Wet chemistry methods, known as “chimie douce”, bring together organic and inorganic chemists. They were pioneered by French scientists, most prominently represented by Rouxel and Tournoux [1], Corriu [2], and Livage [3]. These techniques offer new opportunities for the chemist's imagination and lead to the synthesis of novel hybrid or bio-inspired materials. Sol-gel processes of inorganic materials have been extensively studied during the past 25 years. Based on inorganic polymerization reactions, they allow the fabrication of materials at temperatures much lower than usual glass and ceramic processes. Moreover, chemical syntheses and shaping can be performed in a single step directly from the solution leading to the powderless processing of films or fibres. Hybrid organic–inorganic materials have been synthesized via these chimie douce reactions, and even biomolecules can be encapsulated within sol-gel matrices. A whole range of novel hybrid compounds is emerging, opening a land of opportunity for materials science and technology [4]. The concepts of “chimie douce” have previously been mainly applied to transitions metal oxides [5,6], but have been already extended, for instance to metal phosphates [7] or organic–inorganic hybrid materials [8]. It is the purpose of the present article to discuss the feasibility of extension of this approach to organic high end materials, here the rich world of carbon connectivities, nanostructured materials and their hybrids. Can we generate a “chimie douce” of carbon structures, which are otherwise only made by reactions at very high temperatures, arc discharge, or by decomposition from the plasma or gas phase?
To justify the notation “chimie douce”, some prerequisites have to be fulfilled:
- • the reaction should proceed in a solvent, favourably water or water–alcohol mixtures;
- • the reaction temperature and the applied conditions should be much less extreme than otherwise applied;
- • chemical condensation, shaping to film, coatings, monoliths or hybrids should be performed in one single step.
This is indeed a challenge for carbon-like materials, as the strength of the C−C− bond is much higher than the one of a corresponding M−O−M− bond and in addition much less reversible or apt to cross-exchange.
However, there are options left. Livage and Sanchez stated [8]:
“Sol-gel chemistry involves nucleophilic reactions. The chemical reactivity of metal alkoxides toward hydrolysis, condensation and complexation mainly depends on the electronegativity of the metal atom, its coordination number and the steric hindrance of alkoxide groups. Hydrolysis and condensation rates have to be enhanced by acid and base catalysis or nucleophilic activation. In classical sol-gel chemistry, the molecular design of alkoxide precursors and reactions opens the way to tailor-made materials”.
1 The three requirements of chimie douce: less extreme conditions
Transferred to the world of carbon, this means that we have to consider appropriate activated leaving groups, nucleophilic catalysis, and adjust the conditions to the more inert character of carbon. Such sustainable approaches for the synthesis of polymer–carbon materials have been lately exploited by several groups. Zhao et al. used phenol-formaldehyde condensation and the tool of surfactant templating to generate a variety of porous carbon scaffolds, otherwise really just known from the sol-gel chemistry of metal oxides [9–12]. Rather parallel, Wang used hard templates and opal structures for designing periodic porous carbons, again transferring principles from sol-gel chemistry to the nanostructure formation of carbons [13]. Here, we will mainly focus on a technique which makes carbons in a sol-gel style, based on the use of carbohydrates or low value biomass as monomers for the production of functional porous polymeric and carbonaceous materials [14–19]. To make the reaction sufficiently feasible and similar to inorganic sol-gel reactions, temperatures of 180 to 220 °C have to be applied, i.e. hydrothermal conditions. This is “douce” with respect to all other carbonization reactions, but of course still more extreme than the conditions usually applied in sol-gel synthesis. Obviously, the notation “douce” depends on the scientific community one is working in.
Hydrothermal carbonization, however, nevertheless represents a simple and environmentally friendly technique through which sugars or biomass is first converted into precondensed polymers and then, following further dehydration/polymerization/condensation processes, into carbon rich, coal-like derivatives [20,21]. It represents also an option to sequester carbon and the stored energy from plant material [15,22]. Although this process is already known from 1913 when Bergius and Specht described the hydrothermal transformation of cellulose into coal-like materials [23], the process had been only recently rediscovered and exploited by several groups [14,18,19]. A contemporary review on hydrothermal carbonization is found in [24].
