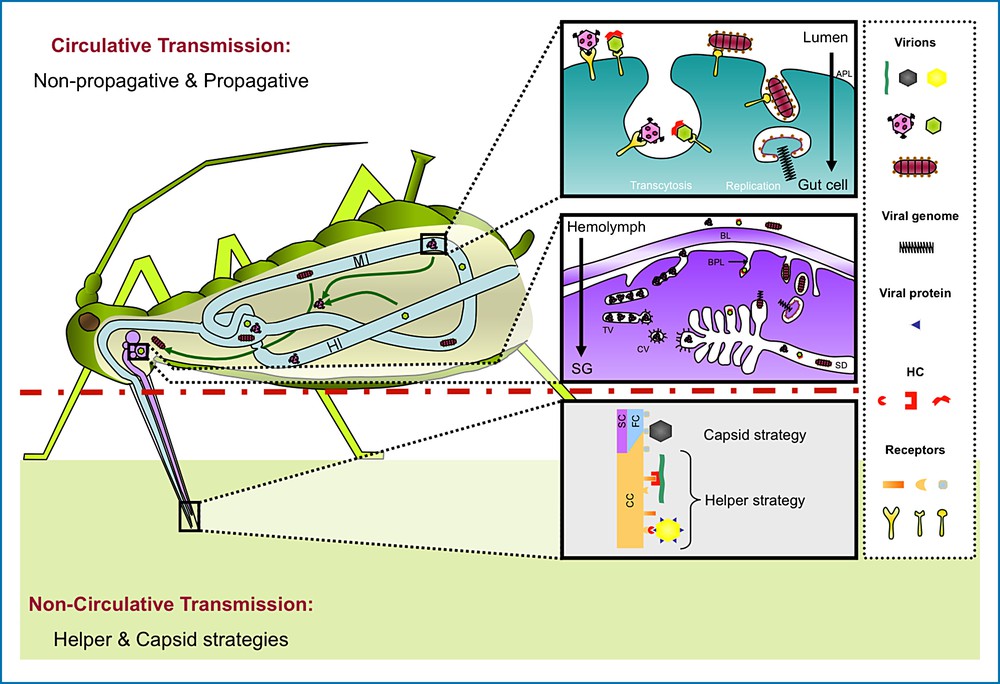
Models of virus retention and internalization in aphids. The sketch illustrates two modes of transmission (circulative and non-circulative) used by plant viruses that are transmitted by aphids. In non-circulative transmission, virus particles attach either directly to aphid receptors on the maxillary stylet cuticle (capsid strategy used by Cucumoviruses, black particles) or via an additional viral compound referred to as helper component (HC) (helper strategy used by Potyviruses, green particles, or by Caulimoviruses, yellow particles). In the case of CaMV (Caulimovirus), a non-structural protein (blue triangle) must be bound to the virion prior to its attachment to a receptor located in the common canal (CC) resulting from fusion between the food canal (FC) and the salivary canal (SC). In circulative transmission, virus internalization in aphid midgut (MI) and/or hindgut (HI) cells is mediated by attachment of virions to receptors located on the apical plasmalemma (APL). Virus uptake of Rhabdoviruses (red particles) in vesicles is followed by membrane fusion, release of viral genome into the cytoplasm and replication in gut cells (propagative). Conversely, virus internalization of luteovirids (pink particles) or Nanoviruses (green particles) is followed by virion transcytosis from one pole of the cell to the other without virus amplification (non-propagative). Virion release in the hemolymph is followed by subsequent internalization in salivary gland cells (SG) which, for Nanoviruses, is thought to rely on a HC. Virions must first cross the basal lamina (BL) surrounding the gland, then the basal plasmalemma (BPL), before being finally released in the salivary duct (SD). Luteovirid transport in salivary gland cells is mediated by tubular vesicles (TV) and clathrin-coated vesicles (CV).
2 Non-circulative transmission
In early studies on the previously called “non-persistent” transmission mode, decontamination by UV or chemical treatments of the extremity of the stylet bundle of aphids fed on infected plants correlated with suppression of subsequent transmission. The authors concluded that this type of transmission could be thought of as non-specific mechanical transmission, the stylets of the vector acting simply as contaminated “flying needles” [19,20]. Later, a new hypothesis based on virus uptake during sap ingestion and virus inoculation during putative regurgitation was proposed, which suggested that aphids act not as “flying needles” but rather as “flying syringes” [18] – the action of the vector still being considered as a non-specific sucking-up and -down phenomenon. Although it is now very clear that virus-aphid interactions are always extremely specific (see section 2.1 and 2.2), the prime discovery from these early studies was that retention occurs externally – on the cuticle lining the food and salivary canal in the aphid stylets – and that the virus does not penetrate and infect its vector. The category originally called “semi-persistent” was also grouped as non-circulative, because the corresponding viruses are also lost upon aphid moulting, demonstrating undeniably that the association with the vector is indeed external. Nevertheless, because of the extended time required for virus acquisition, retention and inoculation in the semi-persistent mode compared to the non-persistent mode [16] (Table 1), a possible localization of semi-persistently transmitted viruses on the cuticle lining the anterior gut of the insect was repeatedly proposed [21,22]. Even if this “foregut hypothesis” applies in the case of some semi-persistently transmitted viruses, we now have evidence that it does not apply to all viruses in this category. Indeed, Cauliflower mosaic virus (CaMV, family Caulimoviridae), the only non-circulative virus species that has been precisely located within its vector (see below), was found exclusively at the tip of the stylet [23]. At present, it remains unclear whether the distinction between non- and semi-persistent within the category of non-circulative transmission should be maintained, as it might not reflect any qualitative difference in the mechanisms of the virus-vector relationship. For this reason, Pirone and Blanc [24] proposed that the available molecular data on virus-vector interactions be used as the main criteria for defining the two distinct viral strategies found in non-circulative transmission: the “capsid strategy” and the “helper strategy” (Fig. 1). In the capsid strategy, the virus interacts directly with the vector via its coat protein (CP), whereas in the helper strategy the virus-vector interaction is mediated by an additional virus-encoded non-structural protein, generally designated “helper”.
