1 Introduction
Drug discovery from marine natural products has enjoyed a renaissance in the past few years. Indeed, pharmacologically active compounds from plants and microbes represent an important pipeline for new drug investigations. The basic scientific research in chemistry and pharmacology of marine natural products as well as efforts directed at drug development began in the 1970s. Several antitumor marine natural products, derived mainly from marine green algae, and cyanobacteria, exhibit potent antimitotic properties. Not all biologically active natural products are suitable for clinical use, owing to the problems of solubility, bioavailability, toxicity or ineffectiveness against resistant organisms, making preparation of their analogues important in drug discovery efforts.
An enormous number of chemically distinct molecular species arise from the various combinations of fatty acids with backbone structures (e.g., glycerol and glycolipids, etc.). However, it is not intuitively clear why nature has created so many different forms of lipids. As Professor Konrad Sandhoff has demonstrated, the understanding of glycolipid turnover was principally in the realm of biochemistry. However, glycolipids are critical components of cell membranes and occur within newly described membrane domains, known as lipid rafts. Glycolipids are components of important antigen systems and membrane receptors; they participate in intracellular signaling mechanisms and may be presented to the immune system. As components of cellular receptors for microbes and their toxins, glycolipids are also of worldwide importance (Figs. 1 and 2).

Chemical structure of natural SQAG's (R = Fatty acyl chains).

SQAGs time line.
Glycolipids play certain physiological roles, such as the regulation of protein kinase activity and inhibit tumor cell growth. Microalgae are a very good source of glycerolipids. Algae represent valuable sources of a wide spectrum of complex lipids with different potential applications, especially in the pharmaceutical industry. They are beneficial to the treatment of cancer. Negatively charged sulfo-glycolipids are part of the important group of non-volatile lipids that occur in higher and lower organisms. Sulfo-glycolipids are present on the surface of a variety of cells.
Sulfoquinovosylacylglycerols (SQAGs) are considered as potential candidates for further medical investigations.
The sulfolipid SQAG of higher plants and other photosynthetic organisms is characterized by its unique sulfonic acid head group, a 6-deoxy-6-sulfo-glucose, referred to as sulfoquinovose, a derivative of glucose, in which the 6-hydroxyl is replaced by a sulfonate group. Due to its strong amphipathic character, this anionic lipid is commonly thought to have excellent detergent properties [1] that may explain its apparent antiviral and antitumor activities [2,3]. It has been estimated that SQAG is one of the most abundant sulfur organic compounds in the biotic world, since the discovery of SQAG [4] and the elucidation of its structure by Benson et al. [5] over 30 years ago. Glycolipids, as the name implies, consist of two distinct moieties: a backbone lipid, which is hydrophobic, and a carbohydrate, which is hydrophilic. SQAGs are sulfo-glycolipids originally derived from sea urchins [6] and are also found naturally in higher plants and sea algae [7]. They fall into two groups according to the number of fatty acids attached: sulfoquinovosyldiacylglycerol (SQDG) and sulfoquinovosylmonoacylglycerol (SQMG). Even though two isomers are structurally possible, natural products include only the α-isoforms. We can now chemically synthesize most types of SQAGs. Both are valuable antitumor promoters in the biotic world, and are potent inhibitors of deoxyribonucleic acid (DNA) polymerase. Because DNA polymerases are essential enzymes for DNA replication and repair and subsequent cell division, the inhibition of these enzymes will lead to the death of tumor cells, especially under active proliferation conditions. Sulfoquinovosyl diacylglycerol exhibits the inhibition of growth on the human colon adenocarcinoma cell DLD-1 and on the human gastric cancer cell SNU-1 as well as apoptosis on SNU-1. Inogenosis has inhibitory activities in mouse skin papilloma as well as Epstein–Barr virus early antigen. In addition to their antiviral activity, their influence on the immune response in mammalian cells is the focus of many studies shown to play an important role in several biological processes. Recent findings showed that SQAGs act mainly as mammalian DNA polymerase inhibitors, antiangiogenic agents, rediosensitasors, HIV-reverse transcriptase inhibitor, immunosuppressive agents, and many more.
2 Occurrence and abundance
2.1 Photosynthetic organisms
The most abundant membrane lipids in plants are glycoglycerolipids, which constitute about 75% of the total membranes lipids in leaves. In Fig. 3, sulfoquinovosyl diacylglycerol (SQDG) and sulfoquinovosyl monoacylglycerol (SQMG) are the major glycolipids found in higher plants, where they represent up to 50% and 20% of the chloroplast lipids, respectively. Diglucosyl glycerides with various chain lengths have been widely studied since they are easily obtained from natural sources.

Sulfoquinovosyl diacylglycerol (SQDG) and sulfoquinovosyl monoacylglycerol (SQMG).
The occurrence of SQAGs [8–10] as the most important representative sulfoglycolipid could be proved in the microalgae Nitzschia alba [11] Pavlova lutheri [12], Chlorella vulgaris [13], Heterosigma carterae [14] and Porphyridium cruentum [15]. It is generally accepted that sulfolipids SQAGs are present in most of the higher plants, mosses, ferns, and algae. This assumption is based on an extensive survey of different photosynthetic organisms following the discovery of sulfolipid SQAG, as summarized by Haines [16]. More recently, a large number of marine algae have been shown to contain SQAGs [17–19]. However, it should be emphasized that the identification of SQAG was rarely based on a rigorous structural analysis but, rather, on cochromatography with standards. As summarized by Heinz [20], some marine algae contain more than 40 mol% of SQAG in lipid extracts compared with roughly 4 mol% found in lipid extracts of higher plants. Fractionation of sub-cellular membranes from higher plants reveals that SQAG is exclusively associated with plastid membranes [21], while there seems to be a tight correlation between the presence of SQAG and the capability to perform photosynthesis in plants; many photosynthetic bacteria lack SQAG or may contain it only in small amounts. Thus, a large number of cyanobacterial species isolated from different habitats contain approximately 10 mol% SQAGs [22–24]. However, according to a recent report, the cyanobacterium Gloeobacter violaceus sp. PCC7421 lacks SQAGs [25]. Among the anoxygenic photosynthetic bacteria, there are at least two genera, Ectothiorhodospira and Heliobacterium, that are completely devoid of glycolipids and, thus, of SQAGs [26,27]. Within the purple non-sulfur bacteria, some species like Rhodobacter sphaeroides contain SQAGs, as confirmed by the extensive structural analysis [28,29], while only traces of SQAGs can be detected in the closely related species Rhodobacter capsulatus [30] and no SQAGs seems to be present in Rhodopseudomonas viridis [31]. Furthermore, there is some uncertainty about whether SQAGs or a structurally related sulfolipids are present in photosynthetic bacteria of the family Chromatiaceae and the green sulfur bacteria [32].
