1 Introduction
Evolution enlightens all fields of biology through a single unifying principle: the descent with modification of individual organisms, making species varying through time, from generations to generations. As a consequence of the fine-tuning of genetic, developmental, physiological, and ecological processes enabling every organism to live in a given environment, conspecific individuals share common biological characteristics (inclusive of population polymorphism) that make up the functional identity and historical singularity of their community of reproduction. In spite of its still actively debated ontological and philosophical status, the biological species as a set of naturally and spontaneously interbreeding individuals that evolve in a unified way, thus appears as a fundamental unit of biological evolution and taxonomic classification [1,2].
From such an evolutionary perspective, biodiversity–the variety of life on Earth–can be viewed as the sum of all genetic, organismal and ecological variations (both compositional and functional) observed within and between species that coexist at a given time and place [3,4]. In this way, biodiversity goes far beyond the notions of taxonomic richness or abundance, as it also encapsulates genetic variability, morphological disparity, phylogenetic relatedness, trophic web structuring, etc. From genes to biomes and biogeographic kingdoms through individuals, (meta-) populations, species and ecosystems, such a multifaceted biodiversity is simultaneously the natural selection-driven cause and speciation-mediated consequence of evolution.
Whatever the natural group and environmental context under study, linking biodiversity with evolution makes worthy of attention two fundamental facts that emerge from the observation of ancient and extant life:
- – continuously, new species originate, and others become extinct–the average duration of species as evidenced by the fossil record is 1–10 m.y. in order of magnitude, making the vast majority of genetic, organismal and ecological combinations that have existed on Earth already extinct–the few (tens of) millions of extant species are likely to represent ∼1‰ or less of all species that evolved on Earth;
- – most of the identified fossil and living species show rather restricted geographic ranges corresponding to specific biogeographic and environmental conditions associated with the evolutionary history and ecophysiological characteristics (adaptations) of each species.
As a direct consequence, in a physically heterogeneous and ever-changing world, spatial and temporal fluctuations of biodiversity are the rule since the origin of life on Earth, thus legitimating two main questions:
- – How does biodiversity vary through time?
- – How does biodiversity vary through space?