2.1 Viral determinants of the interaction with aphid vectors
With the development of molecular biology and the availability of infectious viral clones for several virus species, mutagenesis and reverse genetics have allowed the precise determination of viral molecules controlling the specific interaction with aphid vectors. These viral determinants always include the viral capsid and, in various cases, additional non-structural virus-encoded proteins. The distinct molecular viral determinants involved in both the “capsid” and the “helper” strategies are discussed further in the following sections.
2.1.1 Viral determinants involved in the capsid strategy
The CP of viruses belonging to the genera Cucumovirus, Alfamovirus, Carlavirus and Crinivirus [25–27] have been demonstrated to be the only viral component determining virion retention within the aphid vector.
The development of artificial membrane feeding of aphids has been a major step forward in this field of research. This system allowed the artificial feeding of aphids, with virtually any solution, through stretched Parafilm® membranes. By providing purified virus particles in this solution, followed by transferring aphids onto healthy test plants, Megahed and Pirone developed the first in vitro aphid-transmission assay [25,28], which is still widely used today. These authors provided the first evidence of the capsid strategy by demonstrating that for some virus species, purified virus particles could be readily transmitted. While this simple experiment suggested that virions were the only required component essential for transmission, the involvement of additional factors still present in the purified virus suspension could not be totally excluded. This doubt was alleviated by a very elegant experiment in which biologically active virus particles of Cucumber mosaic virus (CMV, Cucumovirus) were reconstituted in vitro from viral genomic RNAs and purified CP [29]. Using different combinations of viral RNA and CP extracted from poorly or efficiently transmissible CMV isolates or different Cucumovirus species, two independent research groups [30,31] demonstrated that virus transmission efficiency was determined solely by the CP.
In addition to being the first virus for which this strategy was discovered and deciphered, CMV is also one of the most common pathogens in plants, and is reported to infect over 1000 plant species [32,33]. The group of Keith Perry resolved the structure of CMV particles at 3.2 Å resolution [34] and highlighted the presence of a loop in the CP sequence (the βH-βI loop corresponding to amino acids [AA] 191 to 198), which is located on the surface of the virus particle and is conserved among Cucumoviruses [35]. To assess its involvement in aphid transmissibility, the authors engineered 9 mutations within this loop in the strain Fny-CMV and showed that 8 mutants were severely deficient in transmissibility, despite no noticeable difference in virion stability. The βH-βI loop is strongly negatively charged, and residues 192 and 198 are involved in metal ion binding [34]. The authors hypothesized that the bound ion could influence charges at the surface of the virus particle that could be critical for virus-vector interaction. It was proposed that these charges, and consequently virus binding, might be affected in the mutants, although no direct experimental proof could be obtained.
Although some other viral species in a few other genera have been suggested to be aphid-transmitted according to the capsid strategy (Table 2), no molecular details are available.
Vectors and mode of transmission in different plant virus families (adapted from [155]).
Familya | Vectorsb | Mode of vector-transmissionc |
Betaflexiviridae genus Carlavirus | Aphid | Non circulative |
Bromoviridae genus Alfamovirus | Aphid | Non circulative capsid strategy |
Bromoviridae genus Cucumovirus | Aphid | Non circulative capsid strategy |
Caulimoviridae | Aphid, mealybug, leafhopper | Non circulative helper strategy |
Closteroviridae | Aphid, mealybug, whitefly, | Non circulative |
Comoviridae genus Fabavirus | Aphid | Non circulative |
Luteoviridae | Aphid | Circulative non propagative |
Nanoviridae: Nanovirus, Babuvirus | Aphid, planthopper | Circulative non propagative |
Potyviridae genus Macluravirus | Aphid | Non circulative |
Potyviridae genus Potyvirus | Aphid | Non circulative helper strategy |
Rhabdoviridae | Aphid, leafhopper, planthopper | Circulative propagative |
Sequiviridae | Aphid, leafhopper | Non circulative helper strategy |
a The families are broken down to the genus level when they contain genera with totally different vectors.
b This review being focused on aphids, only viral families and genera that contain member species transmitted by aphids are listed. Nevertheless, when these families and genera also contain member species transmitted by other related insects, these are simply mentioned for information.
c The helper or capsid strategies (see Fig. 1) are mentioned when demonstrated experimentally for at least one species of the viral taxon. When no complement is added to either “circulative” or “non-circulative”, it reflects the lack of further information.
2.1.2 Viral determinants involved in the Helper strategy
As in the capsid strategy, in vitro transmission assays have been crucial in identifying the existence of helper molecules, through the early observation that, in numerous viral species, virus particles lose their transmissibility upon purification [36,37]. Kassanis and Govier really pioneered the concept of the helper strategy in a series of remarkable reports in the 1960s and early 1970s, where they initiated identification of the missing factor that was obviously lost upon virus purification. Their approach was based on the recovery of transmissibility of a non-transmissible Potyvirus variant after co-infection with a transmissible isolate [38]. Similar complementation was obtained when aphids used for transmission of a non-transmissible isolate were first fed on a plant infected by a transmissible isolate [39,40]. The same authors further showed that this complementation was due to a compound extracted from plants infected by the transmissible isolate [37]. In summary, these complementary studies suggested strongly that a viral factor dispensable for the viral infection cycle was involved in aphid-mediated transmission of Potyvirus species. This factor was named “helper component” (HC) and a mode of action was proposed in which two independent interacting domains would link the CP on one side and the putative attachment sites within the insect on the other, a hypothesis later designated the “bridge hypothesis” [24].
