Version française abrégée
Métabasaltes et métagabbros sont souvent collectés par dragages, forages ou durant des plongées en submersible sur les grands fonds des océans modernes. En revanche, les métaserpentinites sont beacoup plus rares. Les premières métabasites découvertes ont été attribuées au « métamorphisme du plancher océanique » (ocean floor metamorphism de Miyashiro [134]) et expliquées initialement comme résultant d'un métamorphisme de type regional [36]. En fait, toutes les métabasites océaniques étudiées résultent de réactions eau/roche en conditions hydrothermales entre des fluides dérivés de l'eau de mer et les basaltes, dolérites et gabbros formant les couches 2 et 3 de la croûte océanique, ou des roches ultramafiques serpentinisées provenant du manteau supérieur. Elles représentent les produits d'un large éventail d'altérations hydrothermales dans des conditions extrêmement variables quant à l'intensité du remplacement (pervasive contre non-pervasive), à la température et au rapport eau/roche.
La grande majorité des métabasites océaniques contiennent généralement des assemblages de minéraux métamorphiques semblables à ceux qui constituent les paragenèses des faciès schiste vert et amphibolite du métamorphisme régional. Cependant, contrairement à ces derniers, les roches océaniques hydrothermalisées ont rarement atteint l'équilibre, et indiquent souvent des domaines de températures fluctuant entre 150 et presque 800 °C, ainsi que des rapports eau/roche variables. Par conséquent, les minéraux secondaires formés dans différentes conditions coexistent souvent dans les métabasites océaniques. De plus, les roches océaniques hydrothermalisées sont très souvent associées à leur protolithe peu ou pas altéré, et contiennent des minéraux reliques et des textures magmatiques, indiquant que la recristallisation n'a pas été complète, parce que l'équilibre métamorphique n'a pas été atteint. Toutes ces caractéristiques confirment la nature éphémère des systèmes hydrothermaux océaniques.
Le modèle de Lister [113,114,116] de la circulation hydrothermale à travers la croûte des dorsales océaniques est présenté, parce qu'il correspond le mieux aux cas d'altération hydrothermale résumés dans le présent article. Ce modèle inclut le concept de « front de fracturation active », qui permet la pénétration de l'eau de mer jusqu'au « réacteur hydrothermal » situé au toit des chambres magmatiques à l'axe des dorsales, c'est-à-dire la transition entre les dykes doléritiques de la partie inférieure de la couche 2 et les gabbros de la couche 3 sous-jacente (Fig. 1a). Lister a proposé une évolution en quatre stades du système hydrothermal axial (Fig. 1b). Dans la plupart des modèles, y compris celui de Lister, la circulation hydrothermale est limitée à la couche 2 basaltique et doléritique de la croûte océanique, alors que la couche 3 gabbroı̈que, plus profonde, est considérée comme si elle n'était pas affectée par l'hydrothermalisme.

(a) Lister's cartoon, [113], of his one-dimensional model of water penetration into hot rock through ‘active cracking’ creating the permeability in the roof of magma chambers at the oceanic ridge axes. T1=the heat source, i.e., an undisturbed hot rock, crystal mush or the magma in the roof of the magma chamber; TK=thin conductive layer ahead of the cracking front where the hot rock cools, shrinks, and the build up of tension needed to cause the cracks to advance; TW=the cracked zone where a hydrothermal cell convects; T0=the reservoir of cold seawater which includes the water column, the pelagic sediment cover (Layer 1), and the highly permeable ‘aquifer’ in the uppermost part of the layer 2 of the oceanic crust. (b) The four successive stages of Lister's model, [114], of the evolution of a hydrothermal system at oceanic ridge axes. Stage 1: initial hydrothermal cell with a cold descending limb (recharge) and a warm ascending limb (discharge). The main components are the same as in (a). Stage 2: the hydrothermal system has evolved into two superimposed convection cells: a lower hot one (that has no discharge onto the seafloor), and an upper one (that discharges warm waters into the water column). Stage 3: the mature hydrothermal system includes a lower ‘very hot’ convection cell without discharge (affecting the host layer 3 rocks with a hydrothermal alteration in the conditions of the amphibolite facies), and a twinned upper cell discharging warm waters (i.e., at about 400 °C) onto the seafloor. The two cells are separated by a sulfide stockwork. Stage 4: the fossil hydrothermal system. Note: the scales “maybe several kilometers for the depth of the cracked zone, a few meters for the conductive boundary layer, and about one meter for the primary cracking spacing” [112].
(a) Schéma du modèle unidimensionnel de la pénétration de l'eau de mer dans une croûte océanique chaude par fissuration active (active cracking) qui crée une perméabilité dans le toit des chambres magmatiques à l'axe des dorsales océaniques [113]. T1=la source de chaleur, c'est-à-dire une roche chaude non fissurée, un ‘crystal mush’ ou du magma ; TK=la mince couche conductive à l'avant du ‘cracking front’ où la roche chaude se refroidit, se contracte, où s'accumulent les contraintes nécessaires à la progression des fissures ; TW=la zone fissurée où la cellule hydrothermale convecte ; T0=le réservoir d'eau de mer froide qui comprend la colonne d'eau, la couverture de sédiments pélagiques (Couche 1), et l'‘aquifer’ formé par la partie la plus superficielle fortement perméable de la Couche 2 de la croûte océanique. (b) Les quatre stades successifs du modèle de Lister [114] de l'évolution d'un système hydrothermal à l'axe des dorsales océaniques. Stade 1 : cellule hydrothermale initiale constituée d'une branche descendante d'eau de mer froide (recharge) et d'une branche ascendante de fluides chauds (décharge) ; les principales parties constitutives sont les mêmes que dans (a). Stade 2 : le système hydrothermal a évolué et s'est transformé en deux cellules de convection superposées : une cellule inférieure chaude (qui ne débouche pas sur le plancher océanique) et une cellule supérieure qui émet des fluides « chauds » dans la colonne d'eau de mer. Stage 3 : le système hydrothermal mature comprend une cellule de convection inférieure « très chaude » sans debouché sur le plancher océanique et où les roches de la Couche 3 encaissante sont altérées dans les conditions du faciès amphibolite, et deux cellules jumelles supérieures qui émettent des eaux chaudes (environ 400 °C) au niveau du plancher océanique. Ces deux cellules sont séparées par un stockwerk de sulfures. Stade 4 : le système hydrothermal fossile. NB: Les échelles sont « possiblement de plusieurs kilomètres pour la profondeur de la zone de fissuration, quelques mètres pour la couche frontière conductive, et environ un mètre pour l'espacement des fissures primaires » [112].
Un système hydrothermal simplifié d'une dorsale océanique peut être considéré comme comportant cinq parties constitutives essentielles (Fig. 2) : (1) une source de chaleur représentée par la chambre magmatique axiale contenant du magma basaltique ou un crystal mush en cours de solidification par refroidissement ; (2) un réservoir de fluides pouvant être le siège d'une circulation hydrothermale, constitué, grâce à sa grande perméabilité, par la couche 2 de la croûte océanique ; (3) une « zone de recharge », à travers laquelle descend par gravité l'eau de mer des grands fonds, qui va se réchauffer au contact de la source de chaleur et réagir avec les roches crustales ; (4) un « réacteur hydrothermal », foyer des interactions eau/roche entre l'eau de mer et les roches formant la croûte océanique – le réacteur se situerait au niveau des racines des dykes, c'est-à-dire à la transition entre les couches 2 et 3 de la croûte océanique – ; (5) une « zone de décharge », comportant les remontées (upwelling) essentiellement adiabatiques des fluides hydrothermaux vers le plancher océanique où ils sont émis dans la colonne d'eau, soit de façon focalisée et à hautes températures (320–400 °C) par les évents des « fumeurs noirs », soit de façon diffuse et à basse température (250 à<100 °C) sous forme de « diffuseurs ».
