1 Introduction
The possibility to combine properties of organic, bio and inorganic components in a unique material is an old challenge starting with the beginning of the industrial era. Some of the earliest and most well known organic-inorganic representatives are certainly derived from the paint and polymer industries, where inorganic pigments or fillers are dispersed in organic components (solvents, surfactants, polymers…) to yield or improve optical and mechanical properties. However, the concept of ‘hybrid organic-inorganic’ materials exploded only very recently with the birth of soft inorganic chemistry processes (‘chimie douce’) [1] that shifted the research towards more sophisticated nanocomposites and original structures with higher added value.
Indeed, sol–gel chemistry [1] allows the combination at the nanosize level of inorganic and organic or even bioactive components in a single hybrid composite, providing access to an immense new area of materials science [2–10]. The development of innovative multi-functional advanced materials should have a major impact in future applications regarding several fields as optics, electronics, ionics, mechanics, membranes, protective coatings, catalysis, sensors, biology… [11,12,13].
Many interesting new materials have already been prepared with mechanical properties tuneable between those of glasses and those of polymers, with improved optical properties, or with improved catalytic or membrane based properties. For example, hybrid materials having excellent laser efficiencies and good photo stability, very fast photo chromic response, very high and stable second order non linear optical response, or being original pH sensors and electroluminescent diodes have been reported in the past five years [3]. Moreover, some hybrid products have already reached commercialisation [11,12,14]. Examples include the one million TV sets sold annually by Toshiba, the screens of which are coated with hybrids made of indigo dyes embedded in a silica/zirconium matrix [15], organically doped sol–gel glassware sold by Spiegelau [16] and sol–gel entrapped enzymes sold by Fluka [17]. Another application that demonstrates the interest of using multifunctional hybrids concerns sol–gel derived hybrid organic-inorganic coatings developed by several glass packaging or glass decoration industries in Japan and in Europe. These hybrid coatings (Fig. 1) cannot only give access to a wide variety of colours enhancing the consumer appeal, but they also improve the mechanical properties of glass bottles [18]. Moreover, these dye coloured bottles are easy to recycle as uncoloured glass (these materials do not need colour classification recycling), because, contrary to conventional glass bottles, their colorations do not arise from transition metals that are very difficult to remove upon re-melting. Finally, hybrid zirconia based nanoparticles precursors of nanomembranes, able to work under severe constraints (alkaline medium, T = 400 °C) are nowadays commercialised by Orelis-Rhodia [19].

Easy to recycle coloured glass bottles with improved mechanical properties covered by hybrid coatings, from [16, 18].
The nanostructure, the degree of organization and the properties that can be obtained for such materials certainly depend on the chemical nature of their components, but they also rely on the synergy between them. As a consequence, the nature of the interface or the nature of the links and interactions exchanged by the organic and inorganic components have been used to categorize these hybrids into two main different classes [2a]. Class I corresponds to all the systems where no covalent or iono-covalent bonds are present between the organic and inorganic components. In such materials, the various components only exchange weak interactions (at least in terms of orbital overlap) such as hydrogen bonding, van der Waals contacts, π−π interactions or electrostatic forces. On the contrary, in class II materials, at least a fraction of the organic and inorganic components are linked through strong chemical bonds (covalent, iono-covalent or Lewis acid-base bonds).
Thus, a key point for the design of new hybrids is the tuning of the nature, the extent and the accessibility of the inner interfaces. Another central feature in the tailoring of hybrid networks concerns the chemical pathways that are used to design a given hybrid material [5]. These main chemical routes are schematically presented in Fig. 2.
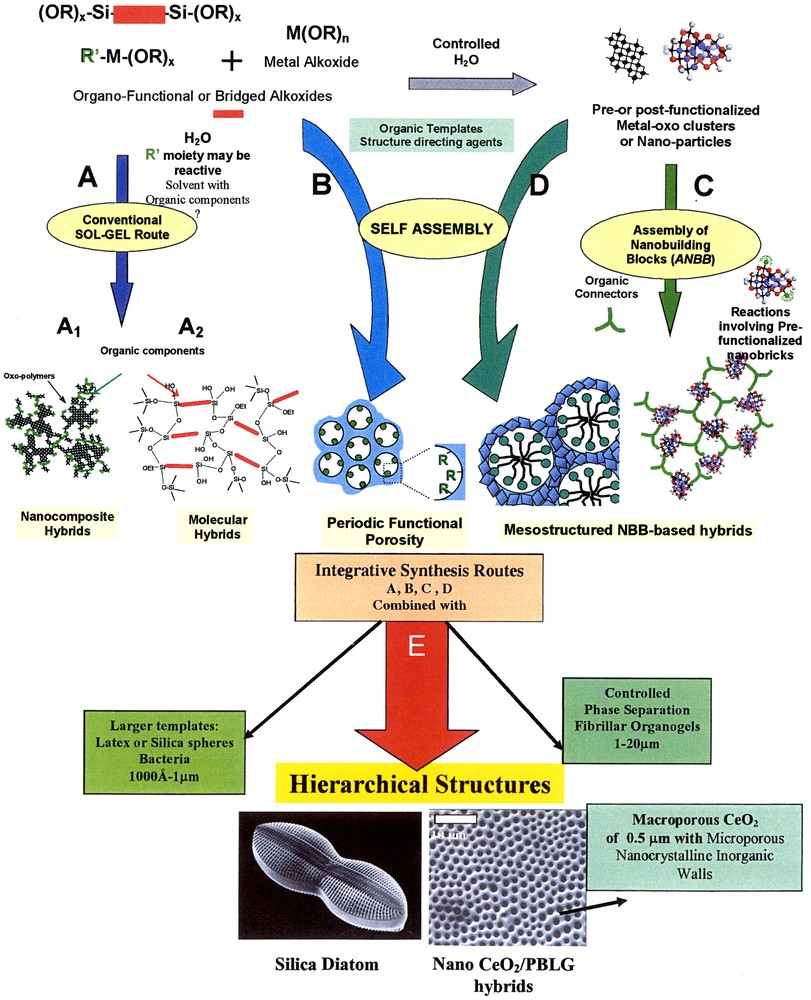
The different paths to obtain hybrid materials. A sol–gel routes (path A1 conventional route for hybrid nanocomposites) leads to hybrid (path A2: molecularly homogeneous hybrids). Path B involves the Assembly of NanoBuilding Blocks (ANBB), of prefunctionalised or post-functionalised clusters or nanoparticles. Route C or D involves the use of templates surfactant) capable to self-assembly, giving rise to organised phases. Path E involves integrative synthesis combining precedent paths from A to D and other processes: such as the use of lithography, casting, organogels or latex beads as templates controlled phase separations, or external fields.