A similar discovery was made for another unrelated virus, in the genus Caulimovirus. Using strictly equivalent approaches of complementation between naturally transmissible and non-transmissible isolates, Lung and Pirone [41,42] demonstrated that a typical HC was also involved in the transmission of CaMV. Later, the existence of HC was searched for in many other plant virus species, and found to represent the major molecular strategy for non-circulative transmission (Table 2). Nevertheless, viruses belonging to the genera Potyvirus and Caulimovirus remain undoubtedly the best characterized viruses producing a HC for their aphid transmission. The viral components involved in the transmissible complexes that are retained within the vector are now well defined and are described in detail below.
2.1.2.1 Potyviruses
According to the International Committee of Taxonomy of Viruses, the Potyvirus genus contains 125 definitive species and 88 tentative species [43], and is thus one of the most numerous genera among plant viruses. Potyviruses possess a filamentous flexuous particle that protects a single-stranded positive-sense RNA genome of about 10 kb with a covalently linked 5′-terminal viral protein (VPg) and a 3′-terminal polyadenylated tail [44]. The viral genome contains a single open reading frame (ORF) encoding a polyprotein that is cleaved into 10 functional products [45] by three viral-encoded proteases [46]. Numerous approaches, based mainly on protein purification, immunoprecipitation and transmission experiments [47–49], have been developed to demonstrate that the N-terminal part of the Potyvirus-encoded polyprotein contains the functional product that helps the aphid-mediated transmission of viral particles. This polypeptide is cleaved off from the polyprotein at its C-terminus by an autocatalytic protease activity and, accordingly, has been designated Helper Component-Protease (HC-Pro).
HC-Pro is a multifunctional protein (for a review, see [50]) involved in many steps of the Potyvirus infection process. As suggested for the first time by Thornbury et al. [51] and confirmed later by Wang and Pirone [52], Plisson et al. [53], and Ruiz-Ferrer et al. [54], the biologically active form of the HC-Pro protein is a homodimer. Protein-protein interactions are supported by regions located at both the N- and C-terminal parts of HC-Pro [54,55]. HC-Pro monomers are composed of two helix-rich domains located near the N- and C-terminal ends of the protein, linked together by a hinge. As described above, the first reported function for HC-Pro was associated with aphid-mediated transmission. Considered as a regulatory factor for viral transmission [56], the first direct proof that HC-Pro indeed acts according to the bridge hypothesis (see above, and Fig. 1) was obtained by using transmission electron microscopy [57]. On the one hand, the interaction between HC-Pro and the stylets of aphid vectors was shown to depend on the presence of a peptidyl domain, the four-residue motif “KITC” located at the N-terminal part of the HC-Pro sequence, which could interact with an unknown aphid receptor [58]. On the other hand, a second motif involved in virus transmission was described at the C-terminal end of the HC-Pro sequence. This “PTK” motif was demonstrated to be required for efficient interaction between HC-Pro and the “DAG” domain located at the C-terminus of the CP [59,60]. Altogether, these data confirmed the binding of HC-Pro to both the viral CP and a putative receptor within the aphid's stylets, forming a molecular bridge between the virus and the vector during the non-circulative transmission process.
In addition to its function in transmission, HC-Pro possesses numerous other well described properties and/or functional domains. From its N- to C-terminus, the HC-Pro protein contains a zinc-finger motif reported to be involved in synergistic effects with other viruses [61,62], nucleic acid binding domains [63], “IGN” and “CC”/“SC” peptidyl domains involved in the replication of viral RNA and systemic movement of virus in the plant, respectively [64,65], and a cysteine protease-like activity supported by the presence of cysteine and histidine residues in the active site [66]. HC-Pro seems also to be involved in virus movement through plasmodesmata [67] and in access to plant vasculature [68] for systemic infection. Finally, the role of HC-Pro as a suppressor of gene silencing [69–71] and its involvement in the host necrosis response to viral infection [72,73] have recently been described. The fact that the different functional domains of HC-Pro overlap suggests a highly orchestrated regulation of its activity. Whether the localization, conformation, post-translational modification and thus biological properties of HC-Pro change throughout the infection cycle has not yet been investigated. It nevertheless represents a question of prime importance in understanding how this central protein orchestrates viral replication, host cell defense escape, cell-to-cell and systemic movement, as well as virus transmission.
2.1.2.2 Caulimoviruses
CaMV is the type member of the plant virus family Caulimoviridae. This family is grouped together with Hepadnaviruses into the Pararetrovirus group due to its mode of replication via reverse transcription of a pre-genomic RNA intermediate [74,75]. The CaMV genome is a double-stranded circular DNA of approximately 8000 base pairs, comprising seven major open reading frames (ORF), six of which have products (designated P1–P6) that have clearly identified biological functions (for review, see [75]). As mentioned above, CaMV was demonstrated to use a HC molecule to control its aphid transmission [41,42], and is now the plant virus species for which the interaction with the vector is best characterized.