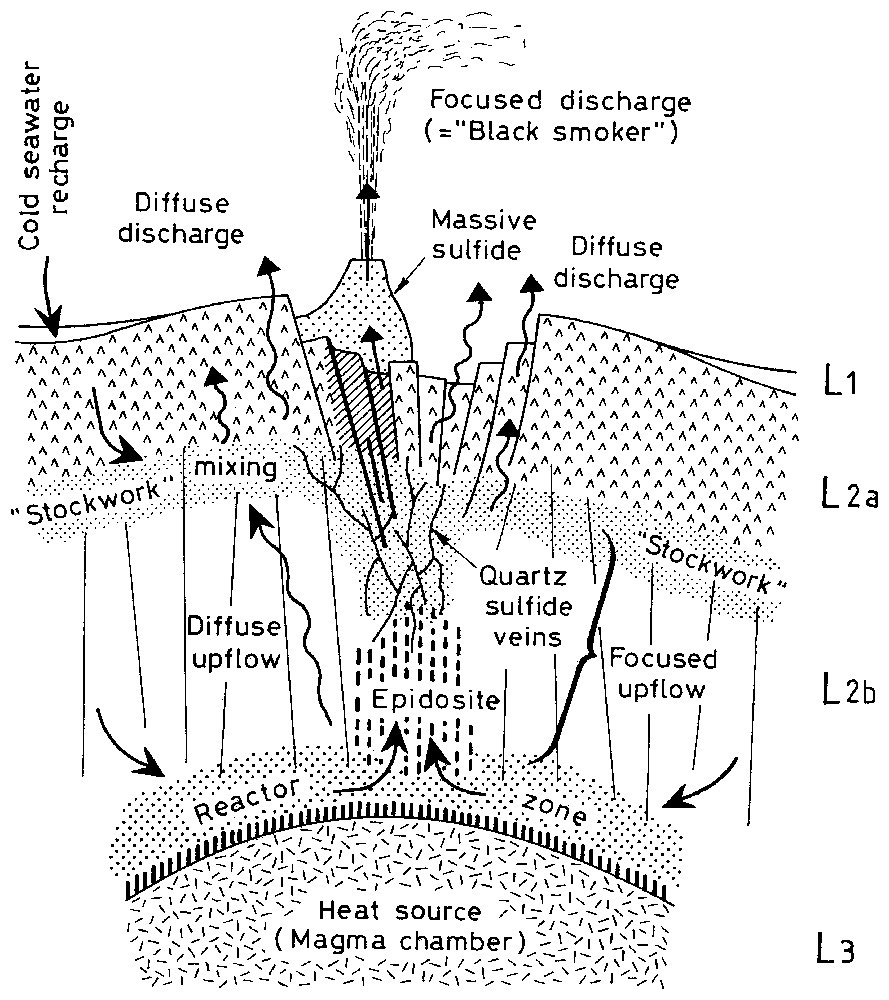
Schematic cartoon of a hydrothermal system at the axis of a slow-spreading oceanic ridge (after Alt [1]). L1=pelagic sediment cover; L2a=highly permeable lava flows forming the uppemost part of Layer 2; L2b=sheeted dyke complex forming the lower part of Layer 2. Notice both focused and diffuse discharges of hot and lukewarm hydrothermal solutions, respectively, and the epidosites in the reactor zone. The vertical distance between the seafloor and the roof of the magma chamber (i.e., the heat source) is about 1.5–2 km.
Schéma d'un système hydrothermal à l'axe d'une dorsale océanique lente (d'après Alt [1]). L1 : couverture de sédiments pélagiques ; L2a=partie la plus superficielle et fortement perméable de la Couche 2 formée de coulées de lave ; L2b=complexe filonnien formant la partie inférieure de la Couche 2. À remarquer les émissions focalisées et diffuses de fluides hydrothermaux respectivement chauds et tièdes et les épidosites de la zone du « réacteur ». La distance verticale entre le plancher océanique et le toit de la chambre magmatique jouant le rôle de source de chaleur est d'environ 1.5–2 km.
L'altération hydrothermale des gabbros de la couche 3 s'effectue dans un domaine de température commençant à un maximum proche de 800 °C (c'est-à-dire dans les conditions du faciès amphibolite) et se terminant à 350 °C (c'est-à-dire dans les conditions de la transition entre les faciès amphibolite et schiste vert), avec des rapports eau/roche généralement inférieurs à 1 [68,69,110,120,121,185,192].
Les métabasaltes présentent rarement des structures de déformation, excepté quand ils proviennent de failles transformantes où ils sont fortement cataclasés et subissent même des mylonitisations polymétamorphiques [79]. En revanche, les métagabbros peuvent parfois être fortement déformés par les cisaillements plastiques qui se développent lorsque les chambres magmatiques commencent à refroidir à l'axe des dorsales, dans les conditions quasi anhydres du faciès granulite [74,131]. Ce stade se poursuit par la transformation des gabbros en amphibolites gneissiques, alors que l'eau de mer pénètre à travers les gabbros en voie de refroidissement [81,131]. Finalement, une déformation cassante se produit dans les gabbros à des temperatures encore plus basses, dans les conditions de la transition entre les faciès amphibolite et schiste vert. Les métagabbros déformés en régime plastique semblent être plus fréquemment collectés sur les rides lentes que sur les dorsales rapides.
Avec plus de 2,1 km de profondeur, le puits 504B du DSDP/ODP, dans l'Est Pacifique équatorial (Fig. 3a), est la section de référence de la couche 2 de la croûte océanique formée de coulées basaltiques et de dykes doléritiques, même si aucun gabbro n'a été collecté au fond du puits [7 (Fig. 3b)]. La couche de 0,5 km de basaltes formant la partie la plus superficielle de la croûte a subi une altération à basse température [109], tandis que les basaltes et dolérites formant l'épaisseur de 1,5 km inférieure ont été altérés dans des conditions hydrothermales. Les interactions eau/roche ont fluctué au cours du temps avec des températures atteignant presque le maximum de 400 °C durant le premier stade d'altération de la partie la plus profonde des dykes, et tombant à 125–195 °C pendant le dernier stade d'altération, qui est probablement encore actif actuellement [5]. Les rapports eau/roche ont également varié au cours du temps et suivant la perméabilité primaire des formations géologiques, qui était de 70 mD dans les coulées superficielles, mais de deux ordres de grandeur plus faible dans les dykes inférieurs. Les métadolérites altérées durant le premier stade d'altération contiennent des assemblages de minéraux secondaires semblables à ceux de la transition entre les faciès schiste vert supérieur et amphibolite, alors que les basaltes surincombants voyaient se former des assemblages minéraux, correspondant au faciès schiste vert inférieur. Durant le deuxième stade d'altération, un stockwerk de sulfures poltymétalliques (Fe, Cu, Zn avec traces de Pb) a précipité dans la zone de transition entre les basaltes et les dolérites [82]. Les métabasaltes encaissants de la minéralisation étaient localement silicifiés et pyritisés. Le dernier stade d'altération, pratiquement limité à des veines, a commencé par des zéolites (analcite, laumontite), de la préhnite, et s'est terminé par de la calcite. Il s'est produit à des températures allant de 250 à 150 °C. Les métabasaltes et métadolérites ne présentent pas de déformations, mais sont affectés par de nombreuses fissures primaires le long desquelles ont circulé les fluides hydrothermaux qui ont réagi avec les roches adjacentes. Des minéraux primaires reliques ont survécu durant les trois stades d'altération hydrothermale.

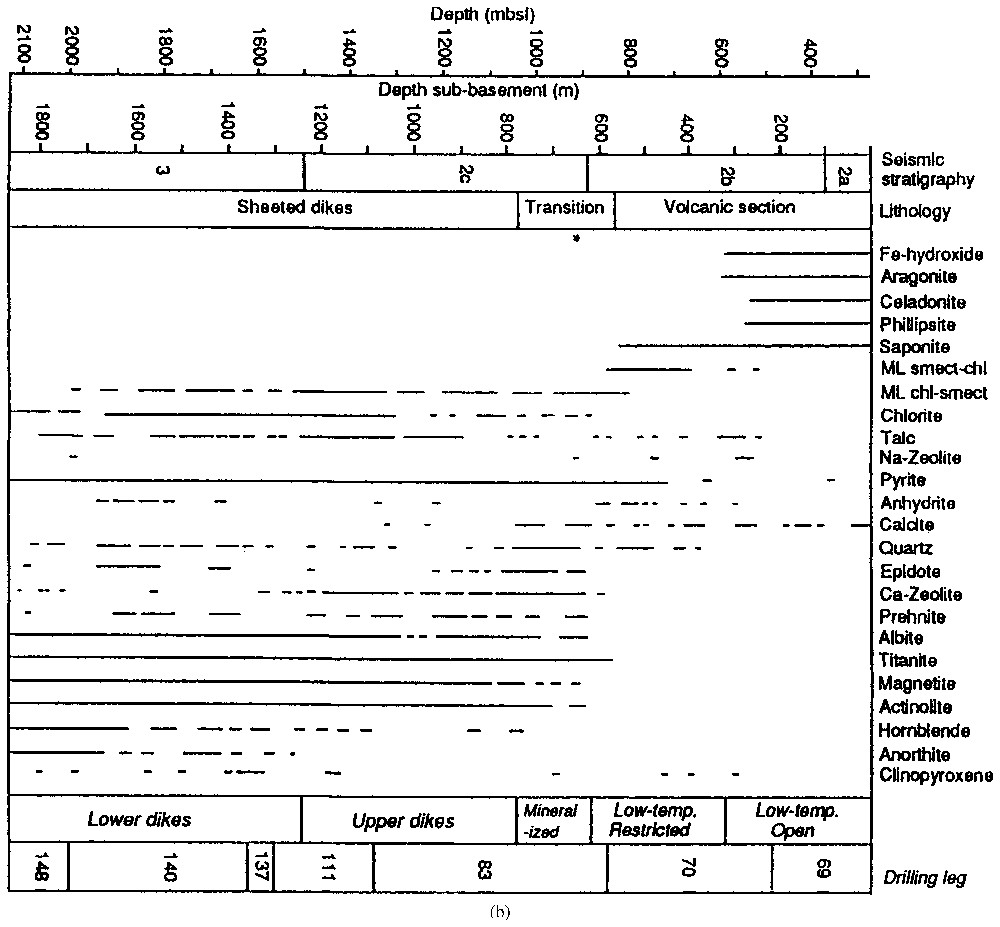
(a) Geographical and geotectonic location of DSDP/ODP Hole 504B in the Eastern Equatorial Pacific (after Alt et al. [7]). (b) Lithostratigraphy and distribution of secondary minerals in DSDP/ODP Hole 504B. Also shown at the right are the penetration depths of the successive drilling legs. An asterisk indicates the location of the stockwork-like sulfide mineralization in the lithological transition zone. ML=mixed layer phyllosilicate; chl=chlorite; smect=smectite (after Alt et al. [7]). Already published by OPD publication services.