Over the last several decades, these two questions have been addressed rather separately, the first one lying at the core of the Macroevolutionary research program [5–10], whereas the second one has more recently provided the basis for Macroecology, a research field concerned with the large-scale distribution and functional organization of life on Earth [11–15]. Nevertheless, while focusing on different topics (the analysis of global patterns and processes of speciation and extinction through time, and the descriptive and inferential spatial modeling of the ecogeographical distribution of biodiversity, respectively), these two questions are not independent: while in practice time and space are treated distinctly, they are functionally related dimensions of biological evolution (Fig. 1).
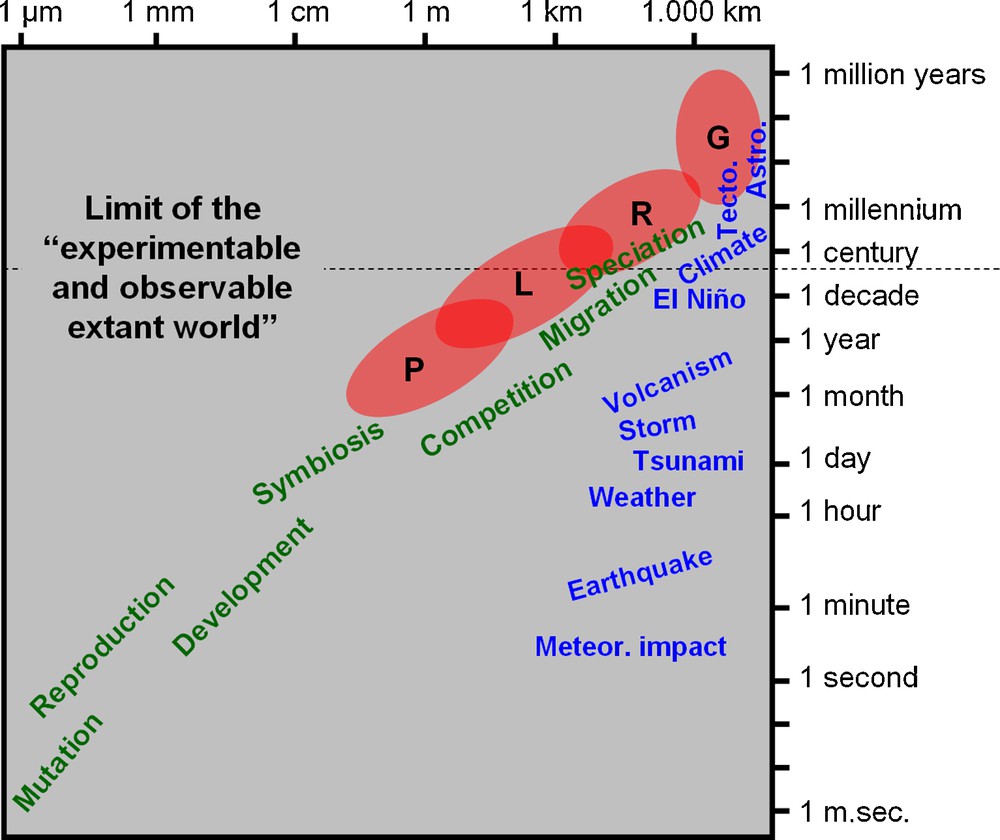
Spatiotemporal scaling of selected physical/geological (in blue) and biological (in green) events (modified from [16]). The red ellipses roughly delimitate the four main spatiotemporal domains of descriptive biodiversity: P: point diversity; L: local community (α-) diversity; R: regional metacommunity (γ-)diversity; G: global diversity. The “limit of the ‘experimentable and observable extant world”’ is an empirical and pragmatic frontier in the scientific study of the Universe. Meteor. impact: meteoritic impact; Tecto.: plate tectonic activity; Astro.: astronomical cycles.
Such a spatiotemporal integration of biodiversity dynamics raises an operational difficulty: from the metapopulation and metacommunity regional level upward, the geographic and chronologic scales at which biodiversity changes (from hundreds to millions of square kilometers and years) are definitely inaccessible to experimental neontology. Instead, they require a deep-time historical perspective and hypothesis-testing approach based on the description and modeling of palaeobiological data coupled with ex silico simulation analyses [14,17–29]. Because the deep-time scale of species origination, evolution and extinction, is de facto the “maximum time scale” of the assembly, evolution and dismantling of local communities and regional metacommunities, a Macroecology not integrating the deep-time dimension offered by palaeontological data would be a myopic science condemned to never entirely and clearly see the object–definitely too large–of its study.
Hereafter we emphasize some aspects of this ongoing integration, highlighting already acquired results and suggesting future research opportunities.
2 A preliminary digression about the depth of evolutionary times
One of the main conceptual difficulties that need to be properly addressed when studying deep-time evolutionary dynamics and processes is to fully take into account the huge depth of geological times over which biological evolution occurred. Geological ages, expressed in millions to billions of years, are finite quantities that largely exceed the human biological and cultural time frame. Only analogies may be helpful. For instance, if one meter represents 2000 years (i.e., 1 year = 0.5 mm, making a human life 3–5 cm-long), then the formation of the Earth (4.54 Ga) is 2270 km away, the beginning of the Phanerozoic Eon (542 Ma) is 271 km away, the beginning of the Cenozoic Era (65.5 Ma) is 32.75 km away, and the beginning of the Quaternary Period (2.588 Ma) is 1294 m away [30].
