1. Introduction
Biodiversity is classically defined as the variety of life on Earth, from the within-species to the ecosystem level, including both biotic and abiotic components and their complex interactions [1]. Resulting from more than three billion years of biological and geochemical co-evolution, it has also largely contributed to the Earth’s geochemical history, adding colossal masses of, for example, carbonaceous structures (coal, oil, etc.), particularly via photosynthetic activity or the construction of vast coral reefs, over the last billion years (see [2, 3, 4, 5] for a quick overview). Biodiversity is also the product of a history of biological complexification and diversification, from the rise of the first living structures around 3.5 to 4 billion years ago, to very large multicellular organisms, via the appearance of multiple bacterial or eukaryotic groups and increasingly complex ecological chains, often under extreme living conditions (see [6, 4, 5]). This unfolding in time and space with the expansion of continents, their collisions and their separation, has also been marked by large-scale events, due to causes both internal and external to the Earth (e.g., a giant meteorite impact at the Cretaceous–Paleogene boundary 65 million years ago), freeing up evolutionary spaces and leading to the domination of new groups (e.g., [7, 8, 9, 10]). As a consequence of this long ecological and evolutionary history, biodiversity can be considered as a complex adaptive system or network [11, 12, 13].
A (retrospectively) singular event took place 3 to 4 million years ago, somewhere in Africa—from the lineage of the African–Asian great apes, evolved hominid species that straightened up, freeing their hands for tool use, and developing large brains and intense social skills (e.g., [14, 15, 16, 17]). While these developments, and a few others, certainly did not make these species more competitive in any particular respect (for example, many of them were already present in other mammals; these hominids, taken individually, were no match for a lion), they did make them surprisingly plastic and adaptable to various environmental conditions. The bushy evolution of these species resulted in the emergence of (self-named) Homo sapiens. Through its ability to manipulate the environment (e.g., fire or stone) and to handle artifacts, H. sapiens began to transform the planet, to such an extent that Crutzen [18] proposed to call our era the Anthropocene1 . The very notion of Anthropocene remains debated among scientists—for example, it has not been adopted as an era by the International Union of Geological Sciences [20], but one can consider that the human impact began about 50,000 years ago and became serious at the beginning of the Holocene, some 11,000 years ago [21, 22]. This impact is today as powerful as that of geological or climatic forces, in many ways much more rapid, and quite clearly the origin of the current biodiversity crisis [23, 21, 24, 25, 3, 26].
This crisis can be defined as biodiversity general collapse and homogenization—recall that the term biodiversity was coined in the mid-1980s in response to the perception that biodiversity was in crisis [1]. There is little doubt that it is caused by environmental (e.g., climate change) and biotic (e.g., biological invasions) changes induced by human activities [26, 27, 28, 19, 29]. These activities have created new and impactful environmental regimes, so much so that biodiversity studies can hardly be conducted without firmly integrating human impacts [25, 30, 21, 31, 24]. It should also be highlighted, and regretted, that this crisis has attracted far less attention than climate change in the general public, stakeholders and politicians, although the two are more than intertwined—the biodiversity crisis, in fact, covers all aspects of the Anthropocene, including the climate crisis [32, 19, 30, 33, 29].
The Anthropocene has been the subject of a large number of studies, particularly with regard to the Earth’s biogeochemical functioning and its impact on biodiversity. The ecological sciences in particular have been widely mobilized to understand the biodiversity crisis [21, 28, 19, 34], but the focus has been on species ecology, i.e. the human impact on the spatial and temporal dynamics of individuals or ecosystems. However, less attention has been paid to the impact on intraspecific diversity or adaptive potential (in other words, how life can adapt to change), and thus to longer-term consequences, i.e. considering the evolutionary dynamics of biodiversity [35, 36, 37, 38]. We note, however, that Folke et al. ([25], Figure 6) mention genetic diversity as one of the planetary limits for which we are in a high-risk zone, and that considering this diversity in management practices has been addressed in the evolutionary literature for several decades [39, 40]. This major change in ecological regime and conditions not only impacted biodiversity per se, but also the conditions (forces, drivers and processes) of its evolution—such a fast disruption by a single species has never happened, and will have short- and long-term evolutionary consequences. Moreover, even if it is rising, the appreciation of the interaction between ecology and evolution, through eco-evolutionary feedbacks, remains undervalued when it comes to tackling the biodiversity crisis in the short term.
The general question addressed here is why we should take intraspecific diversity and adaptive potential, and the eco-evolutionary feedback loops that arise from them, into account in our understanding, prediction and management of the biodiversity crisis in the Anthropocene. The paper is structured around five main sections: in Section 2, I briefly present the major avatars of the Anthropocene, i.e. stressors such as climate change, setting them in a short-term temporal perspective (e.g., how they have and will evolve over decades, and how we can set targets for reducing their magnitude and impact), before providing in Section 3 an analysis of the ecological crisis and its impact on biodiversity through a (forward) temporal perspective. In Section 4, I outline the reasons why biodiversity should be considered from an eco-evolutionary perspective, including how species respond to environmental drivers and how eco-evolutionary feedbacks are affected. Section 5 is devoted to predictions about future biodiversity, focusing on the short term (a few decades). For example, how are predictions modified when evolution is accounted for, and is this relevant? Or is the crisis too violent to worry about evolution? Section 6 is devoted to action, integrating an eco-evolutionary perspective in the various Nature-based solutions to the biodiversity crisis, i.e. solutions that take biodiversity into account.
2. The many avatars of the Anthropocene
Over its long-term evolution, biodiversity has never, in essence, been stable, and has varied more or less rapidly under the influence of both ecological and evolutionary processes. However, the recent irruption of humans imposes/ed biotic or abiotic environmental changes at an unprecedented speed—on time scales shorter by two to four orders of magnitude [21, 28, 19, 13]. Human activities emanate in many forms, and here we will focus on, and take examples from, climate change, land/sea appropriation (fragmentation and use), and biological invasions, among the most impactful “stressors” on biodiversity2 . The latter two have been in place since the emergence of human societies [22, 21, 41] and the former over the last century and half [19], with marked and well-documented impacts, and are unlikely to stop for several decades, even under future optimistic scenarios on human activities.