2.2 Non-photosynthetic organisms
Another widespread but erroneous assumption has been that “the occurrence of SQAG is restricted to photosynthetic organisms”. The recent identification and complete structural elucidation of SQAGs isolated from the non-photosynthetic bacterium Rhizobium meliloti [33] clearly demonstrate beyond any doubt that SQAG is present in some non-photosynthetic organisms. However, it should be pointed out that rhizobia are closely related to photosynthetic purple non-sulfur bacteria [34] and that there are even photosynthetic species of rhizobia [35]. Thus, it may be more surprising that SQAGs are apparently present in a Gram-positive extreme thermo acidophilic non-photosynthetic bacterium of the genus Alicyclobacillus, formerly called Bacillus acidocaldarius [36]. The identification was accomplished by cochromatography with authentic standards, IR spectroscopy and isotope labeling, leaving little doubt about the fact that SQAGs are, indeed, present in this bacterium. Moreover, there is a report on the presence and function of SQAG in eggs and sperm cells of the sea urchin, Pseudocentrotus depressus [37]. Moreover, in R. sphaeroides, novel glycolipids, as well as a betaine lipid previously thought to be present only in algae and lower plants, are newly synthesized under phosphate limitation [38]. It should be also pointed out that there is an inverse relationship between the abundance of the two anionic lipids phosphatidylglycerol and SQAGs so that the total amount of anionic lipids remains constant under optimal and phosphate-limiting conditions. It has been recently discovered that phosphate availability also affects the membrane lipid composition in higher plants. Seedlings of Arabidopsis thaliana grown on agar-solidified medium with decreasing concentrations of inorganic phosphate showed similar changes in the lipid composition, as observed for the cyanobacterium Synechococcus [39].
3 Historical background
About the discovery of SQAGs, one can say that the history of science tells us that great discoveries often follow moments in time when technology has made a crucial leap forward. It is in the nature of the interdisciplinary meetings that, in the words of Sir Richard Burton: “Truth is the shattered mirror strewn in myriad bits; while each believes his little bit the whole to own”. Can we bring these myriad bits together here? Perhaps we have had some success since, as outlined by the title, “SQAGs: A stepping stone in biotic world”, the conceptual bridges are already built.
4 Synthesis
During our search for new glycoglycerolipids active in cancer chemoprevention, our research group has synthesized in recent years a number of esters of 2-O-β-d-glycosylglycerols in which the length, shape, number and position of the acyl chains, and the types of sugar (a- and b-glucose or galactose) were varied. These compounds were very active in inhibiting the tumor-promoting activity of 12-O-tetradecanoylphorbol-13-acetate (TPA) both in in vitro and in in vivo tests, such activities being mainly influenced by the changes in the acyl chains length [40].
Thus, the above-reported promising biological activities of SQDG prompted us to plan an easy synthesis of SQDG analogues based on the skeleton of 2-O-β-d-glucopyranosyl-sn-glycerol to which the previously tested glycoglycerolipid analogues were related and to test their antitumor-promoting activity. Starting from the known 2-O-(2,3,4,6-tetra-O-chloroacetyl-β-d-glucopyranosyl)-sn-glycerol [41], SQDG analogues 1a–c (Fig. 3), namely 1,3-di-O-octadecanoyl (1a), -dodecanoyl (1b), and -hexanoyl-2-O-β-d-sulfoquinovopyranosyl-sn-glycerol (1c) (in which acyl chains of different length were linked to the primary hydroxyls of 2-O-β-d-sulfoquinovopyranosyl-sn-glycerol) were prepared, exploiting chloroacetyls to temporarily and selectively protect glucose hydroxyls with respect to glycerol. As a preliminary checkup of their biological behavior in our paper [42], the obtained compounds were tested as antitumor promoters using a short-term in vitro assay for EBV-EA activation and to get an insight into the influence exerted by the 6′-sulfonate, the corresponding 6′-hydroxy synthetic intermediates, 1,3-di-O-octadecanoyl (4a), -dodecanoyl (4b), and -hexanoyl-2-O-β-d-glucopyranosyl-sn-glycerol (4c), were also tested. The Epstein–Barr virus (EBV) is known to be activated by tumor promoters to produce viral early antigens (EA), and an evaluation of its inhibition is often used as a primary screening for in vitro antitumor-promoting activities [43]. The inhibitory effects of sulfonated (1a–c) and de-sulfonated (4a–c) glucoglycerolipids were assayed using a short-term in vitro assay for EBV-EA activation in Raji cells induced by the tumor promoter TPA, as described [44,45]. Conversely, the presence of only single chains is distinctive of synthetic sulfoquinovosyl-monoacylglycerols (SQMGs). Recently, both SQDGs and SQMGs have been known to be considered as promising compounds for cancer therapy and prevention, due to their noteworthy biological activities. With regard to cancer prevention, 2-O-(β-d-glycopyranosyl)-sn-glycerol-related lipids (synthetic analogues of natural glycoglycerolipids) have recently shown high in vitro and in vivo antitumor-promoting activities and, in particular, the in vitro activities of fatty acid monoesters of 2-O-(β-d-glucopyranosyl)-sn-glycerol [namely 1-O-acyl-2-O-(β-d-glucopyranosyl)-sn-glycerols] proved to be strictly related to the length of the acyl chains. In view of these results, we set out to synthesize a new class of SQMGs derived from 2-O-β-d-glucopyranosyl-sn-glycerol, in which acyl chains of different lengths would be linked to one of the two free hydroxyl groups in the glycerol part, and to test their in vitro antitumor-promoting activities. Here, we have reported a rapid chemoenzymatic synthesis of the sulfoquinovosides 1a–c. 1-O-Octadecanoyl, -dodecanoyl, and -hexanoyl-2-O-(β-d-sulfoquinovopyranosyl)-sn-glycerols – in which the key steps were the selective 6′-β-O-tosylation of the glucose moiety of a suitable starting compound, selective lipase-catalyzed 1-O-monoacylation of the glycerol moiety, and a final oxidation reaction without any protection of the sugar unit. The in vitro antitumor activities of the obtained compounds 1a–c are also reported. A preliminary bioassay was carried out on the obtained compounds, showing their antitumor-promoting activities, and some new structure–activity relationship conclusions on the influence of the sulfonate group could also be drawn. In particular, a highly negative effect of this group was observed for the short-chain compound 1c in relation to the corresponding non-sulfonated 6c. In contrast, when a long acyl chain is present on the glycerol moiety, the simultaneous presence of a sulfonate moiety does not significantly induce variation in activity, showing that the effects of the “negative” (sulfonate and long-chain) groups are not additive. This conclusion is strengthened by the recent observation that 1,3-di-O-octadecanoyl-2-O-(β-d-sulfoquinovopyranosyl)-sn-glycerol, which contains three different “negative” factors (i.e., sulfonate, long-chain, and double acylation of glycerol) has an activity similar to those of compounds 1a and 6a [46]. Work to further characterize the biological potential of these new synthetically available sulfoquinovosyl-monoacylglycerols is in progress.