All along this geological time frame, millions of fossils and biological events are directly or indirectly dated, from the still debated oldest signs of life on Earth (∼3.5 Ga; [31]) to the extinction of the Tasmanian tiger (1936 A.D.), through, e.g., the oldest known stromatolites (3.4 Ga), eukaryotic cells (?3.2–2.1 Ga; [32]), multicellular organisms (2.1 Ga; [33]), vertebrates (530 Ma), terrestrial plants (465 Ma), tetrapods (395 Ma), mammals (215 Ma) and birds (145 Ma). Many of these fossils provide invaluable data for calibrating the tree of life [34]. However, along this immense calendar of the history of life, only the most recent part–the Phanerozoic Eon, corresponding to the last 12% of Earth history–yielded enough palaeontological information to permit the fossil-based study of biodiversity changes through time. Hence, most of the deep-time macroevolutionary and macroecological studies achieved so far have been based on Phanerozoic data (but see [35] for a noteworthy counter-example). This obviously does not mean that no spatiotemporal variation in biodiversity occurred during pre-Phanerozoic times.
For instance, it is very likely that the Snowball/“Slushball” Earth events recorded during the early Paleoproterozoic (2.4–2.2 Ga; [36]) and middle Neoproterozoic Eras (750–580 Ma; [37,38]) profoundly impacted organisms living at those times, but it is currently not known precisely how and to what extent such global glaciations actually affected life [39–42]. Incidentally, it is worth noting here that based on available evidence: (i) the first eukaryotic multicellular organisms followed the early Paleoproterozoic Great Oxidation Event and subsequent Makganyene global glaciation [33,43,44]; and (ii) the evolutionary radiation of multicellular life on Earth, beginning with the onset of the Ediacara biota (575 Ma; [45]) and culminating with the “Cambrian explosion” (530–520 Ma; [46,47]), began just after the end of the last major Neoproterozoic glaciation event (the Gaskiers glaciation, 580 Ma; [48–50]).
3 Phanerozoic diversity changes: from patterns to processes, and vice versa
Over the last 50 years, the macroevolutionary study of Phanerozoic biodiversity (or part of it) at large taxonomic, spatial and temporal scales has led to major advances in the knowledge of global patterns of diversity dynamics [5,6,51–53]. Based on extensive compilations of the fossil record such as Sepkoski's Marine Genus Database [54], the Fossil Record 2 [55] or, more recently, the Paleobiology Database collaborative project, most works focused on taxonomic origination, extinction and turnover dynamics, searching for large-scale trends and models, and discussing potential intrinsic and extrinsic biases and drivers of the observed variations (e.g., [5,51,53,56–72]).
Introducing a deep-time macroecological perspective into such a macroevolutionary framework raises new theoretical and practical questions that have not yet all been solved. Below we briefly discuss selected case studies illustrating some aspects of a macroecological/evolutionary integrated approach.
3.1 Recovery dynamics in the aftermath of major extinctions: insights from an inter-regional perspective
Mass extinctions played a fundamental role in Phanerozoic evolution and overall diversity dynamics [73–81]. By recognizing up to 61 such more or less dramatic global events in the fossil record, five of them leading to the estimated massive disappearance of more than ∼2/3 of the species existing at that time, biotic crises became a standard tool for large-scale biostratigraphy and correlation purposes [82]. Each particular mass extinction appears as the ultimate consequence (through amplifying coextinction processes leading to cascading losses of species; [83–85]) of an idiosyncratic combination of catastrophic events including intense volcanic activity, meteoritic impacts, glacial cooling, harsh hot-house climate, oxygen depletion leading to oceanic anoxia, abrupt sea-level change, and global tectonic events causing destruction of habitat areas [81,86–88]. Regardless of the original external cause(s) that initiated the extinction process, common biotic consequences leading to similar postcrisis diversity dynamics do emerge from severe disturbance of local ecosystem functioning.