The sequencing of two naturally occurring non-transmissible isolates [76,77], as well as the analysis of engineered mutants [78–80], consistently suggested that gene II encodes the HC molecule, which is required specifically and solely for aphid-transmission. Indeed, gene II encodes a protein of 18 kDa, hereafter designated P2 [80], that is totally dispensable for CaMV infectivity [77]. The fact that P2 is not involved in other functions of the virus cycle is a striking difference from the helper strategy of Potyviruses, where HC-Pro harbors several other vital functions. The successful expression of a biologically active P2 in the Baculovirus/insect cell system (BCI) [81] helped considerably in studying the various biological and biochemical properties of this protein [82], as well as the properties of the transmissible complex. For example, aphids fed with this artificially-produced P2 could complement the transmission of virions acquired from crude extracts of a plant infected with a non-transmissible strain, but not from purified virus preparations [81], suggesting the involvement of a putative additional unknown factor that is lost upon virus purification. This additional factor was later identified as the viral protein P3 [83], which is in fact very intimately associated with the CP in the mature virus particles [84,85] and mediates P2 attachment to virions [83].
The fact that another viral protein, in addition to the virion and the HC, constitutes the transmissible complex still fits within the predicted general mode of action of HC known as the bridge hypothesis (see Fig. 1). Indeed, the attachment of CaMV to the aphid is mediated by P2, the N-terminal domain of which specifically recognizes a cuticular protein receptor located at the extreme tip of the maxillary stylets [23,86], whereas the C-terminal α-helix binds, via predicted coiled-coil structures, to the ectodomain of the P3 decorating the virions [82–85]. Prior to acquisition by aphids, an electron-lucent inclusion body containing co-aggregated P2 and P3 with very few scattered virus particles appears within plant cells upon infection [87–89]. Since this inclusion body is specifically and solely involved in vector-mediated transmission [90], it is commonly designated the transmission body (TB). A two-step pattern for the acquisition of CaMV by its aphid vector seems to be controlled by the TB and has been described in detail [87]. When an aphid punctures an infected cell, the TB is disrupted and the released P2 attaches to its receptor within the aphid stylets, whereas P3 is solubilized in a free conformation that is not retained together with P2. Subsequently, the P2-loaded aphid will acquire P3-virion complexes from the viral factories or from the phloem, due to the very high affinity between P2 and virion-bound P3 [87,91]. Although this mechanism of sequential acquisition seems to favor cooperation between related CaMV viral genomes during transmission, through a phenomenon called HC-transcomplementation [24,92], the molecular mechanisms and the adaptive benefits underlying this two-step acquisition pattern remain poorly understood.
2.2 Counterpart receptor in aphids
Despite the fact that non-circulative transmission is the most widely adopted transmission mode in plant viruses, and despite the extensive knowledge of the viral determinants involved in virus-vector interaction (see above), putative binding sites for viral components in the insect vector have been characterized only very recently. A distinguishing feature of non-circulative transmission is that several virus species can be transmitted by the same vector and, conversely, several vector species can transmit the same virus. Although some degree of virus-vector specificity exists in non-circulative transmission [86,93], this specificity is often so broad that the existence of viral receptors has long remained disputed. This question is of major importance, since numerous non-circulative viruses may use the same vector attachment sites, and identification of putative receptor molecules could lead to new strategies to combat the spread of several viruses.
Most research attempting to localize viral receptors has focused on aphid stylets and details of the anatomy of these specialized mouthparts are presented here (Fig. 2). Aphids are insects with piercing-sucking mouthparts whose major component is the stylet bundle. This bundle comprises two external mandibular stylets that surround two inner maxillary stylets, all transformed into four long needle-like cuticular structures, several hundred microns in length (typically 400–700 μm). The mandibular stylets have a penetrating function, guiding the nested maxillary stylets towards deeper tissues [94,95]. Maxillary stylets are sharply pointed at the distal extremity, and they display a very complex inner architecture. The whole length of the inner face of both stylets is sculpted by longitudinal ridges and grooves, interlocking in a “zip-lock” manner due to their complementary structure. Once interlocked, this complex architecture leaves empty ducts, corresponding to one large food canal, and one small salivary canal (Forbes, [94] #2520). Food and salivary canals are thus separated along the length of the stylets, except for the distal last few microns, where they fuse into a single canal [96,97] known as the common duct. As illustrated in Fig. 2A, the inner faces of the two maxillary stylets are asymmetrical – the salivary canal is present in only one of the two stylets, the opposite one closing it with a ridge. When observed under transmission (TEM) or scanning electron microscopy (SEM), maxillary stylets from several aphid species [98] varied in size but showed a very conserved anatomy (Fig. 2B).