The most discussed facet of the Anthropocene is climate change (e.g., [25, 33, 41, 38])—the strongest and best-documented expression of global change, and probably also the most keenly felt, as it directly affects biodiversity, including humans, who experience not only an average increase in temperature, but also more frequent extreme events, such as with marine heatwaves that have about doubled in frequency since the 1980s ([42], chapter 11; [43]). It is therefore a planetary force, omnipresent and multifaceted. The proven increase is already 1.3 °C above the pre-industrial mean value (see [44]) on a global scale, with significant regional variation: temperatures have risen more markedly on lands than on seas, with e.g., the increase in Europe being already 2.3 °C [45]. Marked temporal variation, both on the scale of decades and within years, is also obvious. For example, 2023 has been the hottest year ever, with 1.5 °C above the pre-industrial mean value [44]. Intra-annual variation is also obvious, with, for example, a more marked increase in spring in particularly hard hit Western Europe. The impact on temperatures is largely coupled with an impact on rainfall [42, 46]. But here again, a key feature is spatiotemporal variability, which is particularly important, as it is one of the driving forces behind the functioning of biodiversity [47, 48]. See more details in Appendix 1 (see Supplementary Materials).
Furthermore, it is hard to see how the average temperature could not exceed the pre-industrial average by 2.1 to 3.5 °C by 2100 [42], unless very marked socio-economic changes and mitigation measures take place. Even in the latter situation, the return to pre-industrial values could take centuries, if not millennia, due to the inertia of the Earth’s system [34, 49]. It is, of course, difficult to predict future trends, as they will depend on factors that cause temperature increase (e.g., the size of the world’s human population) or decline (e.g., effective efforts to reduce the injection of greenhouse gases into the atmosphere). We can therefore envisage growth leading to a plateau, or even to a decline if actions in this direction are attempted. This can be visualized in Figure 1, using the idea of an “overshoot period” developed by Meyer et al. [34]—the period during which temperature will exceed a “desirable” threshold value (for example, 2 °C over the pre-industrial mean value). This period can last for varying lengths of time (e.g., decades) before returning to conditions that are more favorable to biodiversity, depending on the implementation of mitigation actions and their temporality (Figure 1), and may even be of “infinite” length (centuries). The notion of overshoot makes it possible to arbitrarily fix the duration of the effect in models and scenarios, and to assess how biodiversity will respond to these new conditions in terms of eco-evolution over several decades to centuries. Note that (i) spatial variability in temperature increase can be accounted for in this framework by considering local overshoots; (ii) these curves may not necessarily be smooth, and may include local peaks (e.g., extreme events); (iii) they may account for “tipping points and alternative states” when the overshoot period is very long. We will use this representation in what follows to understand the eco-evolutionary impact of stressors on biodiversity, to predict its future and to consider Nature-based solutions to global change.
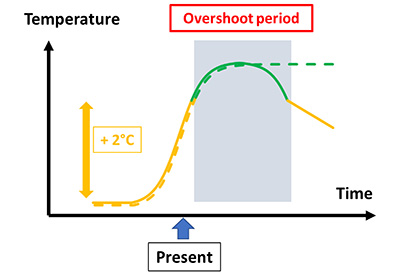
Time curves of consequences of human activities, past and future (modified from [34]). The curve baseline is the global pre-industrial temperature, and they increase up to a desired target temperature that should not be trespassed (2 °C here for the sake of the argument). The continuous line represents a situation in which this threshold is trespassed for some time (the overshoot period), and then the temperature goes down below the target, due to e.g., mitigation actions. The dotted line represents a situation in which the temperature does not go down to the target, for example because no strong mitigation actions have been set up, and remains high (here in a plateau) for a period much longer than the overshoot period. The option of continuous increase is not represented. The present is indicated by “Present”. Masquer
Time curves of consequences of human activities, past and future (modified from [34]). The curve baseline is the global pre-industrial temperature, and they increase up to a desired target temperature that should not be trespassed (2 °C here for the ... Lire la suite
The impact of other stressors (space appropriation and invasive alien species) on biodiversity is no less worrying and marked. Figure 1, including the overshoot period idea, can be used, as for climate change, to understand the dynamics of the consequences of these stressors (detail in Appendix 2, in Supplementary Materials). They all have a dynamic of strong growth compared to the pre-industrial era (which began at various times depending on the stressor), and this dynamic will reach a point that we do not wish to exceed—the beginning of the overshoot period. This point is probably less easy to determine than for temperature, for which we have a simple determination criterion (here, +2 °C)—we can, however, imagine that we do not wish to have more than “a given number” of invasive alien species or an increase in lands devoted to agriculture (Appendix 2, in Supplementary Materials). The duration of the overshoot will also vary from stressor to stressor. For example, in the case of invasive alien species, it is hard to imagine that we will be able to go back to the initial situation, if we define the overshoot based on the number of such species in a given location, but it is possible to reason about the effects on invaded ecosystems. See Appendix 2 in Supplementary Materials for more details.
These three stressors have been considered independently, but they may act in concert, often synergistically and through feedback loops [50, 51, 52]. Let us take two examples. The first combines the effect of climate change and the exploitation of natural resources (overfishing as an emanation of sea appropriation). Climate change is responsible for major direct changes in phytoplankton, while fishing alters directly the fish community that also influences phytoplankton. Both thus alter phytoplankton and, ultimately, trophic networks with complex cascading effects on the fish community and the opening to invasive alien species [53]. The second is the emergence of new areas for biodiversity as a result of global warming, for example at the glacial margins towards the poles, with retreats that can be of the order of km/yr [54]. These areas can be rapidly artificialized, for example for shipping or oil exploitation, and subject to biological invasions [55, 56]. For the first effect, we can imagine a very rapid increase in dynamics (Figure 1), even under those extreme environmental conditions and the same type of dynamics as observed elsewhere under less extreme conditions. Biological invasions, on the other hand, might be slower and limited to a few species—these dynamics can be inferred from post-glacial colonizations, particularly the most recent (see e.g.,[57, 58, 59]). The time scale is likely to be of the order of a few centuries. What we can learn from these examples is that we should analyze in more details combination of effects, but they seem to be synergistic rather than antagonistic (e.g., [50, 51]). The overshoot period (Figure 1) could therefore be more pronounced and longer, with a much longer return (if any) to pre-anthropocene conditions. Whatever the case, stressors, taken individually or in concert, create new ecological and adaptive opportunities in the context of new complex adaptive systems, with major impacts on biodiversity and its dynamics.
3. The impact of human activities on biodiversity
Human activities and the above-mentioned avatars of global change impact biodiversity widely in many respects, and we here provide a simple review and an evaluation of the incidence on both ecological and evolutionary (in the next section) processes. This means distinguishing effects on biodiversity itself vs. on ecological and evolutionary forces and processes—a classical distinction in the ecological sciences between patterns and processes [47, 48]. Importantly, this presentation takes a time perspective: the effect of stressors on biodiversity is certainly past and present, but will also manifest itself in the future, because the overshoot period can last for some time (Figure 1), even if this effect was to be stopped now. A currently apparently healthy species can indeed decline soon, perhaps after trespassing an ecological tipping point (see [30]), such as in the classic study of excessive phosphorus impact on lake eutrophication status [60]—we are talking here about the debt (or legacy) of the crisis [61]. Getting cues of this debt (so-called early warning signals) is difficult, because of the intrinsic unpredictability of such dynamics, but progress is being made on this side (see [30]).