Path A1 corresponds to conventional sol-gel chemistry. These hybrid materials and their main properties have been extensively reviewed and will not be discussed in this article [5,6]. Such hybrid networks are obtained through hydrolysis of organically modified metal alkoxides, (R′nSi(OR)4–n, R′nSn(X)4–n, M(OR)m–n(LZ)n, M being a transition metal, LZ a functionalised complexing ligand, 0 ≤ n ≤ m) condensed with or without simple metallic alkoxides. The solvent may or may not contain a specific organic molecule, a biocomponent or a polyfunctional polymer that can be crosslinkable or that can interact with the inorganic components through a large set of fuzzy interactions (H-bonds, π−π, VdW). These strategies are simple, low cost and yield amorphous hybrid nanocomposites. These materials that exhibit an infinity of microstructures can be transparent and easily shaped as films or bulks. However, they are generally polydisperse in size and locally heterogeneous in chemical composition.
A better understanding and control of the local and semi-local structure of these materials and their degree of organization are important issues, especially if tailored properties are sought for. Four main approaches may be conceived to achieve such a control of the materials structure; they are also schematised in Fig. 1.
- (i) Bridged precursors of silsesquioxanes. The use of bridged precursors of silsesquioxanes X3Si–R′–SiX3 (R′ is an organic spacer, X = Cl, Br, –OR) following the route A2 (path A2 in Fig. 2) allows to make homogeneous hybrid organic–inorganic materials [2c,7–10]. Moreover these materials through the interaction shared by the organic components (π−π stacking, between aromatic moieties or hydrogen bonding between urea derived functions, etc.) allow generating organisation within the solid hybrid network. This self-organisation process allows us to design materials with original macroscopic shapes inside which the chirality of the molecular precursor can be transcripted to the macroscopic level yielding helicoidal hybrid fibres [20].
- (ii) The assembling of well-defined nanobuilding blocks (NBB, route B). A suitable method to reach a better definition of the inorganic component consists in the use of perfectly calibrated preformed objects that keep their integrity in the final material. Such NBB can be clusters, organically pre or post functionalised nanoparticles, nano-core-shells [21] or layered compounds able to intercalate organic components. These NBB are generally capped with polymerisable ligands or connected through organic spacers, like telechelic molecules or polymers, or functional dendrimers (Fig. 2, route B) [5]. The use of highly pre-condensed species presents several advantages:
- • they exhibit a lower reactivity towards hydrolysis or attack of nucleophilic moieties than metal alkoxides;
- • the nanobuilding components are nanometric and monodispersed, and with perfectly defined structures, which facilitates the characterization of the final materials.
The variety found in the nanobuilding blocks (nature, structure, and functionality) and links allows one to build an amazing range of different architectures and organic-inorganic interfaces, associated to different assembling strategies. Moreover, the step-by-step preparation of the materials usually allows for a high control over their semi-local structure.
- (iii) Self-assembling procedures (routes C and D). Alternative approaches based on self-assembly (SA) may be conceived to also achieve a deeper control of the materials, in terms of the local and semi-local structure (i.e., the degree of organization). The ability to create ‘organised matter’ at the micro- and nanoscales has been indeed a significant breakthrough [6,22,23] since the discovery that micellar and lyotropic liquid-crystal phases can act as templates for periodic hybrid organic-inorganic materials [24].
Strategies combining the nanobuilding blocks approach with the use of organic templates that self-assemble and allow one to control the assembling step are also appearing (Fig. 2, route D). This combination between the ‘nanobuilding-block approach’ and ‘templated assembling’ will have a paramount importance in exploring the theme of ‘synthesis with construction’. As a result of this ‘Lego-like’ approach, it covers a complete spectrum of materials, from cross-linked organic phases to organically-textured inorganic networks [5].
- (iv) Integrative synthesis (Fig. 2, route E) [6,22,23]. The strategies reported above mainly allow controlling the design and the assembling of hybrid materials in the 1–500-Å range. Recently, micro-moulding methods have been developed, in which the use of controlled phase separation phenomena, emulsion droplets, latex beads, bacterial threads, colloidal templates or organogelators lead to control the shapes of complex objects in the micron scale [6,25]. The combination between these strategies and that described along Routes A B, C and D allow us to construct hierarchically organized materials, in terms of structure and functions.
These synthesis procedures are inspired in those observed to take place in natural systems for some hundreds of million years. Indeed, learning the ‘savoir faire’ of hybrid living systems and organisms from understanding their rules and transcription modes, could lead us to be able to design and build novel materials [26–28].
This short review will describe and illustrate different strategies to construct inorganic or hybrid networks shaped as thick or thin films, microparticles and fibres. In particular, strategies based on NBB, self-assembly approaches, organogels or latex templates and controlled phase separation allowing to tailor made nanostructured and/or microstructured inorganic and hybrid materials are presented, mainly on the basis of results obtained in our research group.
2 Hybrid structures built from nanobuilding blocks
The use of NBB as starting units to obtain hybrid organic–inorganic structures [4,5] is an approach developed with various systems such as oligosilsesquioxanes and derivatives [29], organotin-oxo clusters [4,5,30], organically functionalized heteropolyoxo-tungstates [5,31], transition metal oxo clusters [32,33] and finally with functionalized nanosized particles (metallic oxides, metals, chalcogenides) [21]. Such nanobuilding units are very versatile. Indeed, they exhibit a large variety of interfaces between the organic and the inorganic components (covalent bonding, complexation, electrostatic interactions…). These NBB with tunable functionalities can, through molecular recognition processes, permit the development of a new vectorial chemistry.
Some typical examples of organically functionalized NBB as starting units, belonging to polysilsesquioxane, organotin, polyoxometallates and metal-oxo clusters chemistry, which synthesis and structures have already been reviewed in several articles [4,5], are presented in Fig. 3.