(a) Situation géotectonique et localisation géographique du puits 504B du DSDP/ODP dans le Pacifique oriental équatorial (d'après Alt et al. [7]). (b) Lithostratigraphie et répartition des minéraux secondaires dans le puits 504B du DSDP/ODP. À droite, sont indiquées les profondeurs de pénétration atteintes par les campagnes de forage successives. L'astérisque indique l'emplacement des minéralisations sulfurées de type stockwerk dans la zone de transition lithologique. ML=phyllosilicates interstratifiés; chl=chlorite; smect=smectite (d'après Alt et al. [7]).
En revanche, les métabasites profondément hydrothermalisées ou complètement remplacées sont bien moins fréquentes que celles dont il vient d'être question ci-dessus. Ces roches semblent être limitées aux zones de remontée (upwelling) des fluides des systèmes hydrothermaux, où des solutions hydrothermales de haute température sont émises sur le plancher océanique. Un tel cas de métasomatisme hydrothermal est représenté par le stockwerk sous et dans le mont hydrothermal actif du TAG, à l'axe de la dorsale médio-Atlantique [83 (Fig. 4a)]. Dix-sept puits forés dans le mont du TAG durant le leg 158 d'ODP ont permis de reconstituer (Fig. 4b) la zonation verticale et horizontale de l'altération du stockwerk situé à quelques mètres autour de la remontée des fluides hydrothermaux qui sont actuellement émis à 368 °C par le complexe de « fumeurs noirs » situé au sommet du mont. À plus de 120 m de profondeur sous la surface du mont, un stockwerk de brèches métabasaltiques, totalement chloritisées, cède progressivement mais rapidement la place à un stockwerk de brèches basaltiques, totalement remplacées par des assemblages pyrite+quartz+paragonite et cimentées par de la pyrite et du quartz, auxquels se joint l'anhydrite plus près de plancher océanique. Les cas de métabasaltes fortement silicifiés et/ou argilisés et/ou séricitisés d'autres localités, comme la ride de Gorda, Pacifique nord-est [213], ou du fore-arc d'Isu-Bonin-Mariana [8], ou le cas des épidosites du fore-arc des Tonga, Pacifique occidental [13], sont comparés à leurs équivalents du mont du TAG.


(a) Geographical location and bathymetric map of the TAG active hydrothermal field. T=TAG active mound; LT=low temperature active hydrothermal site; MIR and ALVIN=inactive mounds (after Honnorez et al. [83]). (b) Reconstructed cross section through the TAG mound showing the zoned distribution of the various alterations of the basaltic basement. 1=regional depth of the seafloor west and north of the mound at 3680 m below sea level (mbsl); 2=top of the basement in Hole 957M at 3687 mbsl; 3=line showing the shallowest level at which altered basalt clasts were recovered; 4=top of basement in Hole 957B at 3672 mbsl; 5, 8=basalt samples recovered post-drilling; 7=basalt with chloritized halos recovered pre-drilling (after Honnorez et al. [83]). Already published by OPD publication services.
(a) Localisation géographique et carte bathymétrique du champ hydrothermal actif du TAG. T=mont actif du TAG ; LT=site d'activité hydrothermale à basse température ; MIR et ALVIN=deux monts inactifs (d'après Honnorez et al. [83]). Profil reconstitué à partir des 17 puits de forage à travers le mont actif du TAG et montrant la zonation des divers types d'altérations du socle basaltique. l=profondeur régionale du plancher océanique à l'ouest et au nord du mont, à 3680 m sous le niveau de la mer (mbsl); 2=sommet du socle atteint dans le puits 957M, à 3687 mbsl; 3=ligne indiquant le niveau le moins profond auquel des clastes de basalte altéré ont été observés ; 4=sommet du socle atteint dans le puits 957B, à 3672 mbsl. 5, 8=échantillons de basaltes collectés après le forage ; 7=basaltes présentant des halos chloritisés échantillonnés avant le forage (d'après Honnorez et al. [83]).
Les deux cas du puits 504B et du mont du TAG illustrant des exemples contrastés d'altérations hydrothermales des métabasaltes de la couche 2 de la croûte océanique sont comparés aux cas d'altération des gabbros de la couche 3 sélectionnés dans la littérature, comme la section de gabbros forée dans le puits 735B du DSDP/ODP et qui a été générée par la dorsale lente SW-Indienne [91,201], ou celle forée avec les puits 749 F and G du DSDP/ODP dans la fosse de Hess mise en place à la dorsale Est-Pacifique, une dorsale rapide cette fois [68,96].
Les occurrences de métabasites hydrothermalisées de façon non pénétrative (non pervasively altered) sont largement répandues sur le plancher des océans actuels, et non pas limitées à des cadres tectoniques spécifiques comme les failles transformantes ou normales. Ceci signifie que ces métabasites forment probablement une partie importante de la croûte océanique. Au contraire, les occurrences de métabasaltes ou métagabbros hydrothermalisés de façon intense et pénétrative (pervasively replaced) sont relativement beaucoup plus rares et limitées en extension, si bien que ceux-ci ne peuvent constituer une fraction importante de la croûte océanique. Néanmoins, de telles roches sont des indicateurs métallogéniques très utiles, car elles sont très semblables aux laves diversement hydrothermalisées qui sont associées aux gisements de sulfures massifs volcanogéniques land-based (par exemple, [213]) qui sont exploités à terre pour leurs métaux non ferreux et leur or.
1 Introduction
The oceanic crust represents the most extensive igneous formation of the Earth crust even though the oldest basement of the present day ocean is only 165 Ma old. Since the time of its emplacement by accretion along the 60 000 km of active oceanic ridges which circumscribe the Earth, until its recycling into the mantle by subduction, the oceanic crust is affected by various alteration processes resulting from its interactions with seawater at seafloor conditions (i.e., ‘submarine weathering’), and seawater-derived fluids in hydrothermal conditions. The successive seawater/oceanic crust interactions are thought to play an essential role in participating to the world ocean budget and regulating the chemical composition of seawater. With 18×1021 kg of seawater, the world ocean water represents 94.7% of the total water reserve of the Earth. It is the largest reservoir of fluids involved in submarine water/rock interactions particularly as compared with the mid-oceanic ridge magmas, which only contain less than 0.4% of H2O+. Hence magmatic volatiles cannot be considered as important contributors to submarine alteration processes of oceanic crustal rocks.
Occurrences of metamorphic rocks derived from the oceanic crust and upper mantle are widely distributed through the various geodynamic settings of the world seafloor. Samples of metabasalts, metagabbros or metaserpentinites have been recovered at numerous locations, e.g., by dredging from fault scarps of oceanic rift valleys or transform faults, and by drilling into the oceanic crust at ridge axes and flanks. Now we know that these metabasites result from various hydrothermal interactions between seawater-derived fluids and the rocks forming the oceanic lithosphere. Oceanic metabasites were found [40,124,151,207] long before submarine hydrothermal activity was directly observed at oceanic ridges in the late 1970s [45,64,182]. Serpentinites, ‘spilites’, amphibolites, ‘diabases’ and other metabasites were already known to be essential constituents of the ophiolite ‘trinity’, since the first definition of the term by Steinmann [187,188]. Ophiolite complexes are now recognized to represent sections of ancient oceanic lithosphere emplaced upon continental crust by dominantly thrusting mechanisms during plate collision ([43,148,149] and references therein). However, this article will exclusively deal with metabasites originated in the present day ocean floor, and ophiolites will only occasionally be quoted as comparison terms.
Thanks to the collaborative efforts of the various ongoing international programs such as ODP (Ocean Drilling Program), InterRidge, DORSALES, etc., we know that hydrothermal circulation through the oceanic crust is a widespread phenomenon related to the generation of new oceanic lithosphere by accretion at spreading centres and above hot spots. It is responsible for an important heat loss from the Earth's interior [186], and the resulting interactions between seawater and oceanic rocks which represent one of the major mechanisms regulating the chemistry of the ocean [1,58]. In addition, hot springs discharged on the seafloor supply energy and food to colonies of chemiothrophic organisms, whose symbiota are used as model for the origin of life in the Earth primitive ocean (e.g., [56,75]), and for the search of life in the solar system (e.g., [14,15]). It is now clear that anaerobic microbes exist within submarine hydrothermal systems supported by degassing and alteration reactions (e.g., [52,191]).
However, to date, it is still difficult to reconstruct the geometry of the fluid recharge and discharge pathways of hydrothermal circulation through the oceanic crust, to assess the relative importance of ridge axis vs. ridge flank hydrothermal circulations and the relative contribution of focused vs. diffuse fluid discharges, and to describe the evolution of a given submarine hydrothermal system with time. Finally, the geochemical fluxes and budgets resulting from the various alteration processes are still uncertain for most of the major and trace chemical elements.