Our group performed together with the group of FBabonneau some investigations regarding the mechanism of transformation of the carbohydrates into carbonaceous materials [20,21], employing characterization techniques such as 13C solid state NMR and GS-MS. It was found out that the process of hydrothermal carbonization takes place via 5-hydroxymethyl-furfural-1-aldehyde (HMF) as a monomer, while C-C-linkage towards the final carbonaceous materials occurs via this intermediate involving cycloaddition, polymerization, and aldol condensation reactions. The latter is presumably even reversible under the applied conditions. Besides solid state 13C-NMR, this structure was also supported by XPS, FT-IR, XRD and Raman spectroscopy [25].
2 The three requirements of chimie douce: colloid formation in water
The most classical reaction of sol-gel chemistry, the formation of monodisperse nanoparticles (for Silica the Stöber-process [26]) can indeed be repeated that way, and carbonaceous nanoparticles are obtained (Fig. 1).
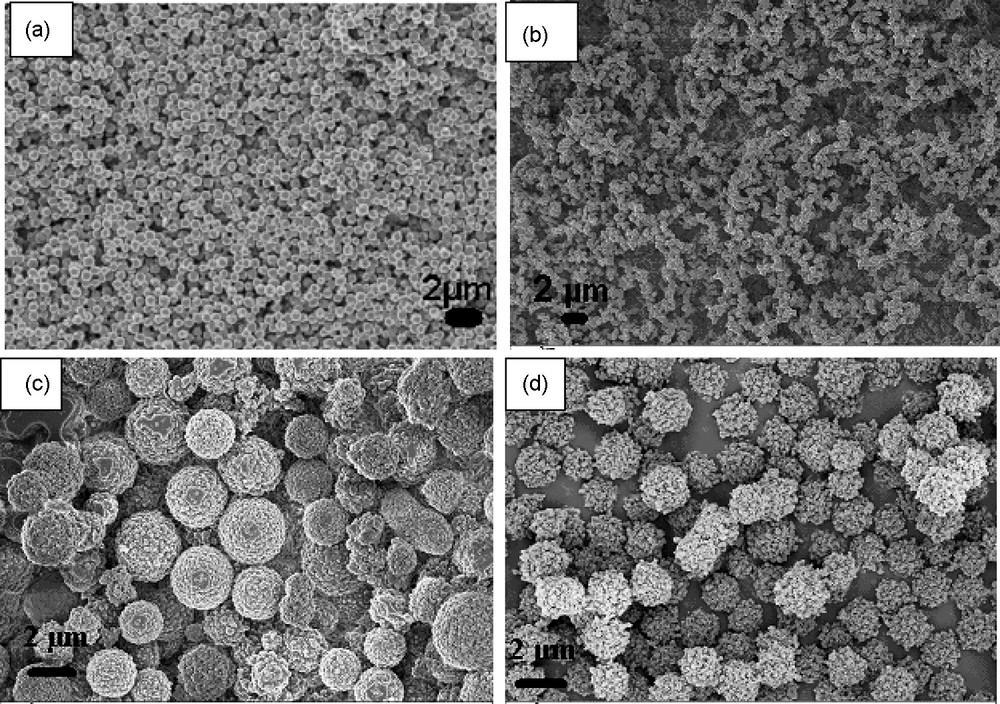
a: nanospheres generated upon the hydrothermal carbonization of glucose at 180 °C after 4 hours; b: nano-sized spheres obtained by hydrothermal carbonization of glucose in the presence of acrylonitrile; c: raspberry-like microspheres obtained upon hydrothermal carbonization of glucose in the presence of 2 wt% acrylic acid; d: raspberry-shaped microspheres obtained upon hydrothermal carbonization of glucose in the presence of 10 wt% acrylic acid.