5 DNA polymerase inhibitor
Cancer therapy usually involves exposing the body to agents that kill cancer cells more efficiently than normal tissue cells. Such therapies must therefore exploit specific molecular and cellular features of the cancer they are aiming to eliminate. Most cancer cells proliferate more rapidly than their normal counterparts, so most cancer drugs target the cell cycle. Cell division can be targeted directly by inhibitors of the mitotic spindle, thus, preventing equal division of DNA to the two daughter cells. The growth signals that result in entry into the cell cycle can be targeted by hormonal manipulation, therapeutic antibodies and drugs that inhibit growth-signaling pathways. However, the most common means of targeting the cell cycle is to exploit the effect of DNA polymerase inhibition. DNA polymerase inhibition causes cell cycle arrest and cell death either directly or following DNA replication during the S phase of the cell cycle. Many cancer drugs employed in the clinic have been used for several decades and are highly efficient in killing proliferating tumor cells. DNA synthesis is sometimes targeted by inhibitors of DNA replication, which directly inhibit DNA polymerases.
6 Evidence for SQAGs
Multicellular eukaryotes are known to contain at least 14 types of DNA polymerases [47]. Sulfo-glycolipids, including the stereoisomers are found to be the potent inhibitors of DNA polymerase a and h in eukaryotes. In 1989, Gustafson et al. [48] reported that a mixture of various types of α-SQDG from cyanobacteria possessed antiviral activity in cultured cells. However, there is a great variety of long-chain fatty acids in natural α-SQDG and it is too difficult to isolate them from each other. As it is well known, cells exist in an ongoing balance among cell growth, cell proliferation and cell death. Cell growth is tightly regulated in tandem with the cell cycle by the relationship of numerous proteins. It is also interesting to note that the cell-permeable form α-SQMG leads to apoptotic cell death. However, since the α-configuration of SQDG with two stearic acids (α-SQDG) can hardly penetrate cells, it has no cytotoxic effect. Diversely permeable form, α-SQMG from α-SQDG by hydrolysis with a pancreatic lipase, inhibited DNA polymerase activity and was found to be a potent inhibitor of the growth of NUGC-3 cancer cells. α-SQMG arrested the cell cycle at the G1 phase and subsequently, induced severe apoptosis. The arrest was correlated with an increased expression of p53 and cyclin E proteins, indicating that α-SQMG induced cell death through a p53-dependent apoptotic pathway. The molecular targets of SQAGs, on the other hand, are unknown, although these agents are strong inhibitors of DNA polymerases a and h [49,50]. The molecular targets of SQAGs should exist on cell membranes or as extracellular proteins, such as growth factors or cytokines. Cell cycle regulation by α-SQMG as well as α-SQDG was also an inhibitor of pol a; it is an essential initiator of DNA replication. We, therefore, hypothesized that the inhibition by α-SQMG must occur by inducing the cell cycle arrest. To estimate the effect of α-SQMG on cell cycle regulation, the group of Prof. Yoshiyuki Mizushina studied the cell cycle of human stomach cancer cell line NUGC-3 cells by flow cytometry with DAPI staining. When the cells were treated with 150 AM α-SQMG, the distribution in the cell cycle was time-dependently changed. In the presence of α-SQMG, the percentage of the cells in the S phase decreased (44.1% to 26.7%) and induced an increase in the percentage of the cells in the G1 phase (38.5% to 61.9%) (Fig. 4; Part I (A), (a and d)). These results suggested that α-SQMG induces cell cycle arrest in the G1 phase. Explicative DNA polymerases are essential enzymes in DNA replication, and the cells treated with the inhibitor were considered to induce the cell cycle block at the late G1 phase. The cell arrest by α-SQMG must also reflect the α-SQMG-dependent inhibitory effect on replicative DNA polymerases, such as pol a. To investigate why α-SQMG blocks with the cell cycle progression at the G1 phase, they have tested the cyclin E content. Fig. 4 (Part I) shows that with the G1 blockage with 150 μM α-SQMG, the cyclin E expression in NUGC-3 cells increased time-dependently.