It will be shown in this article that all of the oceanic metabasites are more or less intensely altered rocks that result from hydrothermal water/rock interactions between the oceanic crust and seawater-derived solutions. However an entire range of hydrothermal alteration intensities is encountered among the samples collected form the seafloor. On the one hand hydrothermally altered metabasalts and metagabbros can be the products of localized but intense and pervasive water/rock interactions in crustal locales such as stockworks proximal to the focused discharges of seawater-derived hydrothermal fluids. Such rocks are similar to hydrothermally altered host-rocks of land-based sulphide ore deposits. Their primary phases are almost totally replaced by secondary minerals, their igneous texture might be obliterated, and their chemical composition is strongly metasomatic. On the other hand, the so-called ‘ocean-floor metamorphism’ generally corresponds to non-pervasive and not so intense water/rocks interactions in widespread regions away from the focused hydrothermal upwelling zone. In such rocks the replacement of primary phases is generally only partial, igneous relict minerals and structures are quite abundant, and the chemical composition is not very different from that of their protoliths. The comparison between studies of the TAG (Trans-Atlantic Geotraverse) active hydrothermal mound of the Mid-Atlantic Ridge, and DSDP drilled Hole 504B into the 5.9-Myr-old flank of the Costa Rica Ridge (Equatorial East Pacific) leads to a better understanding of the wide range of alteration mechanisms of the oceanic crust, and of the spatial and temporal distributions of the various types of metabasalts associated with submarine hydrothermal activity. These two case histories are completed by similar examples drawn from the literature.
A series of special issues dedicated to various aspects of ocean floor hydrothermalism have been published up to the present time. They are actual mines of information about alteration systems and processes, their effects and products. They cannot all be listed here but it is highly recommended to consult the following monographs: Nato Conference Series, Marine Sciences [157]; The Canadian Mineralogist [14]; Economic Geology [156]; US Geological Survey Bulletin [138]; The Geological Society [147]; Geophysical Monographs [91]; The Royal Society [41].
2 Ocean floor metamorphism vs. hydrothermal alteration: pervasive vs. non-pervasive or proximal vs. distal processes?
The first oceanic metabasalts were collected from fault scarps associated with the crest of slow spreading oceanic ridges such as the Mid-Atlantic Ridge [124–126] or the Carlsberg Ridge, in the Indian Ocean [38,40,207]. They were called ‘greenstones’ or ‘spilites’, and initially interpreted as the result of “burial-regional metamorphism rather than of localized hydrothermal alteration, or of autometamorphism” [125]. However, only two years later, Melson et al. [126] proposed that the same ‘greenstones’ from the Mid-Atlantic Ridge were the product of a “post-cooling (of the lavas) hydrothermal metamorphism”. Similarly, the ‘spilites’ form the Indian Ocean [38,40] were considered as “metamorphosed and hydrothermally altered rocks […] formed by the action of hot fluids on already crystalline rocks”. Oceanic metabasalts were then explained as the result of “burial at a depth of about one kilometer or less, coupled with a rise in temperature to about 200 °C (which) would bring the rocks into the lower greenschist facies of metamorphism” [40]. Concluding about the ‘spilites’ from the Carlsberg Ridge, Cann [38] finally wrote: “these spilites were formed by low-grade metamorphism, and were neither the products of reaction of hot basalt with sea water nor that of the crystallization of a special spilitic magma […] (but they were) buried underneath later lava to be heated sufficiently for an assemblage corresponding to the lower greenschist facies to be formed”. Miyashiro et al. [134] and Miyashiro [135] coined the term ‘ocean-floor metamorphism’, which they regarded “as a kind of burial metamorphism in high heat-flow regions of the oceanic ridge crests”. The authors ascribed the metamorphic reactions to either the presence of igneous intrusions or the flow of hot fluid. In the latter case, the agent of ‘ocean-floor metamorphism’ would have been hydrothermal. In the most recent revised edition of Winkler's Petrogenesis of metamorphic rocks, Bucher and Frey included Miyashiro's ‘ocean-floor metamorphism’ in the ‘regional-extent’ type of metamorphism along with ‘orogenic’ (synonymous of ‘regional’) and ‘burial metamorphisms’ [36 (pp. 5–6)]. Nevertheless, the authors concluded the paragraph dedicated to this subject with the following statement: “This process [of ocean-floor metamorphism] leads to chemical exchanges between rocks and sea water; in this respect, ocean-floor metamorphism resembles hydrothermal metamorphism.” Indeed, ‘ocean-floor metamorphism’ is a case of hydrothermal alteration.
As a matter of fact, it had already been concluded, as early as 1973, that metagabbros [29], rodingites [78], and chalcopyrite mineralizations disseminated in greenschist metabasalts [30] dredged from the Mid-Atlantic Ridge resulted from a ‘contact hydrothermal metamorphism’ due to interactions between seawater and the oceanic crust beneath the axis of oceanic ridges, in the vicinity of axial magma chambers acting as heat sources. Similarly, Humphris and Thompson [88,89] proposed that the metabasalts from the median valley of the Mid-Atlantic Ridge at 4°S and 22°S latitude, which exhibit a secondary mineral assemblage similar of the parageneses of greenschist facies of regional metamorphism, resulted from hydrothermal alteration.
However, the equivalent to the hydrothermally altered host rocks surrounding the large land-based volcanogenic massive sulphide (VHS) ore deposits such as, for instance the propylitized, sericitized, chloritized, or argillized lavas, have been rarely collected from the present-day oceanic crust. Such rocks are generally pervasively altered with their primary igneous mineralogy completely replaced and their chemical composition totally changed. Quon and Ehlers [151] described one quartz+epidote rock sample with relict basaltic texture from the North Mid-Atlantic Ridge that could be called an epidosite, but the sample was never studied in detail, and has now been lost. Epidosites are rocks made up of epidote and quartz, with minor chlorite and pyrite. They are depleted in transition metals and generally lack the parental rock igneous texture. The first and only present-day ocean epidosites were dredged from the Tonga fore-arc [13]. The authors concluded that these epidosites metasomatically replaced basaltic and plagiogranite protoliths, and formed under similar conditions to epidosites found in ophiolite complexes. The latter are known but not widespread in various ophiolite complexes (e.g., [145,153,161]) where they occur as veins, mainly in the lower part of the sheeted dike complexes. According to Banerjee et al. [13], the tectonic setting would play a role in the formation of epidosites since both Tongan and ophiolite epidosites were collected from locales related to subduction zones, and none from mid-oceanic ridges. In addition to epidosites rare occurrences of chloritized or ‘sericitized’ metabasalts have been collected from active and inactive submarine hydrothermal fields in the present-day ocean. They will be described and their origin will be discussed in the last section of this paper. Intensely altered oceanic metabasites such as epidosites, and sericitized or chloritized metabaslts are present in the modern oceanic crust but they are very restricted in abundance and extent. Such rocks probably do not form a major part of the oceanic crust.
Both the widespread metabasalts and metagabbros ascribed to ‘ocean-floor metamorphism’, and the rare epidosites, chloritized or ‘sericitised’ basalts, and rodingitized gabbros result from hydrothermal interactions between the oceanic lithosphere and seawater-derived fluids. They differ in the degree of alteration and, hence, in the nature of the end products. The former are non-pervasively altered and abundant relict minerals subsist, whereas the latter are pervasively and intensively replaced. The former result from distal alteration processes away from the up-flow zone of hydrothermal fluids, whereas the latter result from proximal processes, in the vicinity of focused discharges of hydrothermal fluids.
3 Misusing Eskola's concept of metamorphic facies
Similarities between the mineral assemblages of oceanic metabasites and the parageneses of land-based metamorphosed rocks resulting from burial or regional metamorphisms has lead Eskola's metamorphic facies concept to be applied to seafloor and ophiolites metabasites. Ophiolites were commonly presented as vertically zoned sections of oceanic crust with a progressively increasing degree of metamorphism with burial depth in the complex, ranging from zeolite, to greenschist, and to amphibolite facies of metamorphism (e.g., [54,66,67,190]). It “led to the view of metamorphic facies boundaries laying more or less parallel to the original ocean floor […] The degree of alteration is more or less uniform and extensive” [39]. The term “brownstone facies” was even introduced by Cann [39] for oceanic basalts altered at low temperature in oxidizing conditions during ‘submarine weathering’, i.e., halmyrolysis, a non-metamorphic process, in the uppermost portion of the seafloor (for a review of low temperature alteration of oceanic basalts, see [76]). However, already in 1979, Stern and Elthon [189] presented a more refined model whereby “disequilibria retrograde assemblages” were noticed as being the common rule. It was also observed that basalts displaying the mineralogical assemblages characteristic of the prehnite–pumpellyite facies were rarely found in the seafloor, but were more frequent in ophiolites. Prehnite alone is common in oceanic metagabbros (e.g., [78,129]), but pumpellyite has only been observed three times in ocean-floor metabasalts [93,120,128]. Pumpellyite was associated with prehnite in two of these three oceanic occurrences.