Biodiversity has probably never been as flourishing as it is today (before Human impact)—it is quite clear in terms of species numbers [19]. However, it has been struck by five major extinction events—defined as short periods during which more 75% of species disappeared [62, 9] followed by recovery over several million years. All these events are due to causes external to life itself, either endogenous (e.g., volcanism) or exogenous (e.g., meteorite) to Earth. Although we are far from such a massive extinction event, human activities and their consequences have reached a level on all geographical scales that makes the occurrence of a sixth extinction not unlikely [63, 27, 19, 64]. One originality of this crisis is that its source lies in both environmental (e.g., climate) and biotic (e.g., biological invasions) changes caused by human activities. Quantifying this crisis assumes that we “know biodiversity”. This is far from true, with optimistic estimates of ca. 20% of described species, i.e. two million out of an estimated value of eight to 20 million living species [65, 66]. Available data (arguably limited) focus on terrestrial animals (70,000) and plants (400,000) with far less constituted datasets in the marine realm and in small organisms, especially “microbes”. Our knowledge of population dynamics and of trait variation is even more limited.
The first and quintessential expression of the biodiversity crisis, and its first definition, is species extinction [63, 67]. A very conservative estimate of the current extinction rate is ten times larger than the background rate [68, 19, 13]. Seven hundred vertebrate species (at least), out of 70,000 described species, have disappeared over the last half millennium, while a few species are expected to appear/vanish over such a period through speciation/extinction, so that the vertebrate extinction rate, assuming a quasi-steady state of species number, might be 100 times higher than the background rate. Importantly, species extinction has accelerated over the last century: for example, two thirds of mammal extinctions recorded over the last half millennium have occurred since 1900 [13]. In this biodiversity collapse, islands have often paid a huge tribute. For example, 13 of the 22 native bird species from Guam have disappeared since the introduction of a predator snake [69], and half of the more than 700 terrestrial snail species from Hawaii have experienced the same fate, producing a 7.5 to 13% extinction rate since 1500, some thousand times higher than the background rate—ironically, new species are described that have already disappeared [70, 71]. It should also be added that many species will have to pay the above-mentioned extinction debt [61]. Even under scenarios with a temperature increase of 2 °C and a brief overshoot period, 10% of species are at risk of extinction [34]. The magnitude and duration of this period are therefore critical values.
However, even if impressive, this statement relies on an incomplete diagnostic: (i) species are disappearing, but the number of individuals per species (species abundance) is also collapsing. A striking example are European birds with a decline of 25% between 1980 and 2016, with a major contribution of agriculture to this trend [29]. In insects, a marked decline in number (and also biomass) has been reported (e.g., [72]), but the trend might differ among environments [73], as also observed in marine species [74], in a background of strong methodological discussions [75, 76]. (ii) Rapid reorganization of species assemblages (biodiversity redistribution and homogenization) is occurring on a global scale [77, 78]. This poses an ecological problem, as this reorganization is largely due to opportunistic and invasive species that might affect species diversity by increasing extinction rates in local species (see point (i)). For example, marked effects have been detected in the oceans with dramatic changes in species composition in many regions, although not always associated with a loss in species richness [79]. (iii) All stressors also have deep consequences on ecosystem functioning, profoundly modifying interactions among species and between species and their environments, an expression of which being ecological extinction. To the extinction debt mentioned above, we add here an ecological debt: species, although still extant, are no longer sufficiently abundant to perform their functional roles [80] (and even worse when they are extinct). For example, 40% of plant species are considered endangered [81] with a substantially decreased contribution to food chains and ecosystem processes. Of course, other species, including invasive ones, can sometimes serve as substitutes, an expression of ecological functional equivalence [82]. This will be difficult if whole communities and food chains reach tipping points and transition towards degraded states, as is the case for lake eutrophication [60] or desertification [83]. Less visible, but not less important, is the case of kelp forests, which occupy almost 30% of coastal ecosystems, and are declining fast and might soon reach a point of no return [84]. (iv) Interaction between stressors might lead to even more negative outcomes than their simple addition. To the ecosystem-level example mentioned above, we should add population-level examples with perhaps more directly visible effects. For example, population shrinking might be compensated by immigration, as classically shown in metapopulation theory [85]. However, this assumes that appropriate habitats indeed support populations contributing to migration, but such habitats are themselves under pressure (e.g., because of land appropriation), reducing metapopulation size, which could be further decreased by competition with invasive alien species down to collapse [85], as shown in freshwater snails in the French Antilles [86]. (v) A small part of species has indeed benefitted from human impacts, namely commensals and domesticates, with a strong impact on biodiversity, and we should also modestly recognize that the bacterial world remains a black box with regard to biodiversity massive change. These two aspects are considered in more detail in Appendix 3 (see Supplementary Materials).
We can conclude this section by stating that human activities have markedly shaken biodiversity at all its organization levels, from population to ecosystems, and spatial scales, with a major reorganization of species, communities and ecosystems, even if we are not (yet) experiencing a classical major extinction (i.e., 75% species extinction; [87, 27, 19]). As a consequence, most species are facing new environmental conditions to which they are not necessarily adapted (new adaptive landscapes) and are therefore under strong demographic pressure, possibly up to ecological tipping points [25, 30], and constraining evolutionary processes and potential, the scope of the next section.
4. Biodiversity and eco-evolutionary dynamics
At this point, we have not considered intraspecific diversity, and the associated adaptive potential, in the biodiversity crisis. In fact, it has been less considered than the higher levels of biological organization considered above. However, genetic diversity is considered one of the planetary limits for which we have reached a high-risk zone [25], but intraspecific diversity loss remains much more difficult to document than biodiversity loss at the species level [88]. Avatars of global change often result in a decrease (collapse) in population size in impacted species and ecosystems, which might lead to the loss of infraspecific diversity (as species extinctions do), enhancing the role of stochastic processes, such as genetic drift (e.g., [89]), possibly driving populations in an extinction vortex, with a decreased number of individuals leading to decreased genetic variation, itself leading to a decreased number of individuals. This has been suggested for some large mammal species, such as the vaquita [90]. Theoretically, such loss can be rescued by incoming gene flow, provided that immigration is not impeded [89, 47]. Global change can also induce a new selection regime or the mobilization of intraspecific diversity. There is for example wide evidence that climate change induced both selective responses and mobilization of phenotypic plasticity ([36, 91, 38] for recent perspectives), as detailed in Box 1. Another effect of global change, the genetic counterpart of biota homogenization at species and community level, is genetic mixing between entities (species or lineages) that were previously separated, especially through the introduction of invasive alien species. Such secondary contacts may promote hybridization and introgression, and the emergence of evolutionary novelties [92, 93]. So intraspecific diversity is not just lost, it is also being restructured, especially in the context of large-scale biodiversity hauling, similar to what we have seen above at the species and community levels.