As for silica, also copolymerization reactions can be performed, again adjusting the relative reactivity to each other. Here, the raw activation products of carbohydrates are combined with synthetic functional organic monomers1 [30]. Such spherical latexes consist mainly of renewable raw materials, have a low cost base and expand sol-gel chemistry to carbonaceous materials. Thus, for example, hydrothermal carbonization of glucose in the presence of acrylic acid [30] resulted in a new type of material with a raspberry-like structure and an increased numbered of carboxylic groups (Fig. 1c and d). The addition of acrylic acid induces a change in the particle morphology, that is, the surface of the particles is no longer smooth, and the architecture indicates that they are formed out of small aggregated particles (∼250 nm). The increased functionality (eg. carboxylic groups) was demonstrated using 13C solid-state Magic Angle Spinning (MAS) NMR, FT-IR and zeta potential. Due to the high number of carboxylic groups, these types of materials proved to be feasible adsorbents for the removal of toxic heavy metals from aqueous solutions, with a very strong binding affinity for Cd 2+ (89 mg/g adsorbent) or Pb2+ (352 mg/g adsorbent).
In a similar fashion, other organic functional monomers can be successfully incorporated within the carbohydrate based carbonaceous matrix (e.g. vinyl imidazole, acrylonitrile, acrylamide) resulting in porous materials with different functional surfaces. Acrylonotrile gives remarkably small and homogeneous particles (Fig. 1b). The material containing vinylimidazole functions proved to be a successful catalyst for the transesterification of ethyl acetate with benzyl alcohol,2 a model reaction for the biodiesel fabrication process.
3 The three requirements of chimie douce: coatings, monoliths and hybrids in one reaction
The ability to generate coatings and hybrids with HTC was demonstrated throughout the generation of porous materials using the nanocasting procedure [15]. Thus, using hydrothermal carbonization of glucose in the presence of different nanostructured silica templates and simply alternating the polarity of the silica surface, different carbon morphologies such as macroporous casts, hollow spheres, or mesoporous particles could be obtained. In this series, very hydrophobic silica template lead to macroporous carbonaceous films, while the moderately hydrophobic silica template lead to hollow spheres with a mesoporous surface. The dehydroxylated silica filled 60% wt with carbohydrate precursor, resulted in mesoporous spheres where most of the porosity results from the interstitial pores of the small carbon spherules coating the silica pores. Even SBA-15 could be successfully replicated using “carbohydrate sol-gel chemistry”, leading to mesoporous hexagonally ordered structures with good crystallographic meso-order, uniform pore sizes and high surface areas [15]. Some of those morphologies are shown in Fig. 2. Since all these carbohydrate based nanocasts were generated under hydrothermal conditions, there are still a large number of oxygenated functional groups residing at their surface.

Example of porous, layered and coated materials obtained using hydrothermal carbonization of glucose: a: macroporous film-cast obtained using totally hydrophobized silica templates; b: hollow spheres obtained using surface hydrophobized silica templates; c: mesoporous spherical replicas by filling mesoporous silica, d: hexagonally ordered structure obtained by nanocasting of SBA-15 [25].
Carbohydrate-based monomers formed in situ and their condensation were also successfully used for “sol-gel”-coating of preformed nanoparticles [27–29]. One particular example is the coating of silicon nanoparticles followed by further carbonization at elevated temperatures to improve conductivity [28]. Due to the hydrothermal conditions, besides the carbon coat, a thin layer of silica was simultaneously formed at the surface of the silicon nanoparticles. The resulting composite material (Fig. 3) showed – after further calcinations – a significantly improved lithium storage performance in terms of a highly reversible lithium storage capacity, excellent cycling performance and high rate capability. This is due to the fact that the carbohydrate-based coating has due to its flexibility a buffering role, minimizing the mechanical stress induced by huge volume change of active silicon, preventing thus the deterioration of the electrode integrity and keeping in the same time the high capacity associated with silicon.