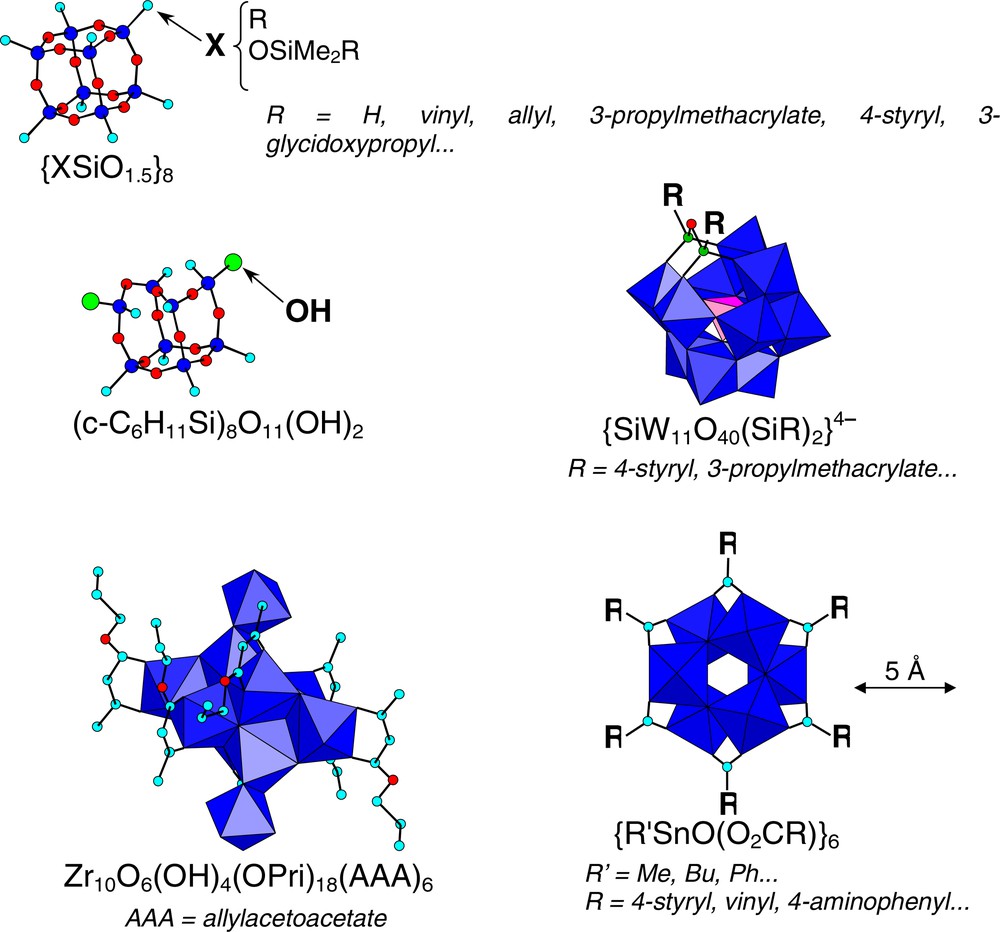
Various examples of oxo-clusters that can be used as NBB.
2.1 Hybrid structures built from tin based nanobuilding blocks
Sn shares with Si the stability of its bonds with sp3 carbon towards nucleophilic agents such as water. These bonds offer strong covalent links between tin oxo-polymers/oligomers and functional organic moieties, but they can also reduce the inorganic functionality of tin and favour the formation of oxo-clusters which can be used as nanobuilding blocks to design new hybrid materials [4,5,30].
Tin oxo-carboxylate clusters, such as {RSnO(O2CR′)}6 (R = methyl, phenyl, butyl and R′ being any organic function), abbreviated ‘Tin-6’, represent a family of nanobuilding blocks, in which the carboxylate groups can be used to provide the assembling functions [30]. The ‘Tin-6’ molecular structure (Fig. 3), where all tin atoms are six-coordinate (distorted octahedra), exhibits a hexagonal prismatic or ‘drum’ shape with the bridging carboxylate groups located on the six square faces of the ‘drum’. The ‘Tin-6’ cluster {BuSnO(O2CC6H4NH2)}6 exhibits on its periphery six amino functions amenable to subsequent reactions. It was functionalized, via the reaction with OCN(CH2)3Si(OEt)3, to yield quantitatively a dendrimer-like compound with 18 peripheral ethoxy functions amenable to hydrolysis-condensation [34]. It was also assembled via the reaction with 1,4-phenylene diisocyanate or 1,6-diisocyanatohexane [30].
Another interesting nanobuilding block is the oxo-cluster {(RSn)12(μ3–O)14(μ2–OH)6}2+, abbreviated ‘Tin-12’ [35]. Depending of the synthesis conditions the +2 positive charge is balanced by different anions (OH–, Cl–, R′SO3–, R′CO2–…), which are located at both cage poles, close to the hydroxy groups, which bridge the six-coordinate tin atoms. In solvents of low dielectric constant, no ionic dissociation is observed, but it takes place in solvents such as DMSO. The ‘Tin-12’ clusters are good and versatile nanobuilding blocks for the synthesis of well-defined tin-oxo based hybrid materials that can be used as models; possible synthesis strategies associated to this cluster are depicted in Fig. 4 [30,35].

Schematic representation of the various strategies that can be used to assemble {(RSn)12O14(OH)6}2+ into hybrid materials.
This cluster presents two different interfaces: the covalent interface provided by the Sn-C bond, and the ionic interface associated to the charge compensating anion X–. Each or both can be used to assemble such NBB. In one case, the organic moiety bound to tin should be polymerisable (e.g., R = butenyl, propylmethacrylate, propylcrotonate, 4-styryl…).
In the second case, charge compensating organic dianions can be used to bridge the clusters [4,30]. This was achieved by reacting {(BuSn)12O14OH6}(OH)2 with carboxymethyl terminated PEG macromonomers, in THF. Combining this strategy with embedded spirooxazines, new photocromic hybrid materials can be obtained. The photochromic behaviour of spirooxazines doped ‘Tin12-(COO)2-PEG’ hybrids is consistent with a preferred solvation of the dye moieties by the organic polymer host rather than by the tin-oxocomponent (see the cartoon in Fig. 5). The soft PEG component allows enough flexibility to avoid mechanical or steric constraints on the dye, and also allows high dye solubility. Moreover, the shielding of the polar ends of the cluster (carrying Sn–OH groups) by the carboxylate anions prevents stabilisation of the polar open forms of the dyes. As a consequence, the resulting hybrid layers with tuned structures and interfaces exhibit a fast photocromic response and a strong optical contrast provided by high dye content [36].

Proposed structure and synthetic pathway of a photochromic dye-doped hybrid network constructed from ‘Tin12’ NBBs crosslinked by telechelic poly(ethyleneglycol) (PEG), on the basis of 119Sn NMR, 13C CP-MAS, FT-IR and UV/Vis spectroscopy and DSC experiments.
The use of polymerisable anions offers an alternative strategy. Indeed, {(BuSn)12O14OH6}2+ can be functionalized with 2-acrylamido-2-methyl-1-propanesulfonate (AMPS), a highly polymerisable group. The homopolymerisation of such functionalized oxo-hydroxo butyltin nanoclusters leads to hybrid materials in which the nano-sized tin-based component is perfectly defined. {(BuSn)12O14OH6}(AMPS)2 can also be copolymerised with methylacrylate, in toluene, to yield gels. In line with the electrostatic interactions which are responsible of the cross-linking, these gels are soluble in DMSO [35].
2.2 Hybrid structures built from transition metal-oxo based NBB
The syntheses and structures of many high valence metals (TiIV, ZrIV, CeIV, NbV) or even heterometallic oxo-clusters have been described in the literature (see, e.g., refs [4] and [5]). Such species are usually prepared through the controlled substoichiometric hydrolysis of the metallic alkoxides M(OR)n or of the corresponding complexed alkoxides, M(OR)n–x(LZ)x, in solution. The full characterization performed by crossing XRD, FTIR and multinuclear NMR allows one to separately analyse the modifications of the metal-oxo core or the surface.
2.2.1 Hybrids from prefunctionalized transition metal-oxo clusters
Several clusters bear ligands containing an assembling function, in most cases, a polymerisable group, such as allylacetoacetate (aaa), methacrylate (OMc) or acrylate (OAcr) [4,5,32,37]. Examples of this approach are Ti6O4(OEt)8(OMc)8, Ti3O2(OPri)5(OCMe=CH2)3, Ti4O2(OPri)6(OAcr)6, Zr10O6(OH)4(OPri)18(aaa)6, Nb4O4(OPri)8(OMc)4, Zr6(OH)4O4(OMc)12, and Zr4O2(OMc)12. Their assembly through radical initiated polymerization must be performed in non-protic solvents (toluene, benzene or THF).