In this paper, the expression ‘greenschist-like (or another) facies mineral assemblage’ will be used to designate a non-equilibrium mineral assemblage similar to the diagnostic mineral equilibrium (or near-equilibrium) assemblage, characteristic of the various facies of regional metamorphism.
The main arguments supporting that ocean-floor metabasites are the product of hydrothermal interactions between crustal rocks and seawater-derived hydrothermal fluids are the following.
- (1) In crustal regions with average heat flow, the shallow burial depth below the seafloor surface at which the oceanic metamorphic rocks were collected prevents high enough temperatures to be reached, which would correspond to the observed metamorphic mineral assemblages during burial-regional metamorphism. On the other hand, much higher heat flows are locally measured along the ridge crests or ridge flanks as compared to those normally measured in deep basins away from spreading centres.
- (2) Non-equilibrium mineral assemblages are generally observed in oceanic metabasites. In opposition to Cann's experience, who claimed that “it is possible to conclude from the close spatial juxtaposition and wide development of secondary phases that they represent some sort of equilibrium assemblage” [39], the presence and often, the abundance, of primary basaltic relict minerals along with assemblages of secondary minerals belonging to various parageneses characteristic of different metamorphic facies, indicate that non equilibrium conditions prevailed during replacement (see also [189]).
- (3) In addition, it is observed that the metamorphic assemblages are generally restricted to open space fillings such as cracks, vesicles and vugs, and or alteration halos replacing rock adjacent to veins. Multiple openings and in-fillings of the veins are also commonly observed.
- (4) Finally, it will be shown later that the chemical compositions of hydrothermal solutions collected from present-day ocean floor hot springs, clearly indicate hydrothermal interactions between crustal rocks and seawater-derived fluids.
These observations indicate that the sea floor water/rock interactions were related to recurrent but short-lived circulations of exogenous hydrothermal fluids along open fractures, and that they did not produce equilibrium metamorphic mineral precipitations in the voids nor complete recrystallizations of the host rocks.
One can conclude that the oceanic metabasites are not the result of the classical burial-regional metamorphism, which leads to the replacement of protolites by equilibrium mineral assemblages. Hence Eskola's concept of metamorphic facies cannot be directly applied to the oceanic crustal context. In addition, it can be concluded that seafloor metabasites are not derived from primary ‘spilitic’ magmas since the metabasalts result from post-eruptive seawater-MORB reactions in hydrothermal conditions. This was the ultimate argument that put an end to the theory of the primary origin of spilites (e.g., [9] and references therein).
4 Deformation and schistosity in oceanic metabasites
Miyashiro et al. [135] and Miyashiro [134] noticed that the vast majority of the oceanic metabasites are non-schistose and the degree of cataclasis not extreme except for a small number of ‘gneissic metagabbros’ from the Mid-Atlantic Ridge at 30°N (already mentioned by Quon and Ehlers [151] and Thayer [195]) which were explained as the products of “contact metamorphism in the lower crust or the upper mantle”. It was shown later (Miyashiro, in litt. and unpublished) that these ‘gneissic metagabbros’ were ice-rafted rocks of Precambrian age probably derived form the Labrador continental shield. However, actual ‘gneissic amphibolites’ exist in the oceanic crust [81] (see below).
On the other hand, strongly deformed metabasites and metaserpentinites were collected from transform faults that intersect and offset the slow spreading mid-oceanic ridges. For instance, gneissic amphibolites from the Vema Fracture Zone (Mid-Atlantic Ridge at 11°N) were demonstrated to result from the complete recristallization of Layer-3 gabbros at temperatures comprised between 400 and 650 °C, and with low water/rock ratios [81]. They are only about 10 Myr old and result from a dynamic, contact metamorphism of gabbros in the lower oceanic crust (Layer 3) that completely re-equilibrated in the conditions of the amphibolite facies along an active transcurrent fault [81]. Similarly, mylonites, ultramylonites and phyllonites were recovered, along with dominant, undeformed or cataclastic metabasites, from the Romanche Fracture Zone that offset by 950 km the Mid-Atlantic Ridge in its equatorial portion. The deformations took place under the conditions of the greenschist to amphibolite facies [77,79]. However, strongly deformed and recrystallized oceanic metagabbros do not appear to be restricted to transform faults where transcurrent tectonics generates crustal shear zones. Metagabbros collected from the other geotectonic settings of the seafloor display cataclastic textures.
Mével and Cannat [131] studied gabbros samples collected from slow spreading ridges by dredging or by submersible (e.g., the MARK area in the Mid Atlantic Ridge South of the Kane Fracture Zone) or through low-recovery drilling (e.g., DSDP hole 334 [75], and DSDP hole 556 [130]) which do not provide continuous, in situ sections of the lower oceanic crust. For such gabbro samples, no correlation between rock deformation textures and outcrop tectonic features can be established. The authors also studied gabbro samples from ODP hole 735B (in the southwestern Indian Ocean), which supplied them with a more continuous set of samples [55]. Mével and Cannat [131] observed that the metagabbros from ODP hole 735B are remarkably similar to the dredged gabbros with respect to their secondary mineralogy, and texture. Even though their study samples were collected in the vicinity of transform fractures zones, Mével and Cannat [131] claimed that “the geometry of deformational structures in these samples (inferred from submersible observations or from the drill cores) is not compatible with strike-slip deformation in the transform fault. Therefore, we infer that these deformational structures resulted from tectonic processes occurring at the ridge axis.” The metagabbros “are often plastically deformed […] from slightly deformed with abundant magmatic porphyroclasts in a recrystallized matrix, through gneissic, to truly mylonitic.” Mével and Cannat [131] presented a three-stage evolutionary model of the deformation and alteration of the gabbros forming the deeper crustal portion of slow-spreading ridges, i.e., during periods of low magma supplies (see Section 6.3). According to these authors, oceanic stretching of the gabbros starts right after their emplacement at or close to the spreading axis where synkinematic ductile deformation generates shear planes at temperatures ranging from 800 to 900 °C, i.e., in anhydrous conditions similar to those of the granulite facies. When the crust spreads away from the ridge axis, oceanic gabbros are successively deformed and altered as the rock temperature decreases and continuing shearing associated with synkinematic ductile cracks allow seawater derived fluids to penetrate and react with the rocks at temperature ranging from 750 to 500 °C, i.e., similar to the amphibolite facies conditions [185,201]. Later and farther away form the ridge axis, seawater penetrates and reacts with the gabbros, at temperatures lower than 500 °C, along brittle deformation cracks of Lister's cracking front (see Section 6.2). Mével and Cannat [131] proposed that the amount of ductile shearing and related high-temperature alteration in oceanic Layer 3 might well be a function of the spreading rate.
5 Are metabasites a major component of the oceanic crust?
Various types of metabasites have been commonly recovered from taluses along the lower walls of the axial rift valley of slow spreading ridges, or from the numerous transform faults that intersect the latter. The oceanic metabasites include metabasalts with mineral parageneses resembling those of the greenschist facies, and metagabbros, rodingites and metaserpentinites with mineral assemblages similar or nearly identical to the diagnostic greenschist to amphibolite facies. In fast-spreading ridges, on the other hand, metamorphic rocks are only known from the deepest transform faults (e.g., the Garret Fracture Zone [19]), or peculiar tectonic settings such as the Hess Deep [68,103,121], and drill sites related to hydrothermal fields ([18] and ODP Sci. Contr. Legs 139 and 168). Hence the recovery of oceanic metabasites was thought to be generally restricted to strongly faulted tectonic settings of oceanic ridges until metabasalts with mineral assemblages like those of greenschist to zeolite facies were recovered by drilling DSDP Hole 504B into the 5.9-Myr-old flank of the Costa Rica Ridge, in the Equatorial East Pacific (see Section 7). Metamorphic rocks collected by drilling into ridge flanks or by dredging along fracture zones have probably been laterally transported by seafloor spreading away from the accretion zone at ridge axes. Later large vertical tectonic movements characteristic of slow spreading ridges, such as the Mid-Atlantic Ridge and the Carlsberg Ridge in the Indian Ocean, would bring up the metamorphic rocks to exposures where they can be collected. As a consequence, many authors thought that oceanic metabasalts were restricted to normal or transcurrent fault scarps associated with slow spreading ridge tectonics. On the other hand, the relatively smooth topography of fast spreading ridges prevents the deep-seated metamorphic rocks from outcropping. Presumably, metamorphosed crustal rocks are present beneath all of the ridges but the contrasted tectonically induced topographies of the two types of ridge easily explain the dissimilar abundances of the metamorphic rock occurrences. However other parameters such as the crustal permeability related to the primary morphology of the lavas (e.g., pillow vs. lobate vs. massive vs. sheet flows [107]), or to posteruptive ridge tectonics, probably play an important role in controlling the extent of hydrothermal fluid circulation, hence of the associated alteration. Therefore it is suggested that ocean-floor metamorphism has taken and is still taking place today at some depth beneath the seafloor, particularly where new crust is created by seafloor spreading and the temperature gradient is greater than the average gradient measured elsewhere. As a consequence it is thought that metamorphic rocks represent an important and probably a major component of the oceanic crust.