For instance, the statistical analysis and modeling of the postcrisis fossil record associated with Phanerozoic mass extinctions led to the concept of delayed recoveries [89,90], i.e., a low-diversity survival phase whose duration is roughly proportional to the extinction intensity. This decay is classically thought to be the direct consequence of prolonged harsh environmental conditions, ecological disturbance and/or preservation failure in the aftermath of the extinction event. It is usually associated with logistic-like (single, coupled or multiphase) models of taxonomic recovery [5,6,91], which involve linear relations between taxonomic richness and per-taxon origination and extinction rates (Fig. 2A). Such a delayed recovery model is frequently illustrated by looking at the end-Permian mass extinction, the most severe Phanerozoic biotic crisis to date [73], where the postcrisis survival phase is thought to span the entire Early Triassic (i.e., ∼5 myr), and the full recovery to take up to ∼40 myr [78,90,93–96].
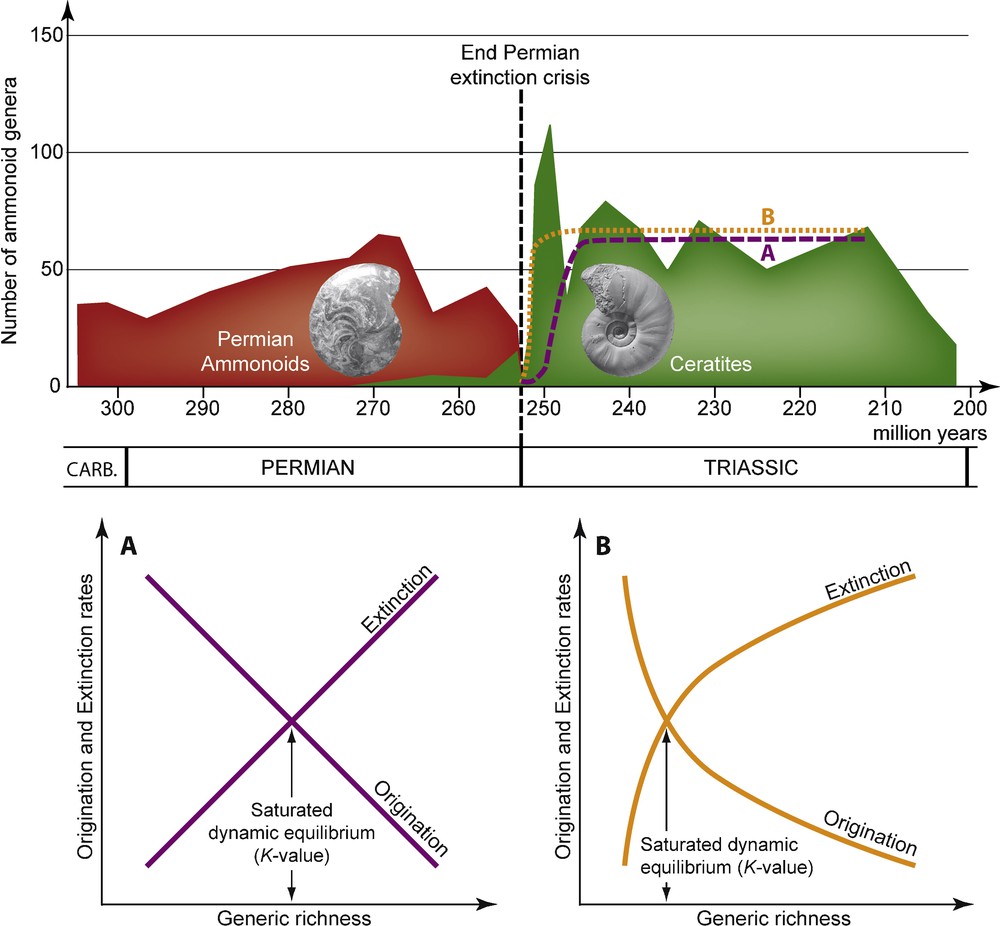
Evolution of the number of ammonoid genera from the End Carboniferous (307 Ma) to the Triassic/Jurassic boundary (201.5 Ma), with postcrisis Logistic (A) and Hierarchical (B) models of diversification (adapted from [92]). Several ammonoid groups (not differenciated here, in red) coexisted during the Permian, but a single one, the ceratites (in green), which originated ∼20 My before the end of the Permian, survived the extinction crisis. The logistic model corresponds to the two-parameter logistic function and the Hierarchical model corresponds to the three-parameter Gompertz function , where S0 is the starting (t = 0) generic richness, K is the steady-state limiting value of S (when t → ∝), rmax is the intrinsic rate of increase (i.e., the maximal rate of richness change, predicted at S = 0), and aOri and aExt are the slopes of the linear relations between ln(S) and the origination and extinction rates, respectively.