Following such an approach, inorganic-organic hybrid polymers were produced by radical polymerization of methacrylic acid or methyl methacrylate with (meth)acrylate-substituted oxozirconium or oxotitanium clusters Zr6(OH)4O4(OMc)12, Zr4O2(OMc)12, Ti6O4(OEt)8(OMc)8 or Ti4O2(OPri)6(OAcr)6 [32,37]. A very small amount of cluster was shown to provide an efficient cross-linking of the polymer chains. Small angle X-ray scattering experiments have evidenced that the cluster size is retained in the materials and that the microstructure of such hybrid materials can be described by a dispersion of spherical or disk-shaped clusters in polymers. Since depolymerisation reactions are inhibited, the resulting hybrid polymers exhibit higher thermal stability [32,37].
2.2.2 Hybrid assemblies of postfunctionalized transition metal-oxo clusters
A large number of oxo-alkoxo-clusters, MnOm(OR)z, have also been prepared from metal alkoxides, especially for Ti(IV) and Zr(IV). They can be used as model systems to understand the construction of hybrid materials, particularly at the inorganic-organic interface. There are two major synthetic challenges related to these clusters: their post functionalisation and the preservation of their cores. Both issues are related to the reactivity of the surface metallic atoms towards nucleophilic attack.
The stability of large clusters such as Ti12O16(OPri)16, Ti16O16(OEt)32 and Ti18O22(OBun)26(Acac)2 towards several nucleophiles (such as acetylacetate and derived compounds, or carboxylic acids) has been thoroughly discussed elsewhere [38,39], and it follows the sequence Ti18O22(OBun)26(Acac)2 > Ti16O16(OEt)32 >> Ti12O16(OPri)16 [39]. Once controlled, the reactivity of titanium-oxo clusters can be advantageously used to obtain new hybrid materials with tailored textures. Two main assembly strategies have recently been reported, based on the use of dendrimeric connectors [33,40] or amphiphilic block copolymers (ABC) as templates [40,41].
2.2.3 Ti16O16-Dendrimer hybrids
Meso organised transparent glassy xerogels can be obtained from a THF solution of Ti16O16(OEt)32 (TS) and first generation dendrimers upon drying. The dendrimers are functionalised on their surface, bearing acidic (G′1(COOH)6) or alcoholic (G′1(OH)6) tips [33]. Bicontinuous phases are obtained, formed by locally-ordered TS cores with correlation distances of ca. 2 nm. The path to these TS-dendrimer hybrids can be separated in three steps: (a) ‘snap-on’ reactions lead to hybrid bricks (‘hybricks’); (b) cross-linking of these hybricks upon solvent evaporation, giving rise to higher-weight species; (c) formation of a bicontinuous hybrid xerogel, in which TS clusters are regularly spaced by the polymers. The complementary tuning of the dendrimeric tips with respect to the inorganic surface can expand this Lego-like strategy to higher generation dendrimers and beyond clusters, aiming at organised arrays of nanoparticles (the structure of these TS-dendrimer hybrids is schematically presented in Fig. 6).

Mesotextured hybrid materials built through the ANBB of Ti16O16(OEt)32 (TS) and carboxylate-capped dendrimers. The TS clusters are regularly spaced by the polymers. The adequate combination of the surface chemistry of the clusters and the versatility of the dendrimeric tips permits to successfully obtain these tailored phases.
2.2.4 Ti16O16/ABC hybrid assemblies
Amphiphilic block copolymers (ABC), consisting of hydrophilic and hydrophobic blocks, are widely used as templates of mesostructured and mesoporous materials, particularly those based on PEO (poly(ethylene oxide)) and PPO (poly (propylene oxide)) blocks [39]. TS clusters have been used as nanobuilding bricks to create mesoscopically ordered phases using PEO-PPO-PEO triblock copolymers as templates [40]. A complex sequence of processes, involving the template, the solvent and incoming water, drastically affects the self-assembly and the mesostructuration of the hybrid phases. A strong interaction between the template and TS induces drastic modifications in the folding behaviour of the templating agent, leading to a decrease of the solubility difference between the copolymer blocks. This decrease promotes polymer unfolding, and a worm-like bicontinuous mesostructure is obtained [39,42]. Upon thermal treatment of these hybrid phases, high-surface worm-like titania (specific surface > 250 m2 g–1) is obtained [40].
On the contrary, hexagonal hybrid phases are obtained when PS-b-PMAA block copolymers and TS are used as NBB in toluene [41]. The templating agent consists of one polystyrene block (PS) and one polymethacrylic acid block (PMAA) with equal chain lengths (105 monomer units). The solvent plays a major role in this case. By selectively dissolving the PS block, spherical micelles are formed, the insoluble core of which is mainly the PMAA block. Once the titanium-oxo clusters are added to the micellar solution, their hydrophobicity leads to their incorporation in the PS corona. Some of them can diffuse into the amphiphilic interface between the PS block and the PMAA block, where complexation reactions between the carboxylate ligands and titanium centres takes place, generating a covalent hybrid interface. As a large excess of the block copolymer is added, only a fraction of the carboxylate groups belonging to the PMAA blocks are bound to the titanium atoms. The EtOH molecules released by the cluster upon complexation may react with the neighbouring –COOH groups, leading to esterification. Water is thus locally produced in a controlled way. This chain reaction may promote the further hydrolysis and condensation of the titanium–oxo clusters. The hexagonal mesophase obtained upon solvent evaporation is ‘freezed’. The formation of an inorganic framework covalently linked to the polymer matrix may enhance the stability of the aggregated structures [41].
2.3 Nanoparticule-based NBB and resulting hybrid structures
The stability problems experienced when dealing with small clusters can be overcome with larger species, such as nano-colloids. Consequently, the devices that are designed nowadays are mainly based on nanoparticles, ranging from 2-100-nm diameter. The development of novel and versatile methodologies that allow multiscale processing of nanoparticles has an outstanding impact on fundamental research and on chemical and biochemical engineering. Functionalized nanoparticles already play an important role, for example in pharmaceutical drug delivery systems, paint dispersion, tyre reinforcement, catalysis, and in many processes involving adhesives, biocements, varnishes and lubricants. Several physical methods such as MBE (molecular beam epitaxy), OMVPE (organometallic vapour phase epitaxy), mechano-synthesis, fast quenching of inorganic vapours, nanolithography, laser ablation or electrical ablation, allow one to make nanomaterials [21,43]. However, wet chemical routes should give cheap and versatile access to nanoparticles and allow us to produce nano-objects in larger amounts [21].