6 How do oceanic ridge hydrothermal systems work?
Before ocean-floor hydrothermal vents were directly observed, hydrothermal activity related to oceanic ridge ‘volcanoes’ had already been suggested as responsible for the precipitation of Fe, Mn-rich metalliferous sediments found on the flanks of the East Pacific Rise [28,31,180]. Lister [111] had argued for the presence of hydrothermal circulation in the oceanic crust on the basis of heat flow measurements transects across active ridges. For instance, across the Explorer Ridge spreading zone, in the NE Pacific, heat flow minima of 1.3–1.9 HFU are localized at the axis of the ridge valley, whereas the adjacent ridge flanks present maxima of 6.6–8.4 HFU, which progressively decrease to the normal lower values farther away form the spreading centre. Lister [112–114,116] attempted to demonstrate that hydrothermal circulation of fluids with temperatures in the range of the transition from greenschist to amphibolite facies, i.e., from 200 to 400 °C, within the oceanic crust is theoretically possible from the mechanics of propagating thermal shrinkage cracks in cooling igneous bodies near magma chambers at the ridge axis. Lister already recognized that crustal permeability is the key parameter controlling oceanic ridge hydrothermal circulation (see Section 6.2 below).
Submarine hydrothermal activity was directly observed at oceanic ridges only in the late 1970s [45,64,182], but hydrothermal water/rock interactions had already been suggested as the mechanism forming the metabasites and disseminated sulfide mineralizations recovered from oceanic ridges (see paragraph 1.1 and references in [30]). Early models had been proposed (e.g., [27,44]), according to which seawater percolating through ridge basalts along thermal contraction cracks reacted with the still hot rocks and mobilized by dissolution as chloride complexes of elements such as Mn, Fe, Co and the rare earths, etc. Corliss [44] concluded that “these solutions may be the metal-bearing hydrothermal exhalations” (responsible for) “the pelagic (i.e., metalliferous) sediments”, and that his “model is similar to one often proposed for the origin of hydrothermal ore solutions on the continents.” Whereas only Mn, Fe oxihydroxides precipitated onto the seafloor upon discharge of the hydrothermal solutions, transition metal sulphides were thought to form at depth within the crust, in altered host rocks containing secondary mineral assemblages similar to the greenschist facies parageneses [30].
The temperature of the hydrothermal solutions were said to decrease by conductive cooling and mixing with cold seawater along the discharge pathway towards the surface. However, the penetration depth into the crust, the geometry of hydrothermal circulation cells, the actual composition and temperature of the hydrothermal solutions, and length of their lifetime were totally unknown. The reason for the relatively late discovery of hydrothermal hot springs, ‘black smokers’ and sulphide precipitates is related to the strategies and tools used to find them. The first direct observations and samplings of hydrothermal activity and its products were carried out using submersibles on the Galapagos Spreading Centre and the East Pacific Rise, at sites selected on the basis of prior detailed heat flow and bathymetric surveys. The studies of active hydrothermal fields have brought a ten-fold increase in our understanding of submarine hydrothermal systems and stimulated the study in the laboratory of hydrothermal water–rock interactions between seawater and MORB in an attempt to duplicate natural oceanic hydrothermal systems. These experiments were carried out at temperatures ranging from 25° to 425 °C, and pressures up to 500 bar [19,21,25,97,127,141–144,159,170–173,175,177,178]. These experiments demonstrated that the black smoker fluids result from MORB-seawater interactions in the conditions of the greenschist facies (i.e., temperatures ranging from 250 to 400 °C, and at pressures corresponding depths of 1.5–2 km beneath to the oceanic ridges). They duplicated the secondary mineral assemblages identified in the natural samples of hydrothermally altered rocks collected form the seafloor. They also emphasized the role of the water–rock ratio during the interactions (e.g., [143]). Finally elemental fluxes and budgets were inferred from the laboratory experiments, e.g., the trapping of 50% of the Mg++ river input to the ocean [57] was explained by the crystallization, in the end products, of secondary Mg-silicates such as actinolite and chlorite already observed in the natural samples. Similarly, the Si, alkalis, and transition metal fluxes measured in the black smoker fluids were verified in the laboratory experiments. A very important side effect of these studies, besides several PhD theses (e.g., [70,139,169]), was the design of new laboratory equipments to carry out the hydrothermal experiments (e.g., [176]). Moreover, the thermodynamic behaviour of seawater itself had to be studied [22,25,26] in order to understand the various cases of hydrothermal systems observed on the oceanic ridges: e.g., the roles of adiabatic expansion [20] and phase separation on metal transport [23] in seafloor hydrothermal system, or supercritical two-phase separations [26,49–51] vs. near-critical conditions [23,24,174].
6.1 The causes of oceanic hydrothermal circulation, the motor of the flows of matter and thermal energy, the thermal engine
First the clinical facts: 72% of the Earth' surface is covered by the world ocean corresponding to 1017 kg of seawater moving above and through the oceanic crust which, as already stated, represents the most extensive igneous formation on Earth. The oceanic crust is generated through accretion of 2.94 km2 yr−1 of new crust along the 60 000 km of oceanic ridges which circle the world seafloor [206]. Since the oceanic crust is, in average, about 6-km thick, this means that about 18 km3 of hot tholeiitic magmas are emplaced every year at an initial magmatic temperature of 1200 °C, along the oceanic ridges. Stein and Stein [186] calculated that the heat flow resulting from cooling of the new crust at ridge axis (i.e., a less-than-1-Myr-old crust) is 10 to 15 times larger than the average global heat flow, and that 7.2% of the total heat loss of the Earth is generated at ridge axis by hydrothermal convection. As the new crust moves away form the ridge axis, the proportion between heat flows due to convective and conductive cooling decreases. After a certain time that varies according to spreading rate, the crustal heat loss is essentially carried trough conduction [164,181,208]. Crustal cooling in a purely conductive regime begins at an earlier age in slow-spreading ridges than in fast-spreading ones. Slow- and fast-spreading ridges cool faster and more slowly, respectively, than an intermediate ridge such as the Costa Rica Ridge. The thickness of the sediment cover on the basaltic basement also plays an important role by ‘sealing’ the crust and, hence, interfering with (i.e., slowing) the heat flux. At DSDP site 504, for instance, the crust of the Costa Rica Ridge flank (which was generated 5.9 Myr ago by an oceanic ridge with a 3.25 cm yr−1 rate) is presently reheated, under a 275-m-thick sediment cover, in an essentially conductive regime: heat flow is about 200 mW m−2 and the temperature at the sediment cover/basaltic basement interface is as high as 59 °C [10]. The temperature at the bottom of the 2111-m-deep drill Hole 504B is 193 °C. In contrast, we will see in the next pages (see Section 8), that the 0.1-Myr-old oceanic crust underlying the TAG mound (located on the slow spreading unsedimented Mid-Atlantic Ridge) is actively cooled by hydrothermal circulation. The oceanic crust at the two sites, i.e., the 5.9-Myr-old crust of DSDP Hole 504B and the 0.1 Myr-old crust beneath the active TAG mound, are presently cooled in two different regimes, i.e., a completely conductive regime and essentially convective regime, respectively.
6.2 Oceanic ridge hydrothermal systems
NOTE: All of the submarine hydrothermal systems that will be discussed in the following pages are related to unsedimented oceanic ridges whose axes are located at water depths exceeding critical conditions for both seawater and hydrothermal fluids. Therefore, no cases of phase separations (e.g., vapour vs. brines) due to boiling of hydrothermal fluids will be discussed in this paper even though they may occur when oceanic ridge axes are relatively shallow such as at the Juan de Fuca Ridge (minimum depth of about 1300 m), and the Mid-Atlantic Ridge near the Azores islands (e.g., Lucky Strike field, with a minimum depth of about 1650 m). However, because the average water depth of oceanic ridge axes generally is about 2500±500 m, and the temperature of the ‘black smokers’ ranges from 320 to 404 °C, hydrothermal fluid may locally undergo phase separation. Bischoff and Rosenbauer [23] experimentally phase separated a natural seawater fluid at 392 °C and 251 bar. Von Damm et al. [202] studied hydrothermal fluids exiting from one and the same vent at the axis of the East Pacific Rise at 9°16.8′N, at a depth of 2582 m, corresponding to a pressure of 258 bar. In December 1989, a ‘black smoke’ with a temperature of 388 °C exited directly from the basaltic sea floor, and no sulphide–sulphate edifice was observed. The physical conditions measured at the EPR vent at 9°16.8′N were very similar to those operating during the experiment of Bischoff and Rosenbauer [23]. In April 1991, the ‘black smoke’ was emitted from a 3–5-m-high sulphide chimney and diffused fluids exited from nearby basalts. In March 1994 the vent ‘black smoke’ had cooled to 351 °C, but the sulphide chimney had the same height as three years before, and the diffuse fluid flow had ceased. The 1991, fluid was low in chlorinity and other dissolved species but high in gases compared to seawater and most of the hydrothermal solutions generally analysed. On the other hand, the 1994 fluid was a brine, with 1.5 times the chlorinity of seawater. The 1991 and 1994 fluids form a compositionally conjugate pair to one another. This observation means that the brine had been stored for three years in the oceanic crust. Delaney and Cosens [49] and Delaney et al. [51] discussed the role of boiling in metal deposition during submarine activity of hydrothermal systems located on ridge axes at water depths smaller than those at which the critical pressure of the solutions is reached. In such cases a two-phase fluid appears made up of a liquid enriched in dissolved salts and with a high pH, and a vapour poor in salt and with low pH. Boiling was inferred from salinities two to five times that of seawater measured in fluid inclusions in secondary minerals from oceanic metabasites [51,98,99,199].