Nevertheless, recent studies on ammonoid cephalopods based on intensive fieldwork and dataset compilation illustrate an “explosive” recovery spanning ∼1 myr [92]. Overall, the observed postcrisis diversity dynamics (assessed by changes in generic richness) do not follow a logistic-like model, but rather a hierarchical model of diversification [14,97], involving exponential relations between generic richness and per-genus origination and extinction rates (Fig. 2B). Remarkably, this rapid and non-delayed ammonoid recovery is associated with the onset of a strong latitudinal structuring of faunal assemblages, shifting from a cosmopolitan distribution of taxa to a highly endemic organization [19,21,98]. Such a rebuilding of geographically-structured ammonoid assemblages is coeval with the remodeling of a steep latitudinal gradient of generic richness, which strongly suggests that the main driver of the ammonoid recovery was the shape and intensity of the latitudinal gradient of Sea Surface Temperature [19,20,26]. Incidentally, those results suggest that middle and late Early Triassic climates could have been not as equably warm as classically considered [21,99–101].
How fast biodiversity and ecosystems recovered after the end-Permian biotic crisis is still an open debate [21,92,93,95,96,102]. Against classical evidence of a long survival phase under harsh environmental conditions in an anoxic/disoxic ocean, recent findings on gastropods [103], bivalves [104] and trace fossils [105,106], as well as ongoing works on diversified metazoan reefs do suggest that benthic communities also quickly rediversified in the aftermath of the end-Permian crisis. Nevertheless, the non-delayed recovery of several groups of nektonic as well as benthic primary and secondary consumers logically requires the simultaneous recovery of primary producers, which has not yet been clearly shown for reasons that remain to be further elucidated, especially in the light of their temporary absence in the fossil record, the so-called “Lazarus effect” [107].
Another puzzling point concerns the origination/extinction dynamics underlying the postcrisis ammonoid recovery. Indeed, the high taxonomic richness level quickly reached by these molluscs during the Early Triassic results from simultaneously very high origination and extinction rates, leading to atypically high turnover rate values, and thus very short median taxonomic duration. For instance, based on available data, a median genus longevity of ∼300 kyr can be estimated for late Early Triassic ammonoids, strongly contrasting with values estimated for the Middle (∼1.5 myr) and Late (∼3 myr) Triassic [92]. The resulting “fireworks” diversification dynamics is an interesting feature of this postcrisis recovery that remains to be further evaluated and contrasted with others clades and recovery phases. In the case of Early Triassic ammonoids, it could indicate that these nektonic molluscs did recover in physically/chemically harsh environmental conditions that were strongly disturbing, but not preventing complex ecosystem from rebuilding and functioning.
3.2 Diversity and climate: a local to regional perspective
At the scale of the entire biosphere, life is not homogeneously and uniformly distributed. Several physical and chemical parameters, and most particularly climate, are the proximal causes for this biotic heterogeneity. Indeed, living beings are not homogeneously and uniformly distributed on Earth because the physical and chemical conditions that prevail at the surface of our planet are neither homogeneous nor uniform. As a consequence, while the description and modeling of global patterns in biodiversity have a long tradition in macroevolutionary palaeobiology, such studies frequently fail in identifying the fine causal relationships linking biodiversity to climate, due to the spatial heterogeneity of environmental reactions to global climate changes. In order to be properly addressed, such relationships must be investigated at a closer geographic level of local population (community) and regional metapopulation (metacomunity) functional assemblages. From both descriptive and comparative points of view, this local to regional level of analysis requires high quality and high-density data based on long-term fieldwork and systematic palaeontology.