In principle, there are several ways to adapt the NBB approach to create nanoparticle-based hybrid materials. All these strategies have been shown above in the case of clusters. Again, the building of the hybrid structures requires a fine tuning at the hybrid interface level. The nanoparticles have to be functionalized in order to be compatible with the organic components of the hybrid; the two main approaches make use of pre- or post-functionalised nanoparticles, respectively. The resulting hybrid networks can be amorphous, nanostructured or exhibit long range ordering.
In this section, we will present some relevant examples of hybrid nanoparticle-based systems, using an NBB approach. An exhaustive review of the synthesis and nature of nanoparticle-based systems is beyond the scope of this presentation; the reader is referred to specific sources [5,21,44].
2.3.1 Synthesis of functionalized nanoparticles
An adequate organic functionalization of metal oxide nanoparticles allows one to design a large variety of new hybrid materials and devices. Chemisorption of organic chains on the particle surface stabilizes the nanoparticles, and can provide a functionality at the same time. A widespread method to produce colloidal dispersions is by means of coating the inorganic particles by Self Assembled Monolayers (SAMs). The hybridation of inorganic particles, via surface capping with organic functionalities can be performed in one step in situ during the particle synthesis or through post-functionalization of the already synthesized nanoparticles (Fig. 2, route B). The central point is to control the interfacial link between the metal species and the organic molecules.
Hydrolysis of metal alkoxides with d or p empty orbitals (Zr(IV), Ce (IV), Ti(IV), Sn(IV), Al(III)) in the presence of a complexing ligand, such as acetylacetone, and a non-complexing organic acid (p-toluene sulfonic acid), followed by ageing at 60–80 °C, leads to non aggregated sols of nano-crystalline particles, which size can be tuned between 2 and 4 nm [38,45,46]. Hybrid sols and xerosols made of nanocrystallites of tetragonal ZrO2, TiO2 (anatase), or SnO2 (rutile-like), can also be obtained. The surface of these nano-objects is protected by complexing agents (acetylacetonates, α- or β-hydroxy acids), which establish iono-covalent bonds with the particles, through favourable complexation equilibria with the metallic atoms located at the particle surface. The surface structure and the nature of the protecting belt have been carefully investigated for titania-based hybrid materials [38,45,46]. These hybrid nanomaterials present several interesting properties.
- (i) The hybrid sols are precursors of ceramic films and membranes after drying and calcination at 400 °C. Nanozirconia coatings have been processed as nanomembranes. TiO2 nanoanatase coatings exhibit photocatalytic properties. Sb-doped-SnO2 films present anti-static behaviour.
- (ii) The hybrid xerosols resulting from solvent removal are also soluble in organic or in polar organic solvents. Therefore, they can be easily redispersed in organic matrices.
- (iii) Functional ligands can be used to impart new properties. For example, nanocrystalline TiO2 (anatase 2–5 nm) with polymerisable capping groups can be obtained through one-pot synthesis by controlling particle growth with a bi-functional complexing ligand, bearing acetylacetonato- and pyrrol functions [47]. The functional ligand ensures protection of the nanocrystalline particles, prevents aggregation and permits to polymerise or copolymerise these nanocrystalline NBB by chemical or electrochemical methods. The chemical strategy used to produce these TiO2-polypyrrol nano-hybrids is presented in Fig. 7.

Scheme of the synthesis path for an anatase–pyrrol nanocomposite. A bi-functional ligand molecule, acac6py (inset) is used to obtain pyrrol-functionalised monodisperse nanocrystalline TiO2 (anatase) in one step; capping pyrrol functions allow further polymerisation of these nanobuilding blocks. The particles can be (electro)chemically assembled into polypyrrol–TiO2 hybrid nanocomposite films; the conducting polymer can be electronically modified, opening a new path for potential applications.
This method that can be extended easily to other couples of metal oxides/conducting polymers (e.g., ZrO2, SnO2 with polyaniline or polythiophene) is efficient to integrate semiconductor nanoparticles into a lightweight conducting polymer, opening an interesting path for potential applications (piezoresistive or conductive coatings, light patternable smart substrates, electrochromic, charge-storage and photovoltaic devices) [47].
2.3.2 Organically postfunctionalized nanoparticles
Although several methods are available to obtain nanoparticles, wet methods, using mono- or biphasic media are envisaged to be more suitable for large scale production. The produced particles can be post-functionalized on their surface in a subsequent step. This process, of course, strongly depends on the particle nature [21,48].
The surface of silica particles can be easily functionalized by reactions in organic media with alcoxysilane (R′–Si(OR)3) or chlorosilane (R′–SiCl3) species [49]. The links between the nanosilicas and the silylated functional organic moieties depend on several parameters, such as surface pre-treatment (water and silanol content), solvent, nature of the organic R functions, etc… However, the nature of the links and the mechanisms involved in their formation are still matter of discussion. Some organo-functionalized nanosilicas carrying R groups with alkylamino, alkyacrylate, alkylepoxy etc. functions have already been commercialised by Degussa and Clariant. The strategy developed for the hybridation of nanosilicas has been used for the functionalization of alumina, zirconia or titania nanoparticles with trimethoxysilylpropylmethacrylate, in order to design reactive ceramic fillers for PMMA-based composites [50]. Many other metal-oxide-based particles can be derivatized through the use of a functionalised complexing ligand. Carboxylate species are used to coat ferric oxide particles. TiO2 has been modified by different groups (F–COOH, F–acetylacetone, etc.). The adequate choice of the grafted F group permits to modify the tribological properties of nano titania (F = alkyl), enhance its photochemical response (dye sensitizer), or open new functionalities (alkylamino groups, polymerisable organic function). Smart methods for polymerizing MMA at the surface of nanometric titania particles have already been developed in the paint and polymer industry [5,51].
2.3.3 Multiscale ordering of functional colloidal nanoparticles
Multiscale ordering of functional colloidal nanoparticles is a powerful technique for the creation of macroscopic devices. This multiscale ordering can be performed via self-assembly processes or through controlled molecular recognition processes. The connection between complementary functionalized colloidal systems is addressed following three main strategies: electrostatic coupling, covalent or non-covalent binding.
2.3.3.1 Networks formed through electrostatic interactions
Mesoscopic structures of TiO2 anatase nanoparticles have been formed through self-assembly processes involving class-I hybrid composites made of multiply charged polytitanate anions and tetramethylammonium cations. These anatase nanocrystals can self assemble into highly ordered superlattices [52].
Another example is given by the assembly of gold particles covered by quaternary ammonium bromide salts (R4N+Br–). 2D and 3D organized systems have been observed, the separation between the objects being controlled by the length of R. Nanosized silica NBB functionalized with primary amines can also be coupled with a ‘counter NBB’ displaying a complementary surface [53]. The complementary NBB can be a gold nanoparticle, capped on its surface by thiol groups that carry alkylcarboxylic functions. By combining these two systems, simple acid-base chemistry induces an immediate charge pairing which results in the spontaneous formation of electrostatically bound mixed colloids. Ordered superlattices composed of nanosized semiconducting sulphides have been synthesized within lyotropic phases [54,55]. Hexagonal-packed arrays of nanocrystalline CdS, a ‘mineral copy’ of an (ethylene oxide)10-oleyl/water mesophase present periodicities ranging between 7 and 10 nm. This method has been extended to ZnS, Cd1–xZnxS and CdSe nanoparticles [54].