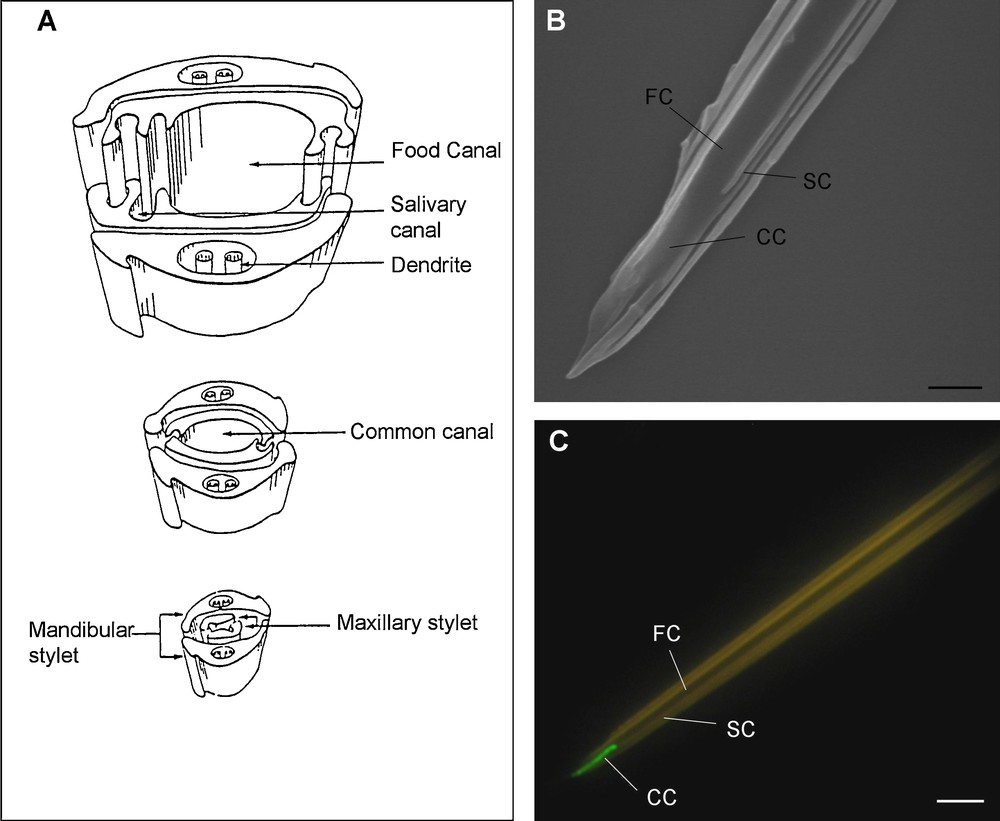
The receptor of a non-circulative virus (CaMV) is located in the common canal of aphid maxillary stylets. A. Schematic representation of the distal extremity of the stylet bundle of Myzus persicae (from Taylor and Robertson [154]). The stylet bundle is composed of four long needle-like chitin appendices, the two external mandibular stylets surrounding the two inner maxillary stylets. The particular features of the maxillary stylets, with their interlocking ridges and grooves, shape two elongated canals – the food and the salivary canals (top drawing) – that fuse close to the distal tip to form the common canal (middle and bottom drawings). B. An isolated maxillary stylet tip of A. pisum observed using scanning electron microscopy (picture courtesy of C. Cazevieille). C. In vitro interaction assay between dissected stylets of A. pisum and the P2 protein (HC) of CaMV fused to green fluorescent protein (from Uzest et al. [23]). Epifluorescence microscopy reveals binding of P2-GFP exclusively at the tip of the maxillary stylets (green fluorescence). Food canal (FC), salivary canal (SC) and common canal (CC) are indicated in B and C. Bars represent 1 and 5 μm in B and C, respectively.
Previous attempts to localize attachment sites of non-circulative virus species in various vectors defined only roughly the anatomical regions of the anterior alimentary tract involved in virus retention. The best documented example is certainly that of the genus Potyvirus, where a combination of light and electron microscopy revealed transmissible complexes of Tobacco etch virus and Tobacco vein mottling virus distributed apparently randomly along the maxillary food canal, sometimes throughout its entire length, sometimes limited to its distal and/or central portions [57,99,100]. Observation of non-circulative viruses adsorbed along the stylets and/or the anterior part of the foregut does not necessarily mean that these virions will be released and inoculated to plants. The most commonly accepted scenario for this inoculation step, which has experimental support for a Cucumovirus and a Potyvirus, is that excretion of saliva would dislodge viruses [101,102]. It is important to realize that this scenario implies that viruses are retained in the common food/salivary duct located at the very distal extremity of the maxillary stylets (Fig. 2 A and B). Indeed, only virus particles retained at this location during streaming up of contaminated sap could be efficiently washed out by ejected saliva.
A novel in vitro interaction assay on dissected individual stylets has been developed recently [23]. Incubation of dissected stylets with CaMV P2 fused to Green Fluorescent Protein (GFP) unequivocally demonstrated that the CaMV receptor is located exclusively in the common canal of the maxillary stylets of aphid vectors (Fig. 2C). The correlation between retention of the virus in this area and its successful transmission was verified by several approaches, thus confirming that the common duct is indeed used, at least by CaMV, for interaction with the vector. This simple interaction-based assay was further used to analyze the action of several chemical and enzymatic pretreatments on the integrity of the receptor molecules, an analysis that has long remained impossible on live aphids, which were poisoned by such treatments. Among several chemicals destroying lipids, sugars and proteins, only protease pretreatment abolished the P2 binding to dissected stylets, suggesting strongly that the receptor of CaMV is a non-glycosylated protein tightly associated to the chitin matrix [23]. These results are of prime importance because they represent the first demonstration of the actual existence of receptors of non-circulative viruses within vectors. Beyond CaMV, this approach certainly paves the way for future investigations, locating and characterizing analogous receptors of other non-circulative species.
3 Circulative transmission
The term “circulative” was introduced by Harris [18] and applies to viruses undergoing part of their life cycle within the body of the vector. In this type of relationship, the ingested virus traverses the gut epithelium at the midgut or hindgut level (for example, see [103,104]) and is released into the hemolymph. From there, some virus species can reach different organs, including reproductive organs, which will further favor their transovarial transmission to the vector offspring. From the hemolymph, the viruses must also enter the salivary glands to be transferred to the saliva, from which they are inoculated to healthy hosts to initiate new infections. The time required for the virus to complete this cycle is designated the “incubation period” (for animal viruses) or the “latent period” (for plant viruses) and varies from several hours to several days depending on the route followed within the vector, as well as on physical factors such as temperature. In most cases, once the virus is ingested, it will persist in the vector for its whole lifespan.