Over the last decade, the study of metacommunities [108] and metaecosystems [109] offered significant advances, identifying this regional scale level as an ecologically and historically meaningful level of functional organization. In the fruitful context of the “Neutral Theory of Biodiversity” and associated questions and predictions (e.g., the functional equivalence hypothesis, the dispersal vs. niche assembly theories, the modeling of species abundance distributions, the species-area relationship, etc.; [110–114]), the metacommunity quickly appeared as the fundamental “Operational Ecological Unit” of neutral macroecology–in the very same way the population is the focal unit of the neutral theory of molecular evolution [115]. Given the geographic extent and associated evolutionary dynamics of regional metacommunities (Fig. 1) and due to the very nature of the fossil record, their deep-time study shows some important operational advantages over local and global analyses. With respect to local analyses, the regional integration of local incidence data strongly mitigates the effects of various sources of local biases (environmental, taphonomical, sampling-related, etc.), because a regional taxonomic assemblage is a synthesis (and not a sum) of information gathered over a set of local samples. Thus, for purely probabilistic reasons, it is expected to be less incomplete relatively to the original regional metacommunity than the local samples relatively to their respective original local communities. With respect to global analyses, data compilation and analysis at the regional scale considerably lower: (i) the sources of taxonomic conflicts between independent authorities; and (ii) problems of temporal correlations over large spatial scales. For these reasons, the descriptive and comparative study of the within- and among-metacommunity deep-time dynamics appear legitimate and pertinent in both theory and practice, justifying the analytical effort done by some workers over the last few years.
First, at an inter-regional level, several analyses of compositional similarities among taxonomic assemblages illustrate the combined long-term effects of climate and geography on the evolution of the spatial organization of faunas in both marine and continental realms (e.g., [25,98,116–118]). For example, using species-level data in a short and well-studied Early Jurassic interval, Dommergues et al. [117] found that neighboring but totally distinct ammonite provinces recorded similar variations in richness and endemism at a time of warming of the seawaters. Nevertheless, that warming did not cancel out the stark palaeobiogeographic contrast between these ammonite provinces, suggesting that palaeogeographic and eustatic (i.e., sea-level fluctuations) features were certainly paramount over climate in that particular case. In contrast, other studies on ammonoids have shown that a warming of the seawaters may enhance cosmopolitanism (e.g., [21,98]).
An example for terrestrial metacommunities is given by Costeur et al. [116] and Maridet et al. [25], who discussed the evolution of inter-regional biogeographic relationships in Europe during the Miocene and Early Pliocene (from ∼23 to 3 M.a.) as illustrated by ungulate (i.e., large primary consumers) and glire (i.e., small primary consumers) mammals, respectively. Results from incidence-based similarity analyses performed at the biozone level were compared to the geographic and climatic framework available for that time interval; they show that the biogeographic dispersal of Miocene ungulates and glires was driven by rather distinct, if not opposite controlling parameters. Indeed, whereas the inter-regional taxonomic homogeneity of ungulate assemblages regularly increases from ∼22 to ∼8 M.a., and then abruptly decreases in the latest Miocene and earliest Pliocene, i.e. at the time of the Messinian salinity crisis [119], glires show an almost perfectly inverse story. The close parallelism between the Miocene inter-regional taxonomic similarities observed for glires and the long-term climatic evolution [120] strongly suggests that the mean annual temperature is the main driving parameter of the evolution of the geographic distribution of these small primary consumers at the continental level. Conversely, at least two, nonexclusive kinds of parameters may be invoked to explain the deep-time trend observed for ungulates: (i) the evolution of the available vegetal biomass, controlled by various physiographic and climatic parameters (e.g., pluviosity, evapotranspiration, etc.); and (ii) the evolution of the palaeogeographic context controlling intra- and intercontinental migration routes (Miocene is the time of the Eastern closure of the Tethyan Ocean and Alpine orogeny). Essentially similar conclusions were reached more recently in a study focusing on the evolution of Miocene macro- and micromammals in the Iberian Peninsula [118]. Interestingly, such observations and hypotheses closely echo widespread observations that on average, micromammals are more climatically specialized, and thus more prone to biomic specialization than macromammals [27,121,122]. Clearly, such results still remain to be further explored, e.g., through the construction of spatialized simulation models incorporating constraints on life history traits [29].