2.3.3.2 Covalent networks
Covalent binding programmed assembly can be simply designed by mixing two sets of NBB presenting surfaces functionalized with complementary reactive functions. Moreover, thiol-functionalized nanoparticles [56] are currently employed for assembling nanoparticles in symmetrically and spatially well-defined architectures.
A more sophisticated derivation is constituted by the so-called biomolecular route, which makes use of very specific interactions, as those found in antigen-antibody pairs, to induce assembling of NBB [57]. Streptavidin–biotin complexes are suitable for this purpose, due to their large energies of dissociation and high stability over a wide range of pH and temperature [58]. This strategy can also open a wide land of opportunities for making biologically programmed nanobuilding block assemblies, with clusters or nanoparticles. A DNA-based method has also been presented, where non-complementary DNA oligo-nucleotides are attached on gold particles by means of thiol groups. An oligonucleotide duplex with ‘sticky ends’, which are complementary to the two grafted sequences is subsequently added. The nanoparticles self-assemble into macroscopic materials, this assembly being reversible upon thermal denaturation [59]. Bacterial tubules also give way to templated hybrid silica structures [60]. These are actually bio-inorganic hybrid materials.
A possible future strategy proposed by S. Mann for the organization of complex architectures in solution would involve dispersions of different nanoparticles, selectively tagged with recognition sites that define its unique position in the nanoparticle-based superstructure to be assembled. As a consequence, nanoparticles would recognize and selectively bind each other upon mixing, yielding assemblies having a well defined typical length, and presenting a tailored positional architecture [57].
3 Templated growth of textured inorganic or hybrid materials with tailored porosity
Porous materials in forms of films, fibres, powders, monoliths or macrospheres are widely used in various domains such as in optic, electronic, chemical sensing, catalysis, separation, etc. This arises from the combination between accessible porosity, high surface area, and the wide physicochemical properties that solid matter can offer (mechanic, dielectric, optic, etc.). However, a precise control of the porosity during synthesis remained a challenge up to the last decade where the template process was introduced. This latter technique once more combines the sol–gel chemistry and the structuring effect of various templates such as surfactant mesophases [6,24], organogel molecular assembly [25,61], hard latex or ceramic beads [62,63] or even nano- or micro-domains created by spinodal phase separation [64,65]. The so-formed organic domains have dimensionality and self-arrangement that dictate the final porous structure of the inorganic network counterparting in the hybrid system. A control at three levels is necessary to achieve such a control on the structure [6]:
- (i) A perfect tuning of the initial solution chemical composition allows us to select the wished stoichiometry (volume fraction of organic phase), to control the reactivity (hydrolysis-condensation rates), and to adjust preferential interactions at the organic/inorganic interface when segregation and self-assembly take place (relative solubility of organic, inorganic and solvent phases).
- (ii) The destabilisation of the initial solution leading to the intermediate hybrid material can be provoked through chemical (i.e. precipitation, gelation) or physical (i.e. evaporation, temperature) solicitations. This step is the most delicate one since phase segregation, self-assembly and condensation must occur in this exact order in order to end up with the desired architecture.
- (iii) Finally, the present as-prepared hybrid materials have to undergo a treatment aiming at creating the organised porosity by removing the organic phase, and at stiffening the inorganic network in the desire solid state. Specific treatments (thermal, UV/O3, solvent extraction…) can be applied to keep the inorganic phase or the functional hybrid phase within the matrix if needed.
So far, such nano-structuring approach has been well developed for pure or mixed metal oxide inorganic networks [6] such as SiO2 [66,67], Al2O3 [68], or transition-metal oxides [69,70,71], but will with no doubt be extended in the future to non-oxide materials such as metals or polymers [71a]. Already, pure platinum [72,73] and carbon graphite [74] meso-networks were prepared by an indirect nanocasting technique that involves impregnation of the porous network with salt or polymers, solidification through reduction or calcination respectively, and dissolution of the primary network.
The best studied mesoporous materials (e.g., the MCM and SBA series) have been prepared mostly by precipitation methods. However, mesostructured materials have been generated by performing inorganic condensation in lyotropic liquid crystalline phases, the so-called True Liquid Crystalline Templating (TLCT) [75]. These hybrid organised phases can also be produced after solvent evaporation from dilute solutions (Evaporation-Induced Self Assembly, EISA [76]). These alternative methods present several interesting points: the control of the inorganic condensation is easier (thus, transition metal systems can be explored), and the resulting materials can be processed in the shape of films, gels, powders, aerosols or fibres [6,77]. The following examples illustrate the wide possibility associated to the present direct templating and self-assembly.
3.1 Meso-organised materials prepared by Evaporation Induced Self Assembly (EISA)
In such a method, an initial solution (containing inorganic or/and hybrid precursors, surfactants (at C<CMC), water, solvent and catalysts) is rapidly or progressively concentrated in non-volatile species [78]. Eventually surfactant free molecules start to segregate into well-defined spherical, cylindrical or lamellar nano-domains (micelles) and ideally self-assemble into mono-, bi- or tri-dimensional mesophases. The great advantage of the EISA technique over classical precipitation routes lies in the shaping of the material at the macroscopic scale. Indeed, thin films can be prepared by spin casting or dip-coating, spherical particles are easily obtained by spray drying using an aerosol generator, large monoliths are obtainable by controlled evaporation inside shaped moulds.
Fig. 8 illustrates the wide potential meso-structuring that can be obtained when dip-coating alcoholic solutions containing hydrolysed alkylorthosilicate or metal chloride salts together with ionic or amphiphilic surfactants in proper conditions. For better clarity, TEM pictures are accompanied by the corresponding 2D-SAXS pattern and model. Structures (a), (b) and (c) respectively correspond to the body centred cubic (Im3m) [79] with discrete pores, the disordered worm-like (WL) or diffused phase, and the well-known hexagonal packing of cylinders (p6m). These three structures are generally obtained with silica [80] and non-silica oxide networks textured by amphiphilic PEO-PPO-PEO triblock copolymers (Pluronics) or CnH2n+1PEO diblocks (Brïj) [70,81]. Structures (d) and (e) are tridimensional hexagonal (P63/mmc) and cubic (Pm3n) only encountered with the ionic cethyltriethylammonium bromide (CTAB) surfactant [82,83]. The latter structuring agent does not provide good organisation with non-silica inorganic phase but can lead to structures (b), (c), (d) and (e) when combined with hydrolysed tetraethylorthosilicate (TEOS) depending on the chemical and processing conditions. The self-assembly mechanism involved in the formation of such nanostructured hybrid thin films has been deeply studied by in-situ SAXS investigation during evaporation [80,84,85]. It confirmed that the self-assembly is governed by four main parameters that are the morphology of the surfactant molecule and its packing parameter (g) related to the micellar curvature radius, the inorganic to organic fraction, the condensation extend of the inorganic part [82] and the relative partial pressures of each product present in the environment [86].