That global change affects all aspects of biodiversity is certainly an incentive to take both an ecological and evolutionary stance on this issue. After all, ecology and evolution are the core natural sciences in the study of biodiversity dynamics (see [89, 47, 48]). However, ecology has classically been seen as dealing with short time scales, and evolution as operating on much longer time scales [94, 95, 96]. Another way to put it is from a perspective of biodiversity organization levels (Figure 2): populations play a central role in evolutionary biology and, to a lesser extent, in ecology (population ecology), and the latter becomes more important as we move towards the more integrated and functional levels of biodiversity, i.e. communities and ecosystems.
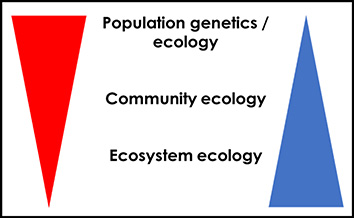
Simplified diagram of the decreasing impact of evolutionary theory (red triangle) in studies considering increasing levels of integration of biodiversity (blue triangle), moving from populations (in fact, genes and individuals; largely including population genetics and evolutionary biology, but also population ecology) to communities (community ecology) and ecosystems (ecosystem ecology). The blue triangle could also be seen as an indicator of space and time, with increasingly larger spatial and temporal scales (in general) towards the figure bottom. Modified from Jarne [97]. Masquer
Simplified diagram of the decreasing impact of evolutionary theory (red triangle) in studies considering increasing levels of integration of biodiversity (blue triangle), moving from populations (in fact, genes and individuals; largely including population genetics and evolutionary biology, but also population ... Lire la suite
However, work carried out over the last few decades has shown that ecological time and evolutionary time can overlap. This is of course the case for short-lived organisms such as bacteria ([98]; Appendix 3 in Supplementary Materials), which adapt very rapidly to environmental changes [99], but also for organisms with slower life cycles, particularly when they are subject to strong selection pressures, such as mosquito populations treated with pesticides [100]. Rapid evolution has also been observed in natural populations of species exposed to environmental stress (e.g., Darwin’s finches in the Galapagos; [101]), predation (e.g., guppies in Trinidad; [102]) or invasive alien species (e.g., snails in the West Indies; [103]). The time scale argument therefore no longer holds, and a more general framework should take into account the fact that ecology and evolution can be fast or slow [95]. We focus here on the fast time scale, since centering on global change, acknowledging that “fast” might depend on generation time and stressor intensity and therefore the rate of global change stressors relative to this time ([95]; Appendix 3 in Supplementary Materials for the case of bacteria).
Box 1—From global change and overshoot periods to eco-evolutionary loops
The ecological impact of global change has been widely documented (e.g., [33]), but its evolutionary consequences have attracted less, and more recent, attention, presumably under the (erroneous) idea that evolution is not concerned with such fast changes (see main text). It is now quite clear that the evolutionary dynamics should be considered, for example when analyzing the consequences of climate change [36, 91, 38]. We use here climate change and the overshoot idea (Figure 1) to address the ecological and evolutionary consequences of global change.
We consider a situation in which climate change (temperature) has a negative impact on species demography, and that this situation lasts at least for some time (the overshoot period; Figure 1). Temperature can affect survival or reproduction (see [104, 105] for review in insects and plants). Demographic decrease is nothing exceptional in general, but can take here extreme values known as population bottlenecks, with both demographic consequences (e.g., very biased sex ratio rendering reproduction difficult) and evolutionary consequences (e.g., increased consanguinity, reduced evolutionary potential; [106, 107]). At the end of the overshoot period, demographic recovery might be possible, but evolutionary recovery is a much longer process, depending on mutation and recombination in the absence of incoming gene flow. Although difficult to document, we indeed have examples of species flourishing following recent bottlenecks, though exhibiting low variation (e.g., [108, 109]).
Not independently of the effects on demography, temperature imposes new selective pressures. Species that were are (more or less) at an adaptive peak prior to environmental change should now track the new conditions to adapt (Figure 3). In more ecological terms, they should move within their niche. They can then either be able to catch up with change, or not (A vs. B in Figure 3). At the end of the overshoot period, those species that have been able to adapt are again constrained to engage in a new round of adaptation, while those that have not might no longer be maladapted. Adaptation (peak tracking) can occur through various mechanisms, including genetic adaptation or phenotypic plasticity [110, 111]. Empirical studies have shown that plasticity is a more common option than adaptation [112, 38]. The point is whether this reserve of plasticity is abundant enough to go through the whole overshoot period, and in any case, phenotypic plasticity is not the magic bullet.