Hydrothermal circulation is more complex than the sole, even though spectacular, gushing of ‘black smokers’ and their ‘plumes’ of dissolved elements and particulate matter suspended in hot fluids. The latter only represent the most visible but distal part of hydrothermal convection, which starts from a diffuse recharge of cold seawater at the seafloor surface before reacting at depth with the oceanic crust, and finally exiting as a hydrothermal fluid discharge on the seafloor. The oceanic crust permeability is the key physical factor that controls the mechanisms of water/rock interactions and the evolution of submarine alteration. Crustal permeability is mainly related to the thermal contraction of the basaltic rocks due to cooling. Later tectonic events, e.g., normal faulting of the floor of the ridge valley, will increase further the permeability of the already cold crustal formations. Therefore, the theory of thermal contraction of cooling magmatic rocks is fundamental to explain the production of cracks needed to make the oceanic crust permeable.
In 1974, Lister first proposed the concept of ‘penetrative cracking front’ by which permeability is created in young, hence hot, oceanic basalts by cold seawater percolating down the crust as it is formed at the spreading axis (Fig. 1a). The process of water penetration into cracking rock is rapid, i.e., about 10 m yr−1. According to Lister [112–116], the ‘cracking front’ is a sharp boundary between the cold, permeable region (i.e., the uppermost lavas outcropping at the seafloor) acting as the reservoir, and the hot impermeable region (i.e., the relatively undisturbed hot rocks surrounding the magma chamber) acting as the heat source. Ahead of the ‘cracking front’ is the thin ‘conduction boundary layer’ where the hot rocks cool and, hence, contract, allowing tensions to accumulate which will be needed to cause the cracks to advance further down. Lister [114,115] predicted that, in the upper oceanic crust (i.e., Layer 2), the crack spacing is of the order of 1–2 m, because the conductive boundary layer preceding the ‘cracking front’ is thin when the crack advance is rapid, i.e., several meters per year as it is the case with fast cooling lavas. When cracks penetrate into hot rocks, the heat stored in them is extracted rapidly into the hydrothermal system at a rate that depends on the rate of advance of the cracks, and on the temperature difference between the hot rock and the fluid. Lister [114,115] also concluded that the ‘active’ hydrothermal system must be an intermittent, local phenomenon, because the penetration of cracks into hot rocks is much more rapid than the seafloor spreading rate, i.e., a m yr−1- vs. cm yr−1-scale. Lister [114] proposed a schematic qualitative 2D model of the fluid flow path in an asymmetric hydrothermal system penetrating the oceanic crust, and “a possible scenario for the birth, growth and death of an ‘active’ geothermal system” (Fig. 1b). The model was supported by calculations and a semi-quantitative model published the following year [115]. Lister's model includes an initial stage with a single open ‘active’ hydrothermal circulation to the maximum penetration depth of 2–3 km, during which a rapid, but short-lived, cracking occurs as the crust is still hot, young and close to the spreading axis. During this stage, the base of the convective system grows the fastest because the least stress needs to be built up in the cooling hot rock to permit the cracks to propagate, and the vertical orientation of the boundary thermal gradient induces the highest heat transfer rate. Open ‘active’ systems with direct discharge onto the seafloor are characterized by hot waters (i.e., 200–350 °C) with extremely short residence time (i.e., of the order of a few hours to tens of hours). The lifetime of the whole ‘active’ phase is ‘of the order of hundreds of years, more like 1000 yr’ [115]. The aspect ratio of the open ‘finger-shaped’ single convection cell does not change as long as the system is undisturbed. Any further increase in elongation will cause a fundamental instability in the circulation, and thence a brake-up into two separate, superimposed independent convection cells characterizing the second stage of the model. The lower cell corresponds to a closed convection of hot fluid, i.e., amphibolite facies conditions, whereas the upper one corresponds to a warm fluid convection recharging with cold seawater, and venting lukewarm fluids at the seafloor. The temperature of the lower cell can now become much higher than in the initial open system, and, therefore, the water–rocks interactions become faster than the penetration rate of the cracks. The lower cell is largely isolated from fresh seawater; hence, it remains acid and reduced, and becomes a ‘sulphide still’ [114]. At the intermediate boundary between the two cells, a “massive sulfide deposit” precipitates, which almost completely seals off the permeability of the crust at that level. As a consequence, the two cells will remain effectively separated from one another. Finally the permeability in the hot cell will collapse because of a combination of chemical (i.e., plugging up by alteration minerals) and physical effects (i.e., static fatigue), and the high temperature phase of hydrothermal circulation ends for that particular system. When the penetrative cracks have ceased to advance into hot rocks and the active circulation cell stops, a substantial permeability remains in the zone above the level of rock closure, and continues to be conductively heated from below. The ‘active’ convection then gives places to a long period of a much gentler ‘passive’ circulation that convects the heat conducted up from below into the surface or subsurface permeable layer of the oceanic crust as long as crustal permeability is retained. The water residence time in the ‘passive’ system is of the order of years to hundreds of years but as the temperature drops the water residence time increases. Alteration at progressively lower temperatures (<100 °C) accompanies the slow cooling of the crust as it spreads farther away from the ridge axis. The ‘passive’ circulation may continue in the remaining permeable zone for many millions of years, until crustal permeability is reduced by clogging of the cracks due to precipitation of low temperature secondary minerals. During this latter stage, the role of sediment cover becomes very important because sediments are much less permeable than the basaltic crust. When the sediment cover is continuous and thick enough, it seals off the waters passively circulating in the crust, which is then allowed to be re-heated. It is presently the case of the crust drilled by DSDP Hole 504B (see Section 7). New systems can open up in any residual hot rock near the system that just died because conductive cooling is slow enough not to extend to rocks more than 300 m away from the permeable zone in the about 1000 year life time of the system. Both ‘active’ and ‘passive’ hydrothermal systems occur in the same permeable rocks. The differences between them consist in their rates of water circulation and residence time, and their life times. Moreover fresh basalt is continually exposed to alteration during the ‘active’ stage, whereas it is not the case during the ‘passive’ stage. Fig. 1b illustrates the four successive stages of the evolution of hydrothermal systems in the oceanic ridges after Lister's figures 1 and 2 [114].
The final picture which arises from Lister's last model is remarkably similar to the vertical zonation of the distribution of alteration products observed in the oceanic crust along the 2 km deep DSDP-ODP Hole 504B (see details in [5] and Fig. 3b in Section 7). From top to bottom: (1) a 310-m-thick, surficial layer of halmyrolyzed basaltic lavas altered at low temperature by oxidizing seawater; (2) intermediate 275-m-thick zone of lavas altered in anoxic conditions by lukewarm fluids resulting from the mixing of the down going cold fluid with upwelling warm hydrothermal fluids (“some mixing with hot fluid leaking from below may occur” [114]); (3) finally, the deeper section of doleritic dykes hydrothermally altered in the conditions of the greenschist facies evolving with time to the zeolite facies conditions. In Hole 504B, a stockwork of sulphide mineralisation was found in the uppermost part of this deepest, hydrothermally altered zone [82] which corresponds to Lister's “massive sulphide deposit” at the “intermediate boundary” separating the two cells [114]. The major discrepancies between Lister's model and the alteration zonation observed in Hole 504B consist in the maximum temperatures reached during alteration in the different zones. According to Lister, the upper cell rocks of his ‘region I’ would be altered at 400 °C (against<70 °C in Hole 504B), and those from the lower cell of his “region III” would be altered at “amphibole (sic) facies metamorphism due to extreme high temperatures” (against <400 °C in Hole 504B).
Wilcock and Delaney [205] argued that the diversity among hydrothermal activities and the sulphide edifices generated by black smokers at mid-oceanic ridges is related to the spreading rate of the ridges. The authors speculate that the contrasts between hydrothermal activities are due to major differences between the heat extraction mechanisms by the hydrothermal systems related to the two types of oceanic ridges. With fast spreading ridges such as the East Pacific Rise, are associated isolated groups of scattered spindly chimneys whereas at slow spreading ridges are found thick complexes of chimneys forming sulphide deposits ranging from several 105 to 106 T in size. Along fast-spreading ridges, spreading essentially occurs by magmatic activity of axial steady state magma chambers that can be insulated by a relatively thick conductive boundary layer because heat extraction is limited by low crustal permeability restricting fluid circulation. Moreover, in fast-spreading ridges, hydrothermal systems sometimes go through episodes of violent ventings (i.e., hydrothermal ‘plumes’ or ‘megaplumes’) that are linked to emplacement of magmas as dykes near the seafloor. The dyking is not only the source of major heat flow but also the cause of increased crustal permeability near the axis of fast spreading ridges. Between dyking events, the heat flux is just large enough to support diffusers-type of low temperature hydrothermal output, and isolated high-temperature black smokers. In contrast, tectonic extension plays a major role in slow spreading ridges such as the Mid-Atlantic Ridge, by maintaining high permeability necessary for long lived, vigorous hydrothermal convection. During the cooling of magmatic bodies near slow spreading ridge axis hydrothermal circulation is driven by downward migrating ‘cracking fronts’ (see Lister's 1981–1982 model above). Through this mechanism large volumes of the oceanic crust are rapidly cooled (Wilcock and Delaney [205] claimed that the entire oceanic crust is cooled), large amounts of heat are extracted, hydrothermal activity lasts longer, and large sulphide deposits are formed, e.g., the TAG mound. As the depth of hydrothermal system following the ‘cracking front’ increases, overburden pressure reduces the crustal permeability progressively decreasing the heat flux, and hence slowing the circulation. Finally venting stops until it is reactivated by a new injection of magma at the ridge axis. The regional survey of water column chemistry [12] supports this model when it proposes that the probability of detecting vigorous hydrothermal ‘megaplume’ at any locale above an oceanic ridge axis increases linearly with the ridge spreading rate.