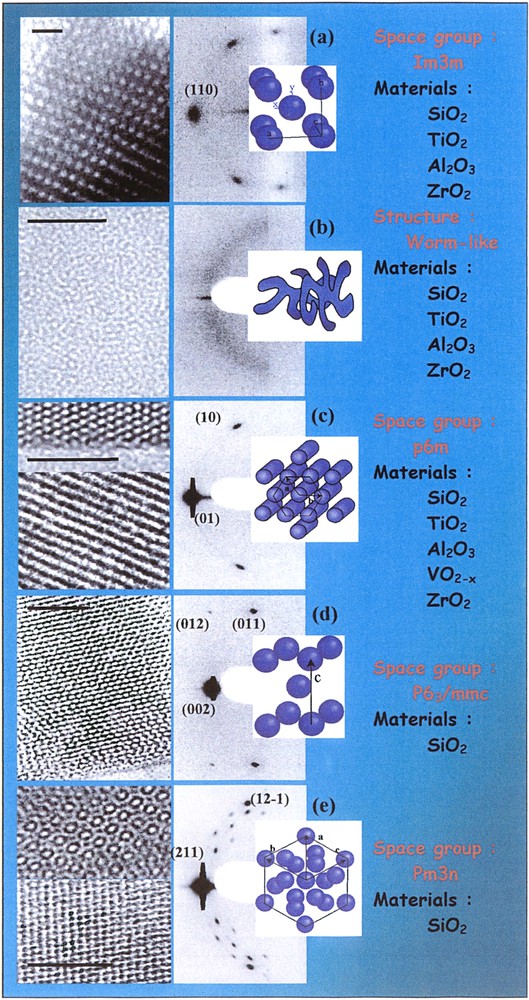
Various structures obtained as thin mesoporous films by the EISA method (scale bar represents 50 nm).
The careful control of these chemical and processing parameters allow us to synthesize reproducibly many periodically organised mesopropous inorganic films, membranes and powders not only with silica [74] but also with TiO2 [39–42,87,88], ZrO2 [89], VO2–x [90], Alumina [91], ZrO2–CeO2 and ZrO2–Y2O3 [92] and other binary oxide SiO2–MO2 [93]. A very important result concerns the thermal stability of the cubic phases of mesoporous titania and ZrO2–CeO2 ZrO2–Y2O3 that retain porosity and organisation even after nanocristallisation of the oxide up to temperatures as high as 750 °C. A beautiful example of mesostructured titania-based films with cubic structure is presented in Fig. 9.

2D-SAXS (left) and TEM image (along a [110] axis, scale bar = 100 nm) of a cubic (Im3m) mesostructured TiO2 film obtained by EISA, using block copolymer (PEO–PPO–PEO) templates. A slight uniaxial contraction is evident in the SAXS pattern, which is due to film shrinking.
Because this EISA route is regarded as the more convenient method to precisely adjust the chemical stoichiometry, to control the spontaneous organisation at the nanoscale, and to adjust the shape of materials at the macroscopic level, it has been very recently extended to the preparation of highly meso-organised porous spheres of controlled diameter by spray drying [78,94]. The same SA mechanism applies for aerosol and for films, except that the spherical initial droplets are in motion in the laminar gas flow and the interface of evaporation is curved. The potential influence of these additional parameters is on the way to be assessed. Fig. 10 displays various examples of organised SiO2 (a) and (b) and TiO2 (c). These latter materials are of great interest as they present adjustable porosity at multiple length scales (i.e. microporosity of primary entities, mesoporosity created by the surfactant nanosegregation, and macroporosity of sphere interstices). Any system that can be textured in thin films can therefore be process as spheres through this process. The versatility of the technique allows to combine surfactant micelles with latex spheres templates. It results in multi-scale porous materials where both meso- and macropores are precisely tuned. They are therefore future candidates for heterogeneous catalysis, chromatography, controlled drug delivery reservoirs, energy transfer devices, etc. This latter application additionally requires the inorganic network to be composed of crystalline anatase nanoparticles of less than 10 nm in size. This is achievable with the present system by using a careful sequence of thermal treatment that was also previously developed for thin films. Fig. 10c is a typical example where an organised meso and macro porosity coexists within a stable network of anatase nanoparticles.
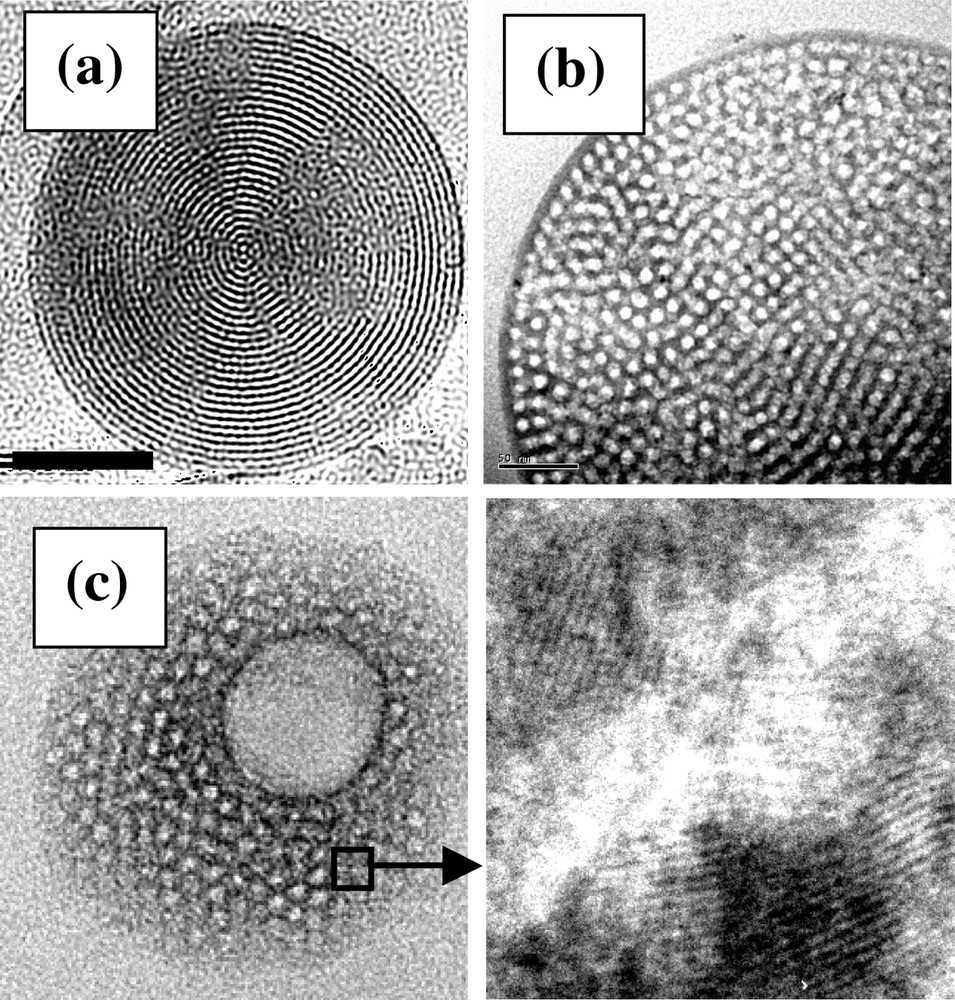
TEM images of spherical aerosol generated mesoporous macroparticles of SiO2 (a) and (b) and anatase TiO2 (c) (scale bar corresponds to 50 nm).