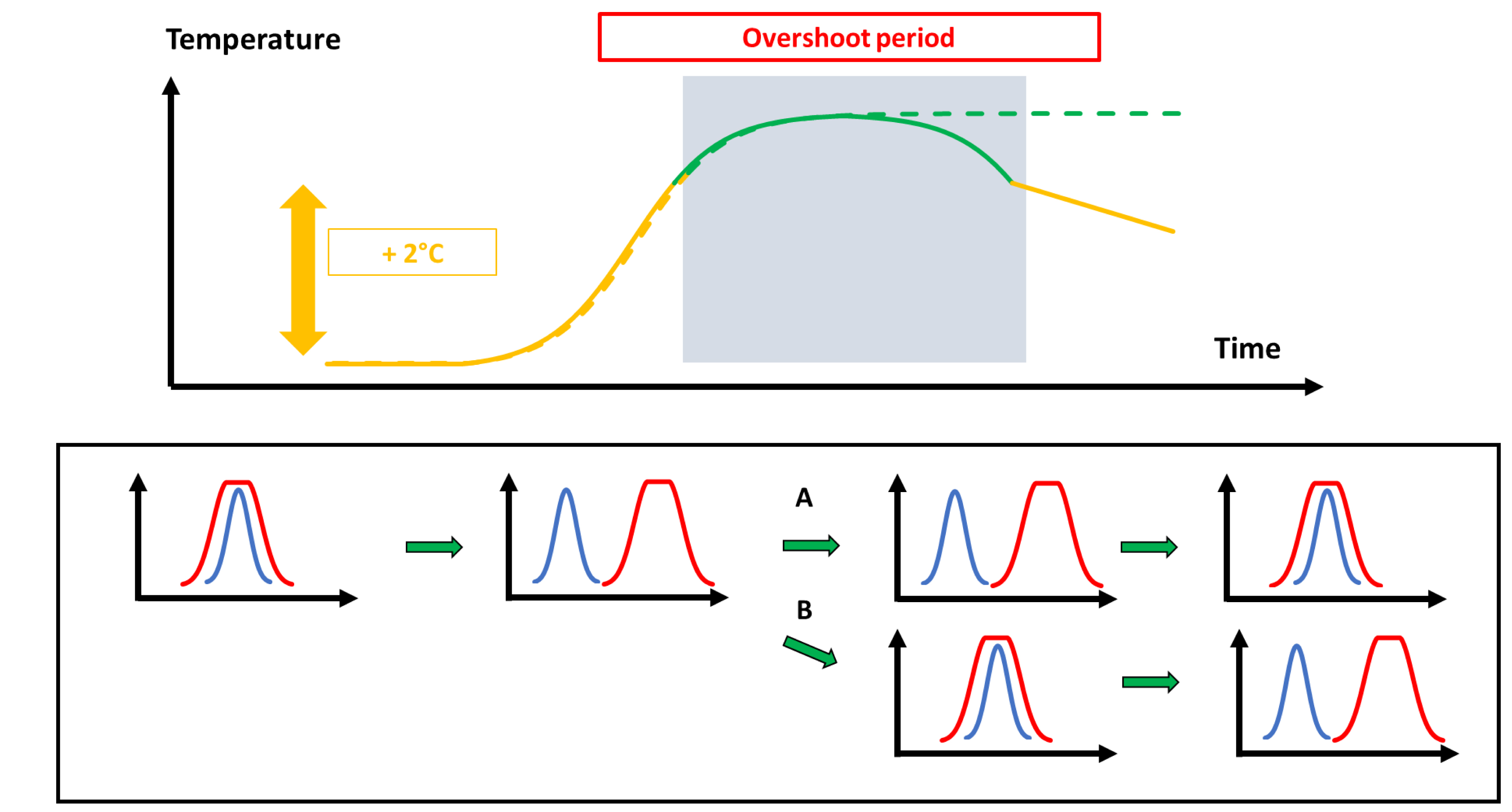
Variation of trait values when temperature changes in time under the overshoot scenario. The upper part depicts time curves and the overshoot period under temperature change as in Figure 1. The bottom part represents the temporal variation of traits (blue curves; adaptive peak in evolutionary terms; [110]) with regard to temperature variation (red curves). Strongly overlapping curves (e.g., leftmost figure) indicate excellent fit between traits and temperature, while non-overlapping curves indicate poor fit. Following a fast temperature change, the trait curve is lagging behind (second figure from left to right in the bottom part). During the overshoot period, peak tracking through adaptive evolution or phenotypic plasticity may lag behind (A) or may be efficient (B). At the end of the overshoot period (rightmost graphs in the bottom part), individuals in (A) may indeed be (again) adapted to temperature, while those in (B) are now far from the optimal trait value and have to evolve again. Note that peak tracking is here represented in a very simplified way. For example, many forces (e.g., antagonistic selection between different traits) might prevent individuals from reaching the adaptive peak (see [110, 111]). Masquer
Variation of trait values when temperature changes in time under the overshoot scenario. The upper part depicts time curves and the overshoot period under temperature change as in Figure 1. The bottom part represents the temporal variation of traits (blue ... Lire la suite
Migration is another option for peak tracking, noting that it can strongly be constrained by global change—migration indeed requires connectivity, which is restrained by land and sea appropriation. Migration brings both genes and individuals to new locations that are colonized when they are empty of the considered species, or reinforced when that species occurs in very low numbers, contributing to an eco-evolutionary response to, for example, temperature [113, 114]. Populations at the colonization front may even be selected for an increased migration rate over a few generations, as shown in the invasive toad Rhinella marina in Australia [115].
Changes in demography, adaptation and migration will also lead to new interspecific relationships (e.g., new competitors or parasites), and interactions with the environment, and new eco-evolutionary feedbacks including peak tracking. The guppy example developed in the main text is a good example at local scale. On a larger scale, poleward migration with increasing global temperatures is indeed leading to new communities and species interactions under new environmental conditions [116, 117]. These new interactions deeply affect demography, and also have evolutionary consequences, as has been shown for example for interactions between local and invasive species, leading to variation in morphological traits ([118] in lizards from the Caribbean) or in life-history traits ([103] in freshwater snails from the same biogeographic area). More complex eco-evolutionary dynamics are certainly at play, as shown in guppies. These are situations in which adaptive tracking is also more complex (compared to the simple situation depicted in Figure 3), since multiple traits, species and environmental conditions may be at stake, and therefore multiple adaptive peaks.
Beyond the issue of time scale, there are several reasons to consider ecology and evolution together. First, the basic material of both is made up of individuals (phenotypes), their numbers and traits, which can be integrated from the within-species to the ecosystem level (Figure 2). The whole dynamic of these various levels is driven by demography and individual fitness (leaving aside exchanges of matter and energy). Individuals constitute groups at the local level, which are connected by individual dispersal. This can be embedded in the “meta” perspective with sets of populations, communities or ecosystems functioning as metapopulations, metacommunities or metaecosystems connected by migration [85, 119, 120]. This brings us logically to our second point: there is a clear analogy between the forces/processes acting at these various biodiversity levels, for example between genetic drift of alleles and ecological drift of species, competition within and among species [121], or gene flow of alleles and migration of species [96, 122]—an analogy described as a parallel view on ecology and evolution by Huneman [123]. Of course this analogy has its limits; for example, natural selection at the population level (interactions between alleles) cannot take the same forms as the variety of interspecific interactions (mutualism, parasitism, competition, predation …).
The parallel view on ecology and evolution is important, but does not account for a deeper coupling due to eco-evolutionary feedbacks and loops [95, 124, 96, 125]. In the stronger version of loops, this occurs when an ecological driver affects the trait evolution of organisms, and that evolution in turn affects the ecological driver—this is what Hendry [96] calls eco-evolutionary feedbacks in the narrow sense. The long-term study of Reznick and colleagues on guppies in Trinidad [102] provides an excellent example. Guppies occupy rivers in which predation by other (invasive) fish is low or high, and an evolutionary response of several traits (e.g., reproduction, morphology) to predation was detected. Selection here derives from marked difference in population density. At high density, guppies exert a strong pressure on their food sources, which in turn affects primary productivity and the dynamics of other fishes that compete with guppies, with an effect on guppy demography. This eco-evolutionary loop involves the different levels of biodiversity mentioned in Figure 2, and set up over a few tens of generations.