Obviously, circulative transmission implies that the virus goes through a number of physical barriers, which ultimately condition the specificity of successful virus–vector interactions. First, viruses have to pass from the gut lumen to the hemocoel through the gut epithelium. Pioneering work demonstrating the existence of this gut barrier was conducted by Storey [105], who observed that non-vector leafhopper species could transmit Maize streak virus (MSV, Geminiviridae) when their midgut was punctured with a needle after they had fed on an infected plant. Since then, an enormous amount of work involving intrathoracic injection of viruliferous solutions into vectors (including aphids) has confirmed that this barrier can stop numerous viruses. Second, the virus must reach the salivary glands, and this transfer can follow different routes (diffusion within the hemolymph for luteovirids, or infecting specific organs such as the nervous system for rhabdoviruses, see below) where incompatible interactions can be observed. Third, the final passage through the salivary glands and into the saliva may also be stopped by specific barriers. The circulative transmission mode is divided into two subcategories depending on whether the virus actually replicates during this journey within the body of its vector (circulative propagative transmission), or not (circulative non-propagative transmission).
3.1 Circulative propagative transmission
Circulative propagative transmission concerns predominantly vector-transmitted vertebrate-infecting viruses (arboviruses), for which it is a major mode of transmission, but it is observed only infrequently for plant viruses. Nevertheless, some bullet-shaped plant viruses in the Rhabdoviridae family (Cytorhabdovirus and Nucleorhabdovirus) exploit this mode of transmission for their propagation, some by aphids and others by related insects like leafhoppers and planthoppers [106]. Sylvester and Richardson [107] reported at least 11 plant rhabdoviruses to be transmitted by aphids. Aphid-transmitted rhabdoviruses display a high degree of vector specificity, and more than half of the viruses have only one reported vector species [108]. Literature concerning rhabdovirus transmission by aphids is relatively limited; therefore, this section focuses mainly on data coming from leafhoppers and planthoppers transmitting rhabdoviruses – the relationship between virus and vectors being considered similar in these cases. The two rhabdovirus genera, Cytorhabdovirus and Nucleorhabdovirus, were defined according to their ability to bud from cytoplasmic membranes or from nuclear membranes, respectively [109]. Plant rhabdoviruses very rarely induce disease in their vector, where they multiply at a low rate, in contrast to the damaging effect they have on their plant host, where they replicate to high levels. This suggests that these viruses are better adapted to their insect host, which may have been the primary host of an ancestral rhabdovirus [110]. This hypothesis is reinforced by the fact that some rhabdoviruses are transmitted to the progeny of the vector by transovarial transmission, whereas no seed or pollen transmission has so far been reported for these viruses. In this regard, the plant host can be seen as a temporary alternative host, in which the virus multiplies at high titer to subsequently infect more invertebrate hosts.
These enveloped viruses are acquired by the insect when feeding on an infected plant, and require a latent period (a few days to a few weeks) during which the virus replicates in insect cells before being inoculated in a plant host during a subsequent feeding [107,109,111]. As indicated above, the virus route in the insect is punctuated by several barriers. The first is located at the gut level, where virions enter gut cells by endocytosis after recognition by specific receptors lining the apical plasmalemma. After replication in gut cells, virus particles escape by crossing the basal plasmalemma, which represents the second barrier. Once released in the hemolymph, virions will gain access to several different organs, in particular the reproductive organs, which provides a vertical transmission route from an infected female to its progeny, albeit at a very low rate [107,110,112]. For viruses transmitted transovarially, additional barriers will have to be crossed, in particular the ovarian and ovariole sheaths, as well as the follicular epithelium. The other organs accessible to virions are the brain, nerve ganglia, epidermis, fat and conductive tissues, retina, muscles and tracheae [107,113]. The hemolymph also plays a role in virus circulation, because virions must survive in this environment and escape the aphid immune system. The last important barrier to be overcome is the principal salivary gland cells. The virus must first enter these cells through the basal lamina and then through the plasmalemma. Virions bud through the plasma membrane and accumulate in intercellular spaces that ultimately connect to the salivary ducts, where the virus can move to a new plant with saliva [113]. Beside this hemolymph route, which shares similarities with the circulative non-propagative mode (see below), rhabdoviruses may also reach the principal salivary gland cells via the central nervous system [114]. This latter transport route has been suspected in the case of Maize mosaic virus (MMV) in its planthopper vector but at this time, there is no evidence that such transport also occurs in the case of aphid-transmitted rhabdoviruses. Infection of nervous tissue could affect insect behavior, in particular salivation, which could have an impact on virus transmission. Therefore, gut cells and hemolymph, as well as salivary gland cells, have been reported to act as physical barriers for rhabdoviruses progression in the aphid, and the inability of an insect to transmit the virus could be due to a failure of the virus to enter, replicate and escape from each of these locations [57].
3.1.1 Interactions between viruses and vectors
Entry of enveloped viruses into host cells always involves a virus recognition step, followed by membrane fusion. rhabdoviruses enter the cells via the endocytotic pathway, and fusion occurs with the endosomal compartment [115]. The trimeric rhabdovirus glycoprotein (G protein) protrudes from the virion's lipid envelope and plays a major role during viral entry: it is responsible for binding the virus to target cells and for fusion between viral and endosomal membranes. Fusion with the endosome membrane is triggered by a conformational change of the G protein induced by the low pH of this cellular compartment. The G protein is a type I integral membrane protein comprising a large ectodomain, a transmembrane sequence, and a small C-terminus inside the viral envelope. Specific interactions between G protein and cell surface components have been demonstrated for vertebrate rhabdoviruses [109,116], but virus receptors in insect cells have never been identified. Involvement of G protein in the recognition or attachment to leafhopper cell surface receptors has been deduced from entry blocking experiments using specific antibodies directed against this protein [117]. The function of G protein in the aphid gut endocytosis process is still hypothetical, and is based on gene function conservation among members of the Rhabdoviridae family. Although there is little sequence homology between G proteins, structural features of these proteins are conserved among plant and vertebrate rhabdoviruses [118,119], and are thought to be involved in receptor recognition. Since rhabdoviruses infect a series of different tissues in the insect, one can suppose that virus receptors may be either ubiquitous or tissue specific. Protein exchange between the G protein of a vertebrate rhabdovirus and the envelope protein from Human Immunodeficiency Virus-1 leads to cell specificity of HIV-1, suggesting that the G protein, in addition to promoting virus entry, also governs cell specificity [120]. The matrix protein (M protein) of rhabdoviruses, which controls virus assembly, is another viral protein involved in virion release from cytoplasmic membranes [121].