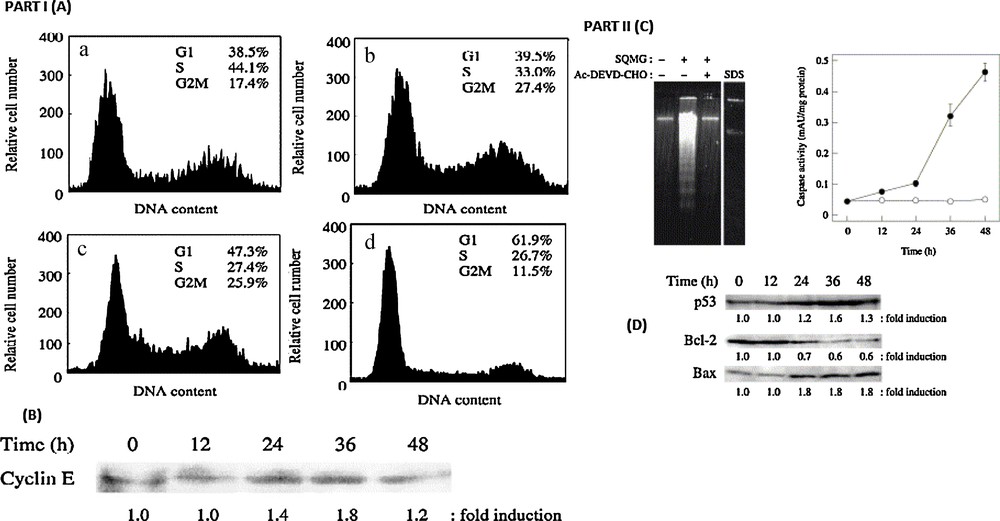
Part (I) Effect of cell cycle distribution by α-SQMG-C18. (A) Flow cytometric analysis. NUGC-3 cells were incubated with 150 μM α-SQMG-C18 for 0 (a), 12 (b), 24 (c) and 48 h (d). The cell cycle distribution was calculated as the percentage of cells that contained G1, S, G2/M DNA analyzed by flow cytometry with DAPI staining. (B) Cyclin E expression in cells incubated with 150 μM α-SQMG-C18 for the indicated times. Protein level was analyzed by western blotting with specific antibodies. Densitometric analysis for the cyclin E protein was performed and fold induction was calculated. Part (II) Induction of apoptosis by α-SQMG-C18. (A). Agarose gel electrophoresis of DNA fragments. NUGC-3 cells were pre-incubated with or without 100 μM Ac-DEVD-CHO for 3 h followed by incubation with or without 150 μM α-SQMG-C18 for 48 h (Lanes 1–3). Lane 4, NUGC-cells were incubated with 0.01% (346 μM) SDS for 48 h. DNA fragments were analyzed by 1.5% agarose gel electrophoresis. (B). Caspase-3 activity in the cells incubated with 150 μM α-SQMG-C18. NUGC-3 cells were incubated with (●) or without (○) 150 μM α-SQMG-C18 for 0, 12, 24, 36 and 48 h. Data are shown as mean ± SE for four independent experiments. (C). Apoptosis-related protein expression in cells incubated with 150 μM α-SQMG-C18. NUGC-3 cells were incubated with 150 μM α-SQMG-C18 for the indicated times. Protein levels of p53, bcl-2 and bax were analyzed by western blotting with specific antibodies. Densitometric analysis for the proteins was performed and fold induction was calculated.
7 Induction of apoptosis and expression of apoptosis-related protein by α-SQMG
To examine whether the growth inhibitory effect of α-SQMG was induced by apoptosis or not, the cells were incubated with α-SQMG for 48 h. Apoptosis was detected by the formation of oligonucleosomal fragments, which is a late event of apoptosis. DNA fragmentation was observed when the cells were treated with α-SQMG (lane 2, Fig. 4 Part II C). In parallel to the DNA fragmentation, it is important to investigate the effect of α-SQMG on the caspase-3 activity, which is a key in the execution of apoptosis mediated by various antitumor agents [51].
Caspase-3 activity was measured in cells treated with 150 μM α-SQMG for various periods. The enzyme activity of caspase-3 increased in a time-dependent manner and especially, after 48 h, the caspase-3 activity in the α-SQMG-treated cells increased by a factor of about eight (Fig. 4, Part D). In addition, the DNA fragmentation induced by α-SQMG was completely inhibited in the cells pre-incubated with the caspase-3 inhibitor, Ac-DEVD-CHO (lane 3, Fig. 4 Part II C).
A specific pol a inhibitor, aphidicolin, arrested cells in the G1 phase [52]. Since α-SQMG could also inhibit the pol a activity, this previous evidence supports our results that cells were arrested in the G1 phase (Fig. 4 Part I A). The transition from G1 to S phase in all eukaryotic cells is tightly linked to the kinase activity of cyclin E/cdk 2 complexes. Cyclin E/cdk 2 activity was shown to be maximal in the G1/S phase [53,54]. The bax system was also tested in the presence of α-SQMG (Fig. 4 Part II). Bax is a member of the bcl-2 gene family, acting as a promoter of cell death and present predominantly in the cytosol. However, partial translocation to the mitochondria could occur on the activation of the caspase cascade, resulting in apoptosis induction [55,56]. In this study, α-SQMG-treated cells showed high levels of caspase-3 activity (Fig. 4 Part II D) and α-SQMG induced apoptosis was inhibited when treated with a caspase inhibitor (Fig. 4 Part II C).
These results suggested that apoptosis induced by α-SQMG is mediated through caspase-3 activation. Pol a is the only enzyme capable of initiation of DNA synthesis de novo by first synthesizing an RNA primer and then extending the primer by polymerization to produce a short DNA extension. Then, chromosomal DNA, after being initiated and having entered into the S phase, is elongated by pol y and pol q, replaced by pol a. These replicative DNA polymerases are highly conserved in eukaryotes, and the inhibition of replicative DNA polymerases could induce the cell cycle arrest at the G1 and S phases and, subsequently, apoptosis. In conclusion, since pol a is essential to the initiation of DNA synthesis, the inhibition of the replicative DNA polymerase by the cell-permeable form α-SQMG might block entry into the S phase from G1, inducing apoptosis. α-SQMG belongs to a new category of apoptosis inducers and could be an anticancer chemotherapeutic agent.
8 Antiangiogenic agents
Angiogenesis, the development of new blood vessels from pre-existing vasculature takes place primarily during embryonic development, but also occurs in adults in a highly regulated manner during physiological processes, such as wound healing, ovulation and menstruation [57]. The prominent role of angiogenesis in cancer biology was first described by Folkman [58]. According to The Angiogenesis Foundation, at least 184 million patients in western nations alone could benefit from some form of antiangiogenic therapy, So far, an estimated US $4 billion has been invested in the research and development of angiogenesis-based medicines, which makes the sector one of the areas of medical research in which one has ever most heavily invested. More than 30 antiangiogenic molecules are now in clinical development, mainly for the treatment of cancer, with many others undergoing pre-clinical evaluation, as we know antiangiogenic molecules according to their mode of action inhibitors angiogenic growth factors, such as vascular endothelial growth factor (VEGF), basic fibroblast growth factor (bFGF) and platelet-derived growth factor (PDGF); protease inhibitors that prevent the breakdown of the surrounding matrix which is needed for blood vessel growth; endogenous inhibitors of angiogenesis, such as endostatin; inhibitors of cellular adhesion molecules; and some molecules with undefined mechanisms. However, it was reported that the receptor tyrosine kinase Tie-2 could play a critical role in tumor-induced angiogenesis. It was also demonstrated that the development of cancer drugs that stifle tumor growth by blocking the formation of the blood vessels they need seems to have turned the corner.