TEM images of the Si@SiOx/C nanocomposite produced by “one-pot” hydrothermal carbonization and further calcination at 750 °C under N2: a: overview of the Si@SiOx/C nanocomposites and higher magnification TEM (in the inset) showing uniform sphere-like particles; b: HR-TEM image clearly showing the core/shell structure; c and d: HR-TEM image displaying local details of the silicon nanoparticles coated with SiOx and carbon.
Interestingly, this type of sol-gel chemistry to generate porous carbons and carbon nanocoatings is not restricted to pure carbohydrates, but also raw biomass plant material can be used [16]. Here, carbon rich nanostructured materials showing interesting features could be produced. Again, hydrothermal treatment first fluidizes the biomass into a “sol”, which then in later stages condenses to a carbon “gel”. Structural analysis revealed essentially two different reaction cascades, depending on the plant material used. Under the applied conditions (16 hours and 200 °C), all mechanically soft biomass (orange peels, pine needles, etc.) were completely disintegrated and transferred into the sol state, resulting in dispersions of carbonaceous nanoparticles (Fig. 4a). The droplet-like appearance of these structures indicates that the whole process indeed progresses via liquid intermediates, which then later polymerize/cyclisize to the final coal-like material.

a: SEM micrograph of the nanoparticles obtained upon hydrothermal treatment of pine needles; b: TEM picture of a porous bicontinous sponge-like network obtained upon hydrothermal carbonization of oak leafs; c: adsorption-desorption isotherm of a biomass sample before and after hydrothermal treatment.
“Hard” plant tissue with structural, crystalline cellulose (oak leafs, pine cones) disintegrated structurally along a different pathway. As the melting point of crystalline cellulose is well above its decomposition temperature, only parts of the biomass are liquefied at the same time, using the residual biomass as a scaffold and for coating. This way, the macro- and microstructure of the biological tissue is preserved to a large extent in the final carbon material. In other words: a piece of wood or a pine cone still looks like a pine cone, but on the scale of up to some hundred nanometers, complete chemical reconstitution of the biomass into a sponge-like, bicontinuous carbonaceous network with structural elements in the 20 to 50 nm range occurs (Fig. 4b). This transformation is also accompanied with the corresponding weight loss of about 50%, due to elimination and condensation.
The nanometer structure of the pore system was additionally characterized by nitrogen sorption measurements. Fig. 4c shows, as an example, the corresponding data of the pine cone hydrothermally treated. A hysteresis loop in the higher mesopore range is found, indicating the onset of the developing porous structure at a length scale of about 5 nm. Gas sorption measurements are only sensitive to pore sizes of up to 30 nm, i.e. the macropore structure if the material is not sufficiently reflected in these measurements. The corresponding measurement on the original dried pine cone material revealed no detectable porosity at all.
The production of such porous materials together with their associated applications clearly demonstrates the potential of a “chimie douce” of carbon materials based on carbohydrates and their hydrothermal decomposition products to replace same of the standard organic monomers, fulfilling the today's requirements towards greener chemistry processes and the sustainability of the starting products. It is a question for a scientific discussion if the key step of HTC is still a “douce” process: the overall chain from the starting products to the final applications to our opinion certainly is. Besides the practical free access to the starting materials (side products of nutrition), the low cost of the final materials, other advantages such as the replacement of organic solvents with water, easy and unsophisticated apparatus and reaction schemes, faster and less production steps offer almost unlimited possibilities for this exciting class of materials. In this sense, it nicely complements the previous work on transition metal oxides and silica hybrids.
1 R. Demir-Cakan, P. Makowski, M. Antonietti, F. Goettmann, M.M. Titirici, Catal. Today (submitted 2009).
2 R. Demir-Cakan, P. Makowski, M. Antonietti, F. Goettmann, M.M. Titirici, Catal. Today (submitted 2009).