3.2 Modification of mesoporous network
The surfactant template approach can also be use to functionalise material with a selected active group (R) [95] following a one-pot synthesis, or to homogeneously post-incorporate functions or nano-objects within the network through post impregnation. The following examples have been developed in our group.
- (i) One-pot functionalisation is possible by using a fraction of non-hydrolysable organosilane precursor (R–Si[OR]3) with a Si(OR)4 silica source. For example, hydrophobic moities carried by fluoro phenyl groups (C6F5(CH2)2–Si(OR)3) can be incorporated to build 2D hexagonal mesoporous hybrid silica [96]. During self-assembly, the hydrophobic group R tends to place itself at the organic/inorganic interface. Once the surfactant is removed by solvent extraction and the network stabilised by a chemical route, the R group remains covalently bonded to the pore surface and is accessible through the porosity.
- (ii) Organic functionalities (pH probes, hydrophobic, electrochemical probes, etc.) can also be grafted onto the transition metal oxides walls (zirconia or titania) by using complexing ligands (carboxylates, beta-diketonates, phosphonates…), leading to hybrid mesostructured materials, with tunable surfaces opening a land of opportunities for designing new devices, catalysts, membranes, sensors and nano-reactors [89].
Magnetic nanoclusters, such as [Mn12O12(RCOO)16(H2O)4] or [Cr12O9(OH)3(O2CC(CH3)3)15] that behave as nanomagnets for information storage at molecular levels, have been successfully incorporated into silica mesoporous materials presenting 2.5 to 6 nm in diameter organised pores non functionalised or prefunctionalised with ethylenediamine-triacetic acid [97]. The optimisation of the filling depends on pore size but in all case the properties of the magnetic nano-guest is preserved.
3.3 Texturation by organogelators
Organogelators are low-weight organic molecules that are able to form thermoreversible physical gels, exhibiting strongly anisotropic structures (i.e. fibres, ribbons, platelets), at very low concentrations (~1% weight) in a great variety of organic solvent. Combining this new family of texturing agents (with steroidal or diaminocyclohexane skeleton, 2,3-bis-n-decyloxyanthracene (DDOA) or 2,3-Di-N-alkoxyphenazine (DAP)) with the sol-gel chemistry has recently permitted to direct the condensation of silica into original shapes at the microscopic levels. The first work were performed by the group of Shinkai [25,98]. They obtained hollow silica tubes, presenting an aspect similar to the lamellar-like patterns observed in spiculae, from the cholesteryl organogelators derivatives, and they succeed in the transcription of the chiral information into a mineral network. Indeed isolate left or right handed helical silica fibres by the simple templating of silica polymerisation with diaminocyclohexane organogelators derivatives carrying the adequate (R or S) configuration [98d].
In our group for instance, DDOA (2,3-bis(n-decyloxy)anthracene) has been successfully employed as template for silica – [61,99] and alumina [100] – based networks. Indeed, fibrous silicas and aluminas with tunable mesoporosity can be obtained. The TEM and SEM characterisation shows that submicronic fibres (200–500 nm) aggregate into fibrous bundles having bimodal porosity. Moreover the fibrous texture of alumina can be preserved up to 800 °C.
Organically modified functionalised hybrid fibres with accessible and modifiable functionalities have also been synthesized from silicon alkoxides and organosilanes DDOA based organogels as templated (Fig. 11). This strategy is very flexible and allows versatility because in a first step the fibrous hydrid network can be grown, condensed and stabilized with common functionalities and then in a second step post functionalisation can be performed with any other inorganic complex or even with fragile organic or bio functions [99].

SEM images of alumina (left, [99]) or hybrid silica (right, [100]) xerogels templated with DDOA organogelators (inset) showing the fibrous textures.
Recently novel organic–inorganic hybrids that present helical symmetries have been obtained by Moreau et al. through the hydrolysis of organosilica derivatives bearing an R,R or S,S chiral diureidocyclohexane spacer. Left- and right-handed helixes are self-generated depending of the configuration of the chosen organic sub-unit [20].
3.4 Texturation through phase separation
Spinodal phase separation occurs when a binary system containing two phases with limited solubility is progressively concentrated. When both phases become immiscible, domains of one pure phase starts to form homogeneously within the system leading to the so-called spinodal phase separation in which domain size, morphology and dispersion depend on the rate and extend of specie concentration and their diffusion in the present medium. If this phenomenon takes place in presence of a condensable inorganic phase, texturation becomes possible. Original and homogeneous macrotextures shaped with coral-like, helical or macroporous sieves morphologies have been obtained following a nanotectonic approach based on the template-directed assembly by poly-γ-benzyl-l-glutamate (PBLG) of organically functionalised CeO2 crystalline nanoparticles. By adjusting a single parameter, such as the template to inorganic ratio, a versatile tuning between templating effect and phase separation yields hierarchical porous materials presenting both micro and macro porosity with inorganic walls constituted of nano-crystalline cerium oxide particles [65] (Fig. 12).

a. CeO2 macroporous network obtained by spinodal phase separation with PBLG. b. Interactions between PBLG and functionalised nanoparticles. Adapted from [65].
4 Conclusion
Hybrid organic-inorganic materials are increasingly taking their position in the free spaces left between inorganic chemistry, polymer chemistry, organic chemistry, and biology. This land of research, initially worked out by the sol–gel community is at present thriving with the appearance of a new class of mesoscopic hybrid structures engineered from the molecular to the nanometric or micronic scales, to satisfy the requirements for a variety of applications from biological and chemical sensing, catalysis, selective separation to optical communications.
Outlooking to the 21st century, nanosciences will be, as biology, one of the fields that will contribute to a high level of scientific and technological developments. Hybrid materials present the paramount advantage to both facilitate integration and miniaturization of the devices (nanomaterials, nanotechnologies) and afford a direct connection between inorganic, organic and biological worlds. Functional precursors or functional nanobuilding blocks facilitate integrative synthesis pathways, where synergistic assembling and morphosynthesis can be strongly coupled. The chemical strategies offered by coupling soft chemistry processes with different macro templates (latex, bacteria, polymers, topological defects in LC…), self-assembly processes, and external fields will allow, through an intelligent and tuned coding, to develop a new vectorial chemistry, able to direct the assembling of a large variety of structurally well defined inorganic network into complex architectures. The research of multiscale structured hybrids (from nanometre to the millimetre) will open a land of opportunities for designing new materials. The future of this unifying field of research mainly depends on skills of all kind of chemists and is only limited by their imagination.