9 Evidence for SQAGs
Among all SQAGs, we have evidenced that SQMGs are effective in suppressing the growth of solid tumors due to hemorrhagic necrosis in vivo. Prof Yoko Mori investigated the antiangiogenic effect of SQMG. Accordingly, in vivo assessment of antitumor assays showed that some tumor cell lines, but not others, were sensitive to SQMG. Significant downregulation of Tie-2 gene expression was observed in all SQMG-sensitive tumors as compared with controls, but not in SQMG-resistant tumors. These data suggested that the antitumor effects of SQMG could be attributed to antiangiogenic effects. α-SQMG synergistically inhibited the growth of human tumors transplanted into nude mice, accompanied by a significant reduction in the vascularity of the tumors [59]. It has been demonstrated that the antitumor effects of SQMG could be attributed to the inhibition of tumor antiangiogenesis, which seems to be involved in the downregulation of the Tie-2 gene. Experiments done by Prof Yoko Mori et. al. has been indicated that SQMG treatment results in hemorrhagic necrosis in tumors. To investigate the mechanism of the antitumor effects of SQMG, we first carried out an immunohistochemical analysis to determine the angiogenesis profiles in tumors. Tumors were excised from mice on the next day after the last injection and cryosections of these acetone-fixed tumors were stained with antimouse CD31 monoclonal antibody as an endothelial cell marker. Representative photos showing immunohistochemical staining of MDA-MB-231 tumors treated with or without SQMG are presented in Fig. 5A, in which CD31-positive ring-form blood vessels are clearly observed for both control (Fig. 5 A (a, b)) and SQMG treatment (Fig. 3c,d). Therefore, the CD31-positive ring-form blood vessels of all samples in 500-mm2 section areas were counted under a fluorescence microscope. For all four SQMG-sensitive tumors treated with 20 mg/kg SQMG, the numbers of blood vessels were significantly decreased (P < 0.01) with SQMG treatments, as compared with controls. We suggested that the antitumor effect of SQMG could be attributed to the inhibition of tumor angiogenesis.

(A). Antiangiogenesis immunohistochemical analysis. Cryosections of MDA-MB-231 treated (a, b) without and (c, d) with SQMG. Arrows indicate CD31-positive blood vessels. Insets in (a) and (c) are magnified and shown in (b) and (d), respectively. Scale bar = 100 μm. Color available online. (B). Influence of SQMG on cell proliferation and apoptosis. (a): human umbilical vein endothelial cells (HUVEC) and (c): NIH3T3 were cultured in the presence or absence of SQMG at the indicated concentrations. Cell proliferation was examined by MTT assay. Results represent means ± SE of triplicate wells on one of three independent experiments; (b): HUVEC were cultured in the presence or absence of SQMG at the concentrations indicated for 48 h, harvested, and double stained with annexin-V–fluorescein and propidium iodide (PI). The percentage of apoptotic (annexin V and PI double positive) cells was determined by flow cytometric analysis. Results represent means ± SE of three independent experiments, 70% compared with the control (Fig. 5C). Thus, these data suggested that SQMG could influence capillary formation. (C). Effect of SQMG on angiogenesis in vitro. (a–c): human umbilical vein endothelial cells (HUVEC) grown on a fibroblast sheet on Matrigel were cultured (a) without and (b, c) with SQMG at the indicated concentrations. After cultivation, the cells were fixed and stained with an antihuman CD31 antibody for 1 h. CD31 molecules were detected using the alkaline phosphate method; (d): the tube formation of HUVEC was quantitated using Image ++ software. Results represent means ± SE of five independent areas on one of three independent experiments. *P < 0.01.
To investigate whether SQMG directly affected the growth profile of endothelial cells, proliferation and cytotoxicity have been assessed by using the human endothelial cell line HUVEC. SQMG was added to HUVEC in concentrations from 0 to 100 μM and cell proliferation and apoptosis were analyzed using the MTT assay and annexin-V labeling assay, respectively. As shown in Fig. 5A (a), there was no obvious inhibitory effect on the proliferation of HUVEC up to the concentration of 25 μM. When 50 or 100 μM SQMG was added to cells, the cell proliferation was inhibited until 71.5 ± 5.6 or 55.3 ± 4.5%, respectively. Meanwhile, when SQMG at concentrations from 0 to 50 μM was added to cells, 8.5–13.3% of the cells were observed to be apoptotic. However, when 100 μM SQMG were added to the cells, apoptotic effects were increased to 33.8 ± 11.6%, suggesting that there was minimal weak influence on the apoptosis of HUVEC up to the concentration of 50 μM SQMG (Fig. 5B (b)). Researchers further considered that it would be important to evaluate the antiangiogenic activity of SQMG using an angiogenesis model in vitro that is considered as closely representing in vivo situations. It is well known that HUVEC co-cultured with fibroblast cells on Matrigel form capillary networks with tube-like structures and adopt characteristics of newly formed blood vessels [59]. Before investigating the influence of SQMG on the formation of these structures, we studied the fibroblast cell proliferation and cytotoxicity using mouse NIH3T3 instead of human normal fibroblasts by MTT assay. Consequently, there was no obvious cytotoxic potential up to the concentration of 100 μM SQMG (Fig. 5B (c)).
It is important to appreciate that no antiangiogenic product has yet gained market approval, and that there have been several prominent failures, in particular with inhibitors of matrix metalloproteinases, such as BAY 12-9566 (Bayer), Prinomastat (Pfizer) and Marimastat (British Biotech). In part, this might be because antiangiogenic ccompounds typically stop the growth of tumors, but do not necessarily shrink them, which makes the design of clinical trials challenging. These data suggested that the antitumor effects of SQMG could be attributed to antiangiogenic effects.