Second, at an intraregional level, studies of deep-time γ-diversity dynamics illustrate the respective role of climate changes and large-scale biogeographic events on the compositional turnover and structuring of metacommunities, especially for mammals [17,22,24,123–130] and fishes [131]. For instance, the diachronic comparative analysis of local vs. regional late Paleogene (38–22 M.a.) mammal assemblages within the Quercy and Limagne area (Massif Central, France) illustrates distinct community and metacommunity evolutionary dynamics, involving at least partially independent sets of controlling parameters [24,130]. On the one hand, local community-level cenogram analyses [132] clearly illustrate the greenhouse to ice-house transition recorded worldwide around the Eocene/Oligocene boundary (EOB, ∼34 myr ago; [120]; see fig. 4 in [130]). This shows that characteristics of mammal local communities such as α-richness and body-weight structuring strongly depend on climatic and environmental conditions and their long-term evolution. On the other hand, the regional mammal metacommunity appears more robust to climatic and environmental changes, the γ-richness being relatively stable throughout the 16 myr analyzed time interval due to higher β-richness values in cold and arid than in warm and humid conditions. Nevertheless, a closer look at the metacommunity dynamics based on the analysis of the prenascence and survivorship poly-cohort matrix [24,130] indicates that the regional assemblage actually does react to the major climatic transition recorded around the EOB. Indeed, from ∼34 M.a. onward the metacommunity shows a ∼2 myr cyclic behavior corresponding to a periodic oscillation between an origination/immigration-dominated phase and a following extinction/emigration-dominated phase. Remarkably, this regular dynamics closely echoes the ∼2 myr rodent species turnover cycle recorded in a 22 myr-long Neogene time series from central Spain [129]. This cycle has been associated with low-frequency modulations of Milankovitch oscillations (Fig. 3), whose stable isotope signatures (δ13C and δ18O) are recorded worldwide in deep-sea sediments from the earliest Oligocene onward, but not before [120,130,133]. In any case, local and regional diversity changes thus appear independent from each other, highlighting the overall importance of spatial integration in the historical and functional processes that control biodiversity dynamics.
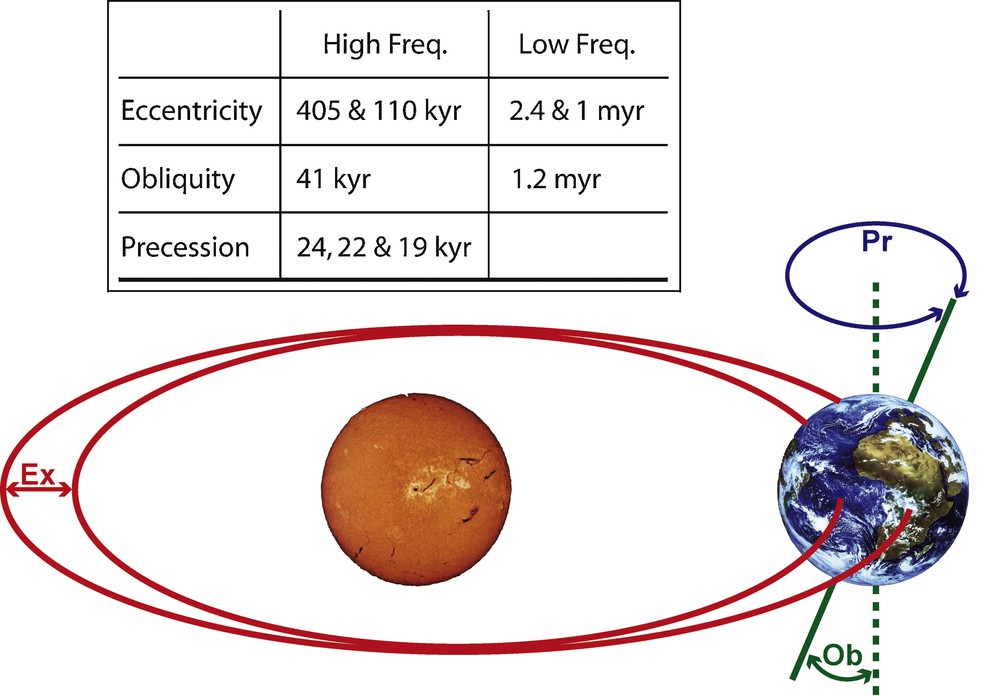
Milankovitch orbital cycles, regulating secular variations of terrestrial insolation. Ex: eccentricity (orbital shape); Ob: obliquity (axial tilt); Pr: precession of the equinoxes (axial gyroscopic motion). Inset: main periods associated with the high- and low-frequency cycles. Low-frequency cycles modulate the amplitude of their corresponding high-frequency cycles; they combine into a ∼2 myr main period cycle.