3.2 Circulative non-propagative transmission
In contrast to circulative propagative transmission, which is widely used by vertebrate arboviruses for their dissemination, circulative non-propagative transmission seems to be restricted to plant viruses. Both modes of transmission, however, share similarities because in both mechanisms the virions cross the gut epithelium to be released into the hemolymph, and then to the salivary gland cells to be secreted in the salivary ducts. The specificity of the non-propagative mode is that, except for gut and accessory salivary gland (ASG) cells, no other cellular types will contain virions, and no replication will ever occur in any of the virion-harboring cells. Plant viruses known or believed to exploit this mechanism of aphid transmission are members of the Nanoviridae (referred to as nanoviruses, [122]) and Luteoviridae (referred to as luteovirids) families. These viruses are phloem-limited, which makes phloem-feeding aphids suitable vectors. Nanoviruses consist of ∼6–8 small icosahedral particles that each contains a circular single-stranded DNA molecule [123]. Luteovirids also have icosahedral particles but their genome is a single-stranded monopartite RNA. Although they share a similar mode of transmission, luteovirids and nanoviruses rely on different viral determinants for virus internalization in the vector: for luteovirids the capsid protein is required for efficient virus transport, whereas an additional HC is suspected to be required for nanovirus transmission [124]. This HC could participate in the transport of virions across the hemocoel-ASG interface of the aphid. This HC has not been identified so far, which brings into question whether it really exists.
Aphid transmission of luteovirids, which is by far better characterized than that of nanoviruses, involves a large number and diversity of proteins from various origins (virus, insect, bacteria, and plant). TEM observations have been applied extensively to follow the route of luteovirids in their vector [125,126]. Intestinal endocytosis and exocytosis of luteovirids rely on a sequential mechanism, starting with clathrin-mediated endocytosis, followed by transport of virions enclosed in vesicles from the apical to the basal pole of the cell, and ending with fusion of virus-containing vesicles to the basal plasmalemma. Virions are always enclosed in vesicles, which suggests that, except for membrane components, no direct contact with aphid compounds occurs. Transcriptome analysis of the expression of intestinal genes after luteovirid acquisition revealed that only 1.9% of genes were differentially expressed in the presence of the virus [127]. Even if this analysis covered only about 20% of the recently sequenced genome of the aphid Acyrthosiphon pisum (International Aphid Genomics Consortium, 2009), the limited levels of down- (maximum 3.45-fold) and up-regulation (maximum 1.37-fold) suggest that the virus hijacks a constitutive endocytosis-exocytosis mechanism without greatly disturbing cell metabolism. The process of virus transport through ASG cells operates in a similar manner but in the reverse direction. While, since most of the viruses can be internalized in both vector and non-vector species, the gut epithelium is not very specific for uptake of luteovirids, the basal lamina and basal plasmalemma surrounding ASG cells have been shown to function as selective barriers to transmission [125].
The high vector specificity of luteovirid transmission [128] suggests that viral determinants located on the surface of the particle determine its recognition by aphid receptors, uptake in the cells and release into the plant. In addition to molecular motifs borne on the surface of viral particles, these different events involve virus-specific receptors located on the surface of the insect intestinal and ASG cells. Once internalized in the aphid body, the persistence of virions in the hemolymph is believed to rely on interactions with a protein synthesized by a bacterium hosted as an endosymbiont by the aphid. Very recently, it was observed that plant proteins located in sieve tubes are able to bind purified luteovirids and could stimulate virus transmission by the aphid when added in an artificial diet [129], suggesting that some phloem proteins could potentially promote virus acquisition by aphids, although the precise mechanism by which this would operate is still unknown.
3.2.1 Viral determinants involved in circulative non-propagative transmission
The icosahedric shell of luteovirids is constituted by the association of 180 monomers of the CP and a few copies of a readthrough (RT) protein that contains the CP at its N-terminus and whose C-terminus is exposed at the surface of the particles. These two structural proteins, CP and RT, play an important role during host infection because both are required for efficient virus transport in the plant [130–133]. The first report of the involvement of the CP in the transmission process resulted from complementation experiments from mixed infected plants resulting in heterologous encapsidation, which modified the vector specificity of some luteovirids [134]. Extensive additional studies, mostly using non- or poorly-transmissible natural or artificial variants, have shown that the RT protein is required for transmission [130,132,133,135,136] and governs transmission specificity [104]. While this protein is not strictly required for intestinal transport, its presence in the virion seems to be a requirement for virus transcytosis through ASG cells [132,137]. However, experiments with non-infectious virus-like particles produced in a baculovirus/insect cell system suggested that the CP alone could bear the viral determinant governing virus transcytosis in aphids [138]. To date, several residues on the luteovirid CP and RT sequences have been proposed to specifically interact with a counterpart in the aphid [131,139,140], but no consensus motif for luteovirid transmission could be deduced from these studies. To alleviate some uncertainties regarding the domains of the structural proteins required for luteovirid transmission by aphids, a new challenge for the future could be reconstitution of the three-dimensional structure of these virions to highlight the precise domains exposed on the surface of the particles.