10 Antiangiogenic radiosensitizers
Although we previously demonstrated that SQAGs are promising agents for angiogenesis for the treatment of cancer, adverse effects and limitations associated with antitumor therapies have recently become apparent. Almost all angiogenesis inhibitors could still cause side effects at effective doses and only additive effects are seen in current combination therapy. To overcome these problems, combining such agents with chemotherapy or radiotherapy is now strongly recommended in clinical practice. Provided such combination treatment, from the onset of therapy, different strategies in developing antiangiogenic agents should be used to enhance any combinatory effects and reduce adverse effects. The first paper reporting that the combination of radiation and antiangiogenic agents effective in animal studies was the one by Weichselbaum's group [60]. The vascular normalization model was the first to implicate synergistic interaction mechanisms between the effects of radiation and the antiangiogenic agents of reoxygenation. This encouraged us to seek agents with efficient synergistic actions in combination and led us to develop the novel concept of “antiangiogenic radiosensitizers”. Radiosensitizers by themselves have no antitumor effects, but synergistically enhance them when combined with radiation. Although radiosensitizers generally target tumor cells, this concept can also be applied to vascular endothelial cells. These agents are harmless in the absence of irradiation, which minimizes systemic side effects. Radiotherapeutic techniques make it possible to focus radiation on tumor tissues. Therefore, such agents synergistically inhibit angiogenesis.
11 Evidence for SQAGs
Sahara et al. [61] reported that SQMGs inhibit the growth of the human lung adenocarcinoma cell line A549 after subcutaneous transplantation into nude mice. Using a human umbilical vein endothelial cell–fibroblast co-culture assay, they examined whether the agents possessed the properties of antiangiogenic radiosensitizers. Interestingly, all assays concerning capillary-like formation on a Matrigel layer, invasion through a Matrigel layer and tube-like formation by human umbilical vein endothelial cell–fibroblast co-culture assay (Fig. 6A) revealed some synergistic inhibition after combining SQMGs with radiation at doses presenting no effects when either of them was used alone [62].
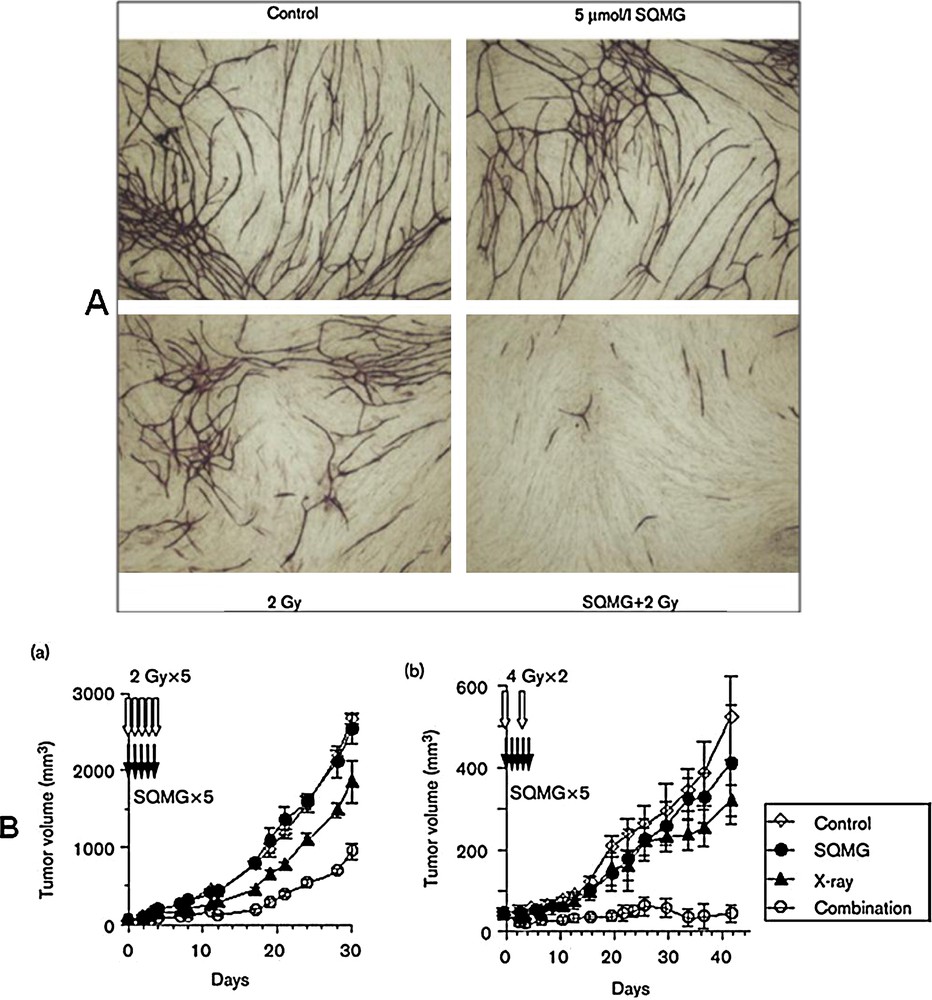
(A). Tube formation of human umbilical vein endothelial cells (HUVECs) in HUVEC–fibroblast cocultures after combined treatment. (B). Tumor growth delay in vivo after combined treatment. (a) SAS (a human tongue squamous cell carcinoma cell line) and (b) A549 (a human lung adenocarcinoma cell line).
When several human tumors transplanted into nude mice were treated with a combination of radiation and SQMGs, a synergistic delay of tumor growth was observed at doses presenting no antitumor effects of SQMG used alone (Fig. 6B).