Considering eco-evolutionary dynamics seems therefore relevant when analyzing the effect of global change on the time scale of a few decades. The duration of the overshoot period is certainly critical, since it will determine whether an evolutionary response can be mounted and then affect the aspect of global change (e.g., increased predation in guppies) that is at the origin of this response. This is further elaborated in Box 1, connecting environmental change and traits through the idea of adaptive/ecological peak tracking. It might also be that eco-evolution proceeds along a weaker version of loops, i.e. that the ecological driver of evolution is not in itself affected by the evolutionary response, but that other ecological factors are [96]. In the guppy example, it might well be that guppy populations have also been affected by climate change, but it is unlikely that guppy evolution impacts, at least directly, the climate.
The guppy example shows how eco-evolutionary coupling could be studied in practice, and we certainly lack similar examples in which the full eco-evolutionary loop has been considered [126, 96, 125]. However, this remains a “simple complex adaptive system”. Studies on much wider spatial (and impact) scales are required with presumably weaker loops and causalities, and a multitude of ecological and evolutionary factors at play—see Box 1 for climate change and Appendix 2 in Supplementary Materials for other stressors. Even if we know that evolution can serve in actions dedicated to the limitation of global change—we have good examples of evolutionary rescue of populations [127, 128], its overall impact at the planetary scale in the face of strong drivers such as climate change remains poorly understood from an eco-evolutionary perspective. Bridging this gap is critical for both predicting future dynamics and finding solutions to global change, issues that we will consider in the next sections.
5. Predictions integrating eco-evolutionary dynamics
The framework used above implicitly appeals to the notion of prediction, since the general idea is to understand how biodiversity will react to the multiple avatars of global change by projecting into the near future—the notion of overshoot (Figures 1 and 3) does indeed lead us to address the question in terms of predictions. Prediction is, in fact, a general question in science—sometimes associated with the very existence of a science, and the biodiversity sciences are no exception, whether ecology [129, 130, 131], evolution [132, 133, 38], or eco-evolution [134].
In a somewhat caricatural way, we will distinguish in the context of the present analysis two forms of prediction, namely explanatory vs. anticipatory ([129, 135]; see also [134] for a more complex classification). The former corresponds to the construction of knowledge about a given object, and can be built on the basis of different approaches, based on hypothesis testing or induction. For example, we may want to test the effect of temperature on the reproductive rate of a species, making the hypothesis that, up to a certain temperature, an increase in temperature leads to a higher reproduction rate. An experimental approach will enable us to evaluate this hypothesis, and quantify the impact of temperature, thus building up knowledge about the biology of this species. Of course, the knowledge built up by this approach is not absolute, and is subject to variation, uncertainty and therefore re-evaluation. It can also be considered as a proxy, when, for example, we assume that the temperature–reproduction relationship in the population studied can be considered valid for other populations from the same species or for those from closely related species.
Anticipatory prediction concerns the future, and is built on the consolidated knowledge derived from the explanatory approach. From this knowledge, it is possible to construct predictions. Taking the previous example, we can use the temperature–reproduction relationship to predict the reproductive rate of the species under consideration if the temperature increases by 2 °C (Figure 1). This prediction can be built from a variety of approaches, including narratives, scenarios, models or projections (see [136, 134, 135, 137]), depending on the objectives and amount of available information and based on one (or more) stressors supporting the projection—for example, a temperature rise or the arrival of an invasive exotic species. This is not a simple exercise, depending on the quality and quantity of data, or the uncertainties about the projected stressor (what temperature increase?). The strengths, weaknesses and difficulties of this approach are detailed in Mouquet et al. [135], Maris et al. [129], Elliott Graves [138] and Hendry [134], and beyond the scope of the current paper. Below we discuss only some aspects that are relevant to short-term eco-evolutionary predictions (Figure 1).
Even if they are associated with uncertainties, the Intergovernmental Panel on Climate Change routinely produces several scenarios for predicted temperatures, e.g., of the 21st century, including the functioning of geo-hydro-climato cycles (https://www.ipcc.ch/). Carrying out the same type of exercise for biodiversity is somewhat more difficult, since the aim is not simply to predict an environmental indicator such as temperature (with of course its uncertainties), but rather biodiversity per se (e.g., the distribution or demography of species, the arrival of invasive species) which itself depends on environmental parameters. Going back to Figures 1 and 3, we can see the parameters to be quantified as a basis for predicting biodiversity’s fate, and some of the difficulties that will arise: (i) the magnitude and dynamics of environmental variation. The IPCC scenario was used to set a target value (2 °C temperature rise); (ii) the duration of the overshoot, if it is not infinite (or very long); (iii) the dynamics of the return to “normal”.
Predicting the future of biodiversity is essentially based on ecological approaches that do not take into account the possibility of evolution. This is already an extraordinarily complex exercise [129, 135, 130, 131], particularly when multiple stressors affect biodiversity [139, 131]. A source of complexity, noted above, is that these stressors will affect a whole range of species, and give rise to new communities and ecosystems. Predictions are based, as mentioned above, on various approaches, but a massive appeal is made to models, for example to predict species distributions [140, 141]. These models can be correlational, for example based on the relationship between the distribution of a species and the temperature envelope corresponding to that distribution, or mechanistic, when we are able to integrate biological parameters, such as migration capacity, which will define the ability to reach favorable environmental conditions. It is even possible to use heuristic models, and an imprecise prediction approach [142], or even a combination of models. None of these modeling approaches is perfect with possible trade-offs between alternative models, as highlighted by Levins [143] in the early days of ecological modeling, nor do they necessarily answer the same questions.
Prediction must also incorporate the possibility of adaptation through evolution, particularly as species will often find themselves facing moving environmental conditions (Figure 3). This means including forces or characteristics such as standing genetic variation, mutation or phenotypic plasticity to track these peaks, and improve the level of adaptation—we saw earlier that this process can be rapid. Including the possibility of evolution will certainly add variance to species responses, and unpredictability. However, this contribution is rarely taken into account in anticipating and managing (see Section 6) the impacts of different global change avatars [132]. Evolutionary biology is indeed generally rather shy when it comes to predicting future trajectories [137, 133]. Day [144] even suggests, on the basis of an approach close to Gödel’s incompleteness theorem, that prediction in evolution is intrinsically limited.