3.2.2 Counterpart receptor in aphid
As described above, internalization of luteovirids by a clathrin-mediated endocytosis process [125,126] relies on the presence of aphid-specific receptors lining the gut and the ASG epithelia. Once enclosed in a clathrin-coated vesicle, virions will benefit from additional cellular proteins constitutively dedicated to nutrient uptake [141].
So far, luteovirid receptors have not been identified but several aphid proteins exhibiting the ability to bind purified luteovirid particles in vitro have been reported [142–144]. They involve several proteins extracted from whole aphids (Myzus persicae), which have the capacity to bind Potato leafroll virus (PLRV) or Beet western yellows virus (now designated Turnip yellows virus, TuYV), and other proteins extracted from the heads of Sitobion avenae, which display the ability to bind purified Barley yellow dwarf virus-MAV. Not all these proteins were identified by mass spectrometry and their involvement in the aphid transmission process was reported for only two of them. The first is symbionin, a homolog of Escherichia coli GroEL [142], which is produced by the aphid endosymbiont, Buchnera spp., and can bind the particles of several luteovirids [145,146], in particular the minor capsid protein [145]. An antibiotic treatment of aphids eliminating endosymbionts inhibits the ability of the treated aphids to transmit PLRV [142]. This result suggests that symbionin, by interacting with the luteovirid particle, could protect virions from degradation by the insect immune system. However, even if necessary for efficient aphid transmission of luteovirids, symbionin is not a factor determining specificity since its presence in aphids does not correlate with their capacity to transmit luteovirids [145,147]. Aphid transmission specificity is more probably attributed to receptors in the gut and the ASG.
The second aphid protein suspected to be involved in the transmission process is SaM50, which is localized in the ASG cells [144]. Aphid acquisition of antibodies directed against this protein, together with virions, greatly reduced virus transmissibility [148]. The other aphid proteins exhibiting virus-binding capacity were an aphid cuticular protein whose role in the transmission of luteovirids is unclear; actin, which could act in clathrin-mediated internalization or intracellular transport of virion-enclosing vesicles; a receptor for activated C kinase (Rack); and glyceraldehyde-3-phosphate dehydrogenase (GAPDH3) [143]. Aphid sequences corresponding to these last two proteins have been mapped recently on the A. pisum genome [141]. These proteins are probably not the true virus receptors, but may function as intracellular signals in the endocytosis pathway [126].
An elegant recent study by Yang et al. [149] identified four aphid proteins associated with the ability of Schizaphis graminum to transmit Cereal yellow dwarf virus-RPV. All four proteins displayed the ability to bind purified virus, and two were identified as luciferase- and cyclophilin-like proteins, whose function could be linked to the endocytosis pathway. This latter study, based on proteome comparisons of vector and non-vector genotypes, also supported the hypothesis that luteovirid transmission is controlled genetically and regulated largely by one major gene, or a set of tightly linked genes, although minor genes can also affect transmission of each virus species or isolates independently [149–152]. Moreover, the multiple genes involved in transmission are either specific for the gut or the salivary gland cells [150]. As already mentioned in this review, transmission of luteovirids is highly specific and each virus species is generally transmitted efficiently by one or two aphid species [128]. Nevertheless, transmission by a given aphid species can be shared between several luteovirids, as is the case for M. persicae, which can transmit at least 7 of the 20 viruses listed in the Luteoviridae family [128]. Electron microscopy observations, which showed that gut tropism can vary between luteovirids in similar aphid species, together with genetic analyses, suggest that transmission of closely related virus species can be controlled by different genes within the same aphid species or genotype [151,153].
4 Concluding remarks
The present review is focused on the mechanisms of interaction between aphids and the numerous viruses they can transmit. This field of research has seen tremendous progress within the last few decades in our understanding of the routes followed by viruses within their respective vectors, and of the panel of genes and protein motifs that viruses devote to this process. Clearly, even in the best characterized examples such as potyviruses, caulimoviruses, rhabdoviruses and luteovirids, many details are still missing, as noted in the text. Moreover, for other aphid-transmitted plant virus species, while the virus-vector interaction is usually classified within one of the major categories, this is often based on fragmentary information and seldom on molecular details.
Nevertheless, the remaining huge black box in this field is the identity of the specific receptors of viruses borne by the stylets, foregut, midgut or hindgut of the insect vectors. This problem is equally acute regardless of the vector species involved (aphids, other insects, nematodes), and solving it would certainly also lead to major practical applications. Indeed, the present and future needs for agricultural production with high yield and quality, the recurrent problems associated with emerging disease agents, the intense global exchange of plant material (and the associated accidental concurrent exchange of insect or other vector species) will continue to dramatically enhance the impact of plant diseases caused by viruses and other pathogens. At present, strategies for controlling viruses take two main forms:
- • the wide use of resistance genes associated with breeding programs;
- • intense and repeated attempts to control vector populations, most frequently through the use of pesticides.
Since the first strategy can be confronted with resistance-breaking by new viral variants, and the second has proved inefficient in totally suppressing vectors and thus is unable to prevent viral outbreaks, there is a need for new and innovative strategies in the near future.
The great expectation from research on virus receptors within their vectors, beyond their obvious fundamental interest, is that their identification would allow the development of competing molecules that would reduce or inhibit plant-to-plant transmission of the corresponding viruses. Furthermore, since several viral species within the same family are responsible for enormous agricultural problems (e.g., potyviruses and luteovirids), one such competing molecule, developed to block the receptors of one virus species, may also be efficient against related viruses. In that sense, virus receptors within insects clearly represent ideal targets from which to develop alternative and more environmentally friendly strategies for controlling viral spread.