Furthermore, the vascular density was significantly lower in tumors that received the combined treatment than in those treated with radiation alone. These results demonstrate that agents with the characteristics of antiangiogenic radiosensitizers do exist. In the course of examining the radiosensitivity of vascular endothelial cells, Garcia-Barros et al. reported that the apoptotic activity in vascular endothelial cells after irradiation was the primary determinant of tumor response. We recently found that radiation alone (B8 Gy) induces a high-frequency (> 90%) senescence-like phenotype in vascular endothelial cells and that such growth arrest could result in the strong inhibition of angiogenesis (Igarashi et al., 2006, in preparation). The results explain the fact that the synergistic effect of SQMGs on the surviving fraction becomes lower as the radiation dose increases. This indicates, therefore, that SQMGs enhance the radiation-induced senescence-like phenotype that leads to the dysfunction of vascular endothelial cells, suggesting that surviving endothelial cells that express a senescence-like phenotype could also contribute to the inhibition of angiogenesis in addition to inducing apoptosis. Furthermore, we can form SQAG vesicles by taking advantage of their lipidic properties [63]. By utilizing these properties of SQAGs, many new therapeutic possibilities may become available, including drug delivery systems and imaging techniques in addition to those of radiosensitizers. Availability of SQAGs may open new possibilities in the development of multi-modal combination therapies against cancer. Additionally, the combination of α-SQMG and radiation caused endothelial cells to adopt a senescence phenotype, but apoptosis was not significantly induced. Finally, the radio response of several different tumors was dramatically enhanced by the co-administration of α-SQMG at doses well below those causing any systemic toxicity. The enhanced tumor response was likely due to decrease angiogenesis because tumors from mice undergoing combined therapy exhibited significantly decreased vascularity. Recently, it is found that α-SQMG binds the extracellular domains of Tie-1 and Tie-2, which are receptors specifically expressed on vascular endothelial cells [64] and play important roles in angiogenesis together with VEGFRs, using phage display screening and surface plasmon resonance analysis [65]. The relationship between the antiangiogenic activity of α-SQMG and binding with such molecules is currently under investigation. The combination of α-SQMG with radiation inhibits angiogenesis at two distinct stages: early inhibition of capillary formation and endothelial cell invasion, and the specific induction of a senescence-like phenotype in endothelial cells at later times, with subsequent growth arrest. The precise molecular mechanism and biological significance of this phenotype in vivo remain unclear, but p53 and p21 clearly play crucial roles in this process. It was recently reported that the accumulation of double-strand breaks ultimately determines the induction of senescence. [66]. Thus, it is also possible that low doses of α-SQMG might affect the repair of radiation-induced double-strand breaks, which are specifically regulated in endothelial cells. Kolesnick et al. reported that tumor vascular endothelial cell apoptosis determines the clonogenic dysfunction of tumor cells and, consequently, tumor radio response [63]. In general, optimal radiotherapy would directly kill the maximum number of cancer cells, whereas angiogenesis inhibitors would kill cells indirectly by depriving tumors of nutrients and oxygen. However, reduced oxygen levels could render cells radioresistant, which makes tumor cells resistant in a fractionation regimen of irradiation. It was recently proposed that some degree of vascular normalization occurs in tumors with time, and the decreased hypoxia promotes radiosensitization [67]. When further optimized, treatment protocols using α-SQMG or similar compounds in conjunction with radiotherapy may hold great promise for the treatment of solid tumors.
Some researchers reported that radiation actually activates angiogenesis via the production of proangiogenic and antiapoptotic factors, such as VEGF, from irradiated tumor cells [68,69]. Thus, it remains unclear as to whether radiation inhibits or activates angiogenesis. Indeed, several papers have reported that the combination of antiangiogenic agents and radiation reduced the radiation effect [70,71]. Combined treatments with α-SQMG and radiation seem to promote the adoption of a senescence-like phenotype by vascular endothelial cells. Finally, the agent remarkably enhances the radioresponse of human tumors transplanted into nude mice, accompanied by a significant reduction in the vascularity of the tumors. Collectively, α-SQMG may be a novel potent radiosensitizer targeting angiogenesis.
12 Immunosuppressive quality of SQAGs
Harnessing the immune system in the battle against cancer has been the focus of tremendous research efforts during the past two decades. In general, two different types of vaccination strategies are being pursued: vaccines that utilize whole tumor cells and vaccines that target defined antigens [72]. Although the main components of tumor cell-based vaccines are irrelevant proteins, such vaccines have the advantage that all relevant tumor antigens are included. However, the complexity of the antigenic composition of tumor cells makes the immunological monitoring of these therapeutic interventions troublesome. Immunosuppressive drugs are indispensable for the success of allogeneic transplantation.
13 Evidence for SQAGs
It has been reported that sulfonolipids possess several functions in the cell growth control mechanism [73] and there was also a possibility that sulfo-glycolipids could be used as immunosuppressive drugs. We have investigated whether these synthetic sulfonolipids could work as immunosuppressive reagents in allogeneic mixed lymphocyte reaction (MLR). Interestingly, one of these sulfonolipids, β-SQDG, demonstrated a strong inhibitory effect on the MLR in a dose-dependent manner.
T-cell activation by allogeneic MLR has long been known as a basic phenomenon in cellular immunology. It was reported that CD4+ T-cells play a more essential role than CD8+ T-cells in primary allogeneic MLR [74]. However, relatively little is known about which subset of T-cells mainly responds to allogeneic stimulator in MLR. CD62L (L-selectin) is one of the adhesion molecules expressed on the T-cell surface, and it also acts as a homing receptor at the endothelial venule in peripheral lymph nodes. This molecule is expressed on naïve and central memory T-cells, suggesting the critical role of immunological surveillance [75]. In this study, flowcytomeric analysis indicated that approximately 70% of T-cells in PBMCs co-expressed CD62L and CD45 RA molecules, and that CD62L+ T-cell subset was composed of four times more CD4+ T-cells than CD8+ T-cells. This observation implied that the majority of CD62L+ -cell subset in PBMCs would be naïve CD4+ T-cells [76]. CD62L expression on naïve CD4 T-cells is required for their efficient re-circulation and compartmentalization between blood and lymph nodes [77]. This data were compatible with the results that CD62L+ T-cell subset was composed mostly of naïve CD4+ T-cell, and that this subset had more efficient proliferative activity than CD62L+ T-cells in allogeneic MLR. It is reported previously that a synthetic β-SQDG has immunosuppressive effects in vitro and in vivo, and that these effects were thought to be caused by the contact inhibition between T-cells and APCs without affecting the cell surface molecule expression, such as CD4, CD28, LFA-1, ICAM-1, CTLA-4, MHC class I, and MHC class II molecules [78]. This data showed that β-SQAG suppressed T-cell responses in MLR as β-SQDG, and subsequently, the effects were shown to be directed against CD62L+ T-cell subset. We confirmed by two different kinds of binding assay that the L-selectin molecule interacted directly with β-SQAG. The ligands for L-selection have been identified, and those modified for binding have also been determined [79]. It was reported that L-selectin binds to glycoproteins that contain oligosaccharides, sLeX in structure and also some of the sulfated glycolipids, for example sulfatide [80]. Moreover, the sulfonoquinovosyl dipalmityl glyceride that is extracted from alga was reported to bind to P-selectin [81]. Since β-SQAG has a chemical structure very similar to that of this glyceride, our current data show that β-SQAG bound to L-selectin would be compatible with these reports. From the date of the blocking assay, the CD62L molecule itself could not influence T-cell recognition of APCs.