4 Concluding remarks: biodiversity is an intrinsically multidimensional parameter
In a physically heterogeneous and ever-changing world, fluctuations in biodiversity are the rule: continuously, new species originate and others become extinct as the direct consequence of the Darwinian coupling between random mutations and natural selection. From this self-organized, nonstationary dynamic equilibrium, sometimes perturbed by more or less catastrophic events, the necessity to fully and explicitly integrate the spatial dimension into the (deep-time) analysis of biodiversity dynamics is flagrant. Indeed, patterns and processes of diversity changes through time do vary between levels of spatial integration, with new and unpredictable effects and characteristics arising at each focal level, making impossible direct extrapolation from one level to another [14,17,28,134,135].
In this spatially integrated approach to deep-time biodiversity dynamics, most studies achieved so far exclusively focused on the taxonomic dimension of diversity. For both conceptual and practical reasons, there is now an urgent need to consider other, complementary components of biological diversity, including the morphological [136,137], phylogenetic [138,139] and functional [140,141] dimensions of diversity. Beyond the theoretical advances that can be anticipated from such a comprehensive and integrated approach, especially in order to untangle functional and historical processes at different spatial scales, significant progress in conservation strategies can be expected [142].
Finally, based on our current knowledge and understanding, two major abiotic drivers of deep-time taxonomic diversity dynamics emerge: the climate and the amount of available space. A global increase of temperature associated with a lowering of the latitudinal gradient of temperature (as all climate scenarios predict for the century to come; [143]) will necessarily lead to a decrease in global diversity through geographic homogenization of species assemblages. On the other hand, the increasing anthropic pressure exerted at all latitudes and on most terrestrial and oceanic ecosystems in the last few centuries, results in a dramatic increase in the fragmentation of environments, a major cause of decrease in local diversity [13]. The cocktail is explosive: combining these two factors–global warming and local demographic and agrarian pressures–, the biosphere may be currently entering its sixth major extinction crisis [84,144–146]. How and to what extent this happens is one of the main topics addressed in this issue of the Comptes Rendus Biologies.
Disclosure of interest
The authors declare that they have no conflicts of interest concerning this article.
Acknowledgments
We are mostly indebted to the French Academy of Sciences, and most particularly to J.-D. Lebreton and H. Décamps, in giving us the opportunity to present this paper during the public meeting “La biodiversité face aux activités humaines” (Paris, 2010/02/09). We thank Manuel Hernández Fernández and an anonymous reviewer for constructive comments. This work is a contribution of the former UMR-CNRS 5125 “Paléoenvironments & Paléobiosphère”, now UMR-CNRS 5276 (G.E., S.L.), and UMR-CNRS 5561 “Biogéosciences” (E.F., A.B.); it was funded by the Région Bourgogne (A.B.) and the Fondation pour la Recherche sur la Biodiversité (G.E., E.F., A.B.).