The study of eco-evolutionary dynamics relies on both theoretical and empirical approaches. We developed here aspects that are of major interest with regard to prediction. From a theoretical point of view, evolutionary biology does have some tools at its disposal for making predictions of the kind required in Figures 1 and 3. The simplest approach might be to use narratives sensu Otto and Rosales [137], as a basis for mathematical models, or perhaps more simply, scenarios. More detailed approaches can be based on models. A widely used approach to build projections on the evolution of traits is quantitative genetics, generally deemed valid in the short term (a few tens of generations), but not beyond, due to the rapid temporal variation in variance and covariance structures between traits [133, 145, 111]. Furthermore, the approach is species-centric, and it seems difficult to generalize it to the community level (but see [146]). Another widely used approach is adaptive dynamics [147] which has for example been used to model the evolution of dispersal [148], but considers evolution on a time scale too long to be relevant in the context of global change. Lion et al. [149] recently proposed a hybrid approach that takes into account elements of both quantitative genetics and adaptive dynamics, which could enable medium-term predictions.
Prediction in evolution can also rely on empirical approaches. For example, Mallard et al. [150] suggests that phenotypic evolution can be predicted at 50 generations in a model species, the nematode Caenorhabditis elegans, even if predicting the evolution of given traits is difficult, for example because of genetic drift. Long-term monitoring is especially worthwhile in this endeavor, whether in the laboratory [99] or under natural conditions [103, 102]. Retrospective approaches are also possible, for example in the context of resurrection ecology, i.e. “the revival of long-dormant organisms via hatching of dormant life stages such as seeds, eggs, and spores/cysts” [151]. Comparing their traits (and fitness) with those of current entities allows to quantify evolutionary responses to stressors. For example, Acoca-Pidolle et al. [152] suggest that a plant, the field pansy, evolved higher selfing rates in response to pollinator decline, based on a resurrection approach (see, for a review, [153]). The next step is to move towards approaches that integrate both ecology and evolution to derive predictions [134], including all biodiversity levels, as we have seen above that eco-evolutionary approaches may give different results from purely ecological or evolutionary approaches. Such an eco-evolutionary approach can be integrated into schemes of future environmental variation of the type shown in Figures 1 and 3. The ecological and evolutionary issues raised in Box 1 should indeed be considered (e.g., peak tracking, adaptation to lower temperatures at the end of overshoot), but producing eco-evolutionary predictions comes with additional challenges. To cite just a few. The first is that we do not have enough work coupling ecological and evolutionary approaches, as mentioned above (Section 4), perhaps because this requires long-term approaches (several decades in plants and animals), which makes it difficult to build predictions taking into account all the associated pitfalls [134]. The second is that we should be clearer about whether or not evolution, which facilitates peak tracking during the overshoot period (Figure 3), will also drive biodiversity into trajectories that make adaptation to new conditions at the end of this period more difficult (i.e., evolutionary traps; [154]). Taking again the example of increased selfing rates with pollinator decline [152], one might ask how pollinators are affected if such an evolutionary trajectory is taken by many plant species, and how to integrate such a genuine eco-evolutionary loop into predictions. A third aspect are multiple stressors [52]—we have essentially used the example of climate change (Figure 1), but predictions in practical cases will often require considering it in connection with, say, invasive alien species. This means more complexity, and probably less precise predictions, opening up the issue of the kinds of predictions we need for action [134, 129, 135].
6. Implementing eco-evolutionary solutions to the biodiversity crisis
A wide range of engineering solutions have been proposed to cope with various aspects of the Anthropocene crisis (e.g., mitigation of stressor impacts, adaptation to their effects), but a good part, especially the grey ones (solutions involving artificial structures, such as dams against floods), can cause more problems than they solve, have only a short-term local impact, and do not address the future of biodiversity at all. More generally, it is unlikely that the human mind will “demiurgically” produce solutions to all problems through this type of approach [13], and that some form of “degrowth” (e.g., reduction of stressors) should not have to be achieved, coupled with nature-based solutions3 , to get actual positive results (see [25]). These approaches are nothing new, and have been developing for almost half a century in conservation biology [39] or ecological restoration [155], relying on concepts such as ecosystem services or, more recently, nature’s contributions to people [32], and considering focal species (as typically in conservation biology) or ecosystems (in restoration ecology). A huge literature has developed on these subjects, and we will not elaborate further. By definition (see footnote 3), nature-based solutions should return benefits to society, the economy and biodiversity [156, 157]—the novelty relies on the fact that solutions should be good for the targeted diversity (e.g., a species to be saved), but also for the untargeted one (e.g., saving the targeted species should not impact negatively the recipient ecosystem).
These approaches have been based on two pillars: the ecological sciences and the humanities and social sciences. As for prediction, the evolutionary principles had limited penetrance, with minor integration of the role of intraspecific diversity and adaptive potential, although their relevance has been highlighted in conservation biology [158, 36, 159, 160], in restoration ecology [161], but also in socio-ecological [31] or ecosystem services [162] thinking. In fact, all human interventions (direct or indirect) are evolutionary in essence, because they modify the adaptive landscape in which species are living, but this needs to be explicitly recognized and implemented. As Mr. Jourdain, Molière’s famous character from Le Bourgeois gentilhomme, eventually realized that he was speaking in prose without knowing it.
A first step would be for intraspecific diversity and adaptive potential to be taken into account less timidly in international discussions and regulations, for example for those sustainable development goals (https://sdgs.un.org/goals) involving biodiversity, as highlighted by Dìaz and Mahli [28]. It is encouraging to see that genetic diversity is mentioned in the Kunming-Montreal Agreement (COP15; see https://www.cbd.int/article/cop15-final-text-kunming-montreal-gbf-221222). Intraspecific diversity and adaptive potential also play a critical role in nature’s contributions to people [163], and probably in nature-based solutions, although their role remains to be formally evaluated. For example, moving individuals in assisted migration or species translocation programs means moving their genes, so a lack of concern for the genes being moved might have (negative) consequences, such as moving individuals that are not adapted to local conditions [164, 165]. Another example is genetic rescue of populations, which might, as already noted, be as important as demographic rescue, through the input of genes from outside [127]. The role of intraspecific diversity should thus be explicitly recognized, assessed and, if relevant, implemented. There is therefore wide room (and necessity) to include them in nature-based solutions. An eco-evolutionary approach would then involve taking into account both ecological and evolutionary processes/forces, and ideally evaluating possible eco-evo feedbacks.