Although sulfatide also bound to L-selectin, the suppressive effects could not be shown. In the case of the binding assay, β-SQAG would be in a simple substance state in 50% methanol solution [82] and fixed on plate, and this state of β-SQAG could bind to L-selectin (Fig. 7). However, the suppressive effects of β-SQAG disappeared when β-SQAG became in a simple substance state by detergents (Fig. 7). Therefore, it was considered that the CD62 L molecule itself could not relate T-cell allow recognition of APCs. It was previously reported that β-SQAG formed a liposome structure in the PBS. In the current study, we exploited this character of β-SQAG and made calcein–SQAG. This data showed that liposome of composed of β-SQAG bound to specific CD62L molecule on the cell surface, but not in the cell cytoplasm.
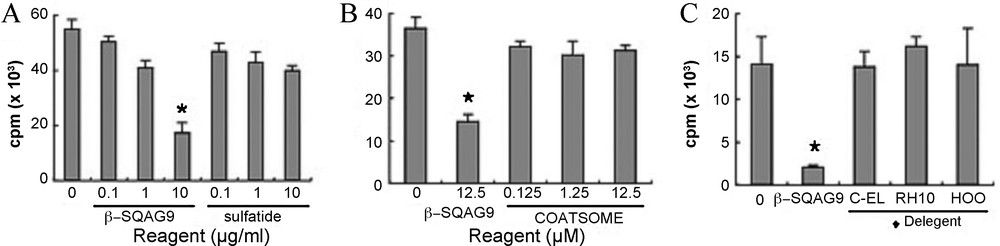
Immunosuppressive effect of β-SQAG depends on liposome formation. β-SQAG was pretreated with 1% of detergents, Cremophor EL (C-EL), Cremophor RH40 (RH49), and NIKKOL HCO-40 (HCO) or not and added to MLR cultivation wells. Each bar represents mean + SEM of triplicate determinations.
In fact, SQDG with two stearic acids hardly passed through the cell membrane [83]. When the liposome structure of β-SQAG is destroyed by detergents, T-cell responses to APCs could not be inhibited by it. So, the structure of the liposome is important for the suppressive effect. However, the liposomes composed of some reagents, such as cholesterol, phosphatidylcholine, and phosphatidylglycerol, were not essential for the inhibition of T-cell responses, but liposomes composed of β-SQAG were critical for the inhibitory effects. Took together, these data lead us to propose that β-SQAG liposomes bind to L-selectin on the cell surface of CD62L+ T-cells and inhibit contact between T-cells and APCs. Consequently, sufficient interaction between T-cells and APCs is inhibited and subsequent immunological reactions are suppressed. Although it is necessary to ascertain whether immunosuppression of β-SQAG occur by contact inhibition in vivo, our current study implied that β-SQAG has a great deal of potential as an immunosuppressive reagent with a novel interaction to L-selectin (Fig. 8).

β-SQAG9 has binding activity to L-selectin. (A–F). Binding activity of β-SQAG9 and the related glycolipids to adhesion molecules was measured by ELISA. Glycolipids were plated out in a 96-well plate, and then these molecules-Fc chimera-peroxidase-conjugated antibodies were added to the wells. Fc chimera-proteins that are bound to the wells were detected by the absorbance at 405 nm. Closed circle, closed triangle, and open square indicate β-SQAG9, β-GDG, and sulfatide, respectively. Means ± SEM are shown in triplicate determinations of three independent experiments.
A problem occurred for in vivo uses: β-SQDG was hard to dissolve in water. If not perfectly dissolved, iv injection of β-SQDG would be dangerous since peripheral blood vessels might become clogged. To solve this problem, we have paid attention to molecular assemblies, especially vesicle formation. The vesicles are usually known to be formed in the system of amphiphiles, e.g., double-chained amphiphiles. β-SQDG has two hydrophobic tails, consisting of stearyl esters that are connected to the hydrophilic sulfo-glucose moiety, so that it might be expected to form vesicles. The vesicle formation of β-SQDG is advantageous i.e. administration in its chemical–structural character. With preparation in water, β-SQDG was hard to form vesicles, because its hydrophilicity was strong. We examined the suitable parameter of the vesicle forming condition. It was possible to take a balance between the hydrophilicity and the hydrophobicity of the β-SQDG molecule to be optimized to form vesicles in 150 μM PBS. In addition, the strong immunosuppressive activity of β-SQDG vesicles is demonstrated. This is the first report of the preparation method of β-SQDG vesicles, which should facilitate in vitro and in vivo applications.
SQAGs can not only arrest cells at the S phase but also at the M phase [84]; thus, the two different cell cycle phases may be impacted simultaneously. The immunosuppressive effects of synthetic SQAGs, including stereoisomers, were interesting for the development of a promising clinical drug [85]. Especially, β-SQDG-C18 was thought to be a valuable candidate because of the preliminary observations of its high inhibitory activities in spite of low toxicity.
14 HIV-reverse transcriptase inhibitor
Since the discovery of the human immunodeficiency virus (HIV) in 1983, dramatic progress has been made in the development of novel antiviral drugs. The HIV epidemic fuelled the development of new antiviral drug classes, which are now combined to provide highly active antiretroviral therapies. Considering SQAGs as potent HIV-reverse transcriptase inhibitors, there are much selected study is available as evidence until date [85], so it is too early to reach the authentic conclusion that yes! SQAG's are able to inhibit HIV-reverse transcriptase. The groups of researchers are enthusiastic to know the reality and are working to reach the authentic conclusion. For sure, future will be clearer.
15 Conclusions
In reality, the road to a marketed drug is often far from the frequently depicted linear path. Extracting practical insights and lessons from such experiences by discussing drug discovery. In the golden age of drugs discovery (1940–1960s), synthetic drugs were generally discovered empirically, without pre-selection of targets. Many of those compounds were developed for clinical use in systemic monotherapy and were the progenitors of further generations of drugs, addressing the single target problem. If single targets are not optimum, what are the options? Multi-targeted drugs? The available data discussed above and accumulated evidences suggest that sulfoquinovosylacylglycerols (SQAGs) exert extensive biological activities, such as DNA polymerase inhibition [47–51], antiangiogenesis activity [59], radiosensitizer [62,63–71], immunosuppressive quality [73–84,86] and HIV-reverse transcriptase inhibition [85]. Due to the high interest related to bioactive natural sulfo-glycolipids, work is in progress to further identify the biological potential of these new synthetically available sulfoquinovosylacylglycerol analogues towards the development of multi-targeted drugs.
Acknowledgements
Financial support provided by the University of Milan is greatly acknowledged.