Using again Figure 1 as a guide, such eco-evolutionary practices could be used to deal with the magnitude of the stressors (here, up to 2 °C), the overshoot period duration, and the dynamic of environmental targets. In practice, this means avoiding genetic and ecological drift (i.e., diversity loss), understanding the impact (strength and direction) of natural selection, which assumes quantifying the link between phenotype and fitness [110, 111], and eventually importing genes and individuals to cope with temperature increase, at least for overshoot duration. This also means considering the associated issues of local adaptation and genetic exchanges with local individuals [127, 128]. At the population level, population size and fragmentation are useful guiding indicators [36, 159, 166]. At the overshoot end, importing again new genes might be useful to cope with a come back to pre-overshoot temperature. Reasoning (and acting) at the community level is a further challenge, as intraspecific diversity should be evaluated and monitored in the target species (e.g., translocated), but also in the recipient communities—simply counting species in the latter will not be informative about their genetic diversity, given that diversities within and among species are not necessarily correlated [167, 122]. Both at the population and community level, it is quite clear that the fast advances in genomics over the last decade, including full genome sequencing and environmental DNA approaches, offer powerful tools for characterizing (epi)genetic diversity [159, 37, 168]. However, phenotyping is also critical and complementary to connect this diversity to the phenotype and fitness, and therefore to fast environmental change (Figure 3). The major challenge will then be to evaluate whether conservation or restoration actions drive the target species and ecosystems into virtuous eco-evolutionary loops, as again nature-based solutions should benefit biodiversity.
Implementing eco-evolutionary (nature-based) solutions to the biodiversity crisis is associated with major locks, especially when it comes down to managers and policy-makers (and the general public): (i) considering that appropriate biodiversity should be used in nature-based solutions, i.e. considering basic principles of ecology, such as adaptation to a given environment, is already a first challenge in a short-termist world [156, 157]. Considering intraspecific diversity goes a step beyond, because the role of this diversity is underappreciated, or even unknown, except perhaps for species of direct interests to humans (e.g., crops, pets) or for concepts such as consanguinity; (ii) Nature-based solutions require consensus and democracy, since they also aim at well-being and improved social contexts [169, 157]. On a large geographic scale, this means international cooperation … in a world that is not always eager to cooperate, even to deal with massive challenge such as climate change. For more local issues, discussion, acceptance of non-scientific sources of knowledge, and co-construction seem essential [163, 32, 157]. Such an approach would be possible if we had time ahead of us; however, the problems are already right in front of us, and we may have to accept the implementation of solutions based on uncertain predictions and limited scientific knowledge, as already mentioned.
7. Conclusion and perspectives
Although the Anthropocene has not been adopted as an era by the International Union of Geological Sciences [20], the human impact on the planet, its climate and its biodiversity is massive and multifaceted, and its magnitude is likely to increase further, as there are few signs that humanity is “putting the brakes on its activities”. The focus, quite rightly, on the climate crisis somewhat overshadows that of biodiversity, the main aspects of which we have outlined in this paper. Yet the biodiversity crisis is having a massive impact on human life, and in turn on various facets of the Anthropocene, starting with climate change. The various aspects of the biodiversity crisis, from its analysis to predictions and solutions, have been addressed by essentially ecological approaches—this is absolutely vital. However, biodiversity (including humans) continues to evolve, in the Darwinian sense, and it is therefore necessary to take evolutionary processes into account throughout this chain of work. My tenet in this article is that biodiversity should be considered from an eco-evolutionary perspective, while acknowledging the major role played by human activities. Biodiversity evolution can indeed feed back on ecology (including stressors), so an eco-evolutionary perspective is required, with its feedbacks and loops [95, 134, 125]. The notion of environmental overshoot (Figure 1) then provides a heuristic temporal perspective on the impact of the different avatars of global change, such as climate change (see also Appendix 2 in Supplementary Materials). The overshoot duration, whatever the environmental stressor considered, will indeed be long enough to require taking into account genetic diversity and evolutionary potential, even for long-lived species. This can be achieved by considering temporal trait variation and the factors responsible for their evolutionary dynamics, as represented in a very simple way in Figure 3. This academic exercise can set a basis to analyze the dynamics of biodiversity, but also to predict its fate and to implement solutions to the biodiversity crisis, for example in nature-based solutions.
In conclusion, I would like to highlight a few issues and problems: (i) Biodiversity is faced with a multitude of stressors, often acting synergistically, that need to be considered together [139, 170, 52]. An interesting proposal is to develop a “networked” and temporal vision of these effects (which are not necessarily the same if they occur in sequence or together, with possible transgenerational effects; [52]). This is consistent with the notion of overshoot, which includes the possibility of adaptation over dozens of generations. (ii) Integrating an evolutionary perspective is not easy, and even less so for an eco-evolutionary perspective—socio-economic anthropocentrism is a major obstacle, as is the disconnect between humans and “nature” [171]. Powerful socio-economic interests are at stake, which are pushing us not to act or to propose technological solutions that will/may pose more problems than they will solve in the near future [172, 25, 3]. It is essential to recognize the scale of the problems posed by global change for biodiversity, to discuss them democratically, and to try ad minima to behave decently towards biodiversity [173, 32, 25]—perhaps do what is possible, even if hopeless, as an ethical imperative [71]. Building a new pact with “nature” is certainly more ambitious, yet necessary (e.g., [171]), setting it on human resilience [24] and on transformative change, i.e. fundamental, system-wide reorganization, therefore calling for widely different socio-economic perspectives (https://www.ipbes.net/transformative-change). (iii) It is questionable whether the ecology and evolution research community is structured to tackle the problems posed by global change on biodiversity (see [35, 38]). It has been involved in the management of environmental problems for several decades, for example with the emergence of conservation biology in the 1980’s [39], and proposes applicable solutions integrating ecological and evolutionary processes [158, 36, 160]. However, research in these fields remains largely underfunded (see [38]), and is perhaps destined to remain so. The reason for this may lie in the fact that ecology and evolution are revolutionary sciences, in the sense that they have taught us two bad news: we are just one part of biodiversity, one ape among many [174], but a hyper-powerful and destructive ape that does not seem very concerned about the necessary stewardship of Earth. Yet an eco-evolutionary vision seems more necessary than ever to find solutions, in conjunction with other approaches, particularly socio-economic ones [31, 24, 12], and to map out a future less gloomy than the one we can envisage today.
Declaration of interests
The authors do not work for, advise, own shares in, or receive funds from any organization that could benefit from this article, and have declared no affiliations other than their research organizations.
Funding
PJ’s work is supported by CNRS-Ecologie & Environnement.
Acknowledgements
The author thanks T. Giraud and J.-D. Lebreton for inviting to write this paper with a wild card on the topics to be addressed, and E. Fronhofer, T. Giraud, P. Huneman, F. Viard, and an anonymous reviewer for important comments on the manuscript.
1 “Civilization is living now under a syndrome of too many people, with those in ‘developed’ parts of the world consuming an unfair share of Earth’s resources, and all together using an unsustainable fraction of our planet’s natural capital” [19].
2 Pollution or water use could also have been considered as well. The framework developed below could be adapted to them with no major change.
3 According to IUCN, nature-based solutions are actions to address societal challenges through the protection, sustainable management and restoration of ecosystems, benefiting both biodiversity and human well-being (https://www.iucn.org/resources/issues-brief/ensuring-effective-nature-based-solutions).