Baker and Hammond [11] mapped the distribution of hydrothermally produced thermal and light attenuation anomalies in the near-bottom seawater column along 525 km (i.e., four ridge segments were surveyed 2 to 5 times per year during five consecutive years) of the axis Juan de Fuca Ridge, in northeastern Pacific. The Juan de Fuca Ridge is a medium spreading rate ridge (3 cm yr−1) whose segments display contrasted morphologies. The magmatic budget hypothesis [118,119] proposes that along axis morphology and bathymetry variations are controlled by variations in the rate of magma relative to the spreading rates. Hence Baker and collaborators' working hypothesis that a similar relationship exists between along axis hydothermal activity and magma budget. Hydrothermal venting presently occurs along about 20% of the ridge axis. This survey is the first quantitative and continuous survey of hydrothermal venting along a multisegment oceanic ridge. However the Hydrofast cruise of the R/V Jean Charcot, in 1986, actually represents the first attempt at continuously mapping geochemical anomalies (i.e., Mn++, Fe++, 3He and CH4) in the seawater column above the black smokers discharged by segments of the East Pacific Rise, between 10 and 13°N [32]. Crane and collaborators similarly searched for hydrothermal fields by continuously measuring temperature anomalies during side-scan sonar deep tow surveys along the Juan de Fuca Ridge and the East Pacific Rise [46,47]. However, these earlier studies were not quantitative nor did they reveal the actual venting distribution along the axis of the ridges. According to Baker and Hammond [11], there is a relationship between the magma budget (as expressed by the inflated along-axis morphology of the various segments) and the discharge of hydrothermal solutions: the highest the magma input (hence the shallowest the bathymetry), the strongest the hydrothermal output. According to the authors this relationship implies that relatively small magma batches are episodically supplied to discontinuous and spatially distributed melt bodies, and that there exists no constant re-supply to a large steady-state magma chamber. Baker and Hammond [11] proposed that the probability of hydrothermal venting occurring at any locale of the Juan de Fuca Ridge is proportional to the spreading rate of its various segments. They scaled this probability from 5 to 50% for the slowest to the fastest spreading segments, respectively.
Oceanic hydrothermal systems, like all subaqueous hydrothermal systems, are composed of the following five complementary components (see Fig. 2).
- (1) A heat source, i.e., in the case of an oceanic ridge, an axial active magmatic chamber or cooling magmatic intrusion. The hydrothermal activity is as ephemeral as the heat source driving the fluid circulation, which is therefore a non-steady-state process (see above). The magmatic budget of the ridge controls the plate spreading-rate, and both frequency and lifetime of the heat source.
- (2) The oceanic crust is a potential enormous fluid reservoir. In the case of oceanic hydrothermal systems, the amount of water available for hydrothermal circulation through the crust is not a limiting factor as it is often the case of many continental hydrothermal systems. The permeability of the oceanic crust decreases from several tens of mD in its upper most 100 m to a few tenths of mD in the deeper doleritic feeder dyke section (e.g., from roughly 700 to 2000 m into basement in DSDP Hole 504B), and even less permeable in the gabbros of the lower crust. The crustal permeability not only controls the geometry and the flow rate of the hydrothermal circulation, but also the extent and the rate of hydrothermal reactions through the water/rock ratio.
- (3) A recharge down-going limb of bottom, cold and oxygenated seawater. Bottom seawater temperature is<2 °C at the average depth of ridge axis, hence the water percolates down the highly permeable upper crust by gravity. During its progressive warming up, as it descends, seawater changes composition during halmyrolysis of surficial basalts: e.g., it loses K, P and U when precipitating authigenic clay minerals (such as smectites and celadonite) and Fe-oxihydroxides, and becomes anoxic when losing oxygen by reacting with the primary sulfides and titanomagnetite, and forming clay minerals and Fe-oxihydroxides. It loses Ca++ and SO=4 by precipitating anhydrite at temperatures>150 °C, but the sulphate will dissolve when the hydrothermal circulation stops as the crust moves away from the heat source. The excess seawater sulphates will be reduced by reacting at higher temperatures with basalts in the ‘reactor zone’ [146]. The open fissures are successively filled with smectites, phillipsite, and Ca-carbonates, in chronological order of deposition.
- (4) A ‘reactor zone’ where recharge seawater-derived fluids warms up to temperatures ranging from 350 to 400 °C as it draws near the heat source and crosses increasing temperature isotherms surrounding the latter. In the ‘reactor’, seawater derived fluid reacts with hot oceanic crustal rocks near the roof of active magma chambers or cooling magmatic intrusions, close to the transition between oceanic Layers 2 and 3 [1,8,145,200]. At such a depth, appear non-equilibrium hydrothermal mineral assemblages similar to parageneses of the greenschist facies of regional metamorphism. The hydrothermal minerals generally form paragenetic sequences that precipitated from solutions whose chemical activites and temperature changed through time. Primary olivine and augite are totally and partly, respectively, replaced by actinolite, chlorite, and minor talc. Plagioclase is either albitized or partly replaced by anorthite-rich secondary plagioclase in the deepest and warmest sections of DSDP Hole 504B [200]. On the other hand, titanomagnetite is generally replaced by titanite and, as a consequence, magnetic properties of the crust are obliterated. Fissures are filled with actinolite, chlorite, quartz, epidote and, locally, Fe, Cu and Zn sulphide mineralisations. Later veins of zeolites (e.g., analcite, laumontite, heulandite), prehnite, and finally, calcite are formed at progressively decreasing temperatures, ranging from 250 to less than 100 °C [4].
- (5) A discharge upwelling limb of hot, reduced and chemically evolved hydrothermal fluids [141]. Hydrothermal fluid rises adiabatically [20] from an initial temperature in the ‘reactor zone’ close to 400 °C to a slightly lower temperature when exiting at the seafloor. To account for the chemistry of hydrothermal fluids venting at high temperature on the seafloor such as the black smokers, reactions at low water/rock ratios are required in the reactor zone [178]. The adiabatic upwelling of hydrothermal fluids is focused by very high permeability zones (e.g., open fractures, interpillow spaces, and brecciated rocks related to faults) that allow the discharge at high velocity flow rates (measured up to several metres per hour) of the black smoker hot hydrothermal fluids. Alternatively, the upwelling can be slowed down along lower permeability zones (e.g., porous formations) where the hydrothermal solutions are conductively cooled by mixing with bottom seawater, giving rise to warm or moderate temperature ‘diffusers’ hot springs [96,155]. Actually the first directly observed hot springs in the Galapagos Spreading Centre are ‘diffusers’ whose temperature ranges up to 17 °C [58]. Zierenberg et al. [213], Edmond et al. [59], Tivey et al. [197], Mills and Elderfield [132], James and Elderfield [96] demonstrated, through fluid chemistry studies, that the ‘diffusers’ of the Sea Cliff hydrothermal field (northern Gorda Ridge), and those of the TAG hydrothermal field (Mid-Atlantic Ridge), respectively, result form mixings between ‘black smoker’ fluid and seawater. The focused discharge of black smoker fluid at high fluid-flow velocity acts as a pomp entraining seawater into the highly permeable pile of hydrothermal products. As a consequence of the mixing massive silicification of the basaltic rocks occurs at temperature ranging from 85 to 247 °C forming a ‘cap rock’ at the surface of the seafloor (see below [213]). Similarly, large amounts of the three main hydrothermal minerals, i.e., pyrite, quartz-opal, and anhydrite, forming the TAG hydrothermal mound precipitate at temperatures<220 °C (see Section 7 [8,83]), whereas most of the Zn precipitates as sphalerite close to the mound-seawater interface [96] but no ‘cap rock’ is formed.
The respective contribution to oceanic ridge heat and fluid flow budgets by diffused (i.e., low temperature) and focused (i.e., high temperature) discharges of hydrothermal solutions is a very important question with respect to the global heat and chemical budgets but it still being debated. Schultz et al. [162] estimated that the heat flow out of the system of the Endeavour segment of the Juan de Fuca Ridge due to the diffuse mode flow may exceed that due to high-temperature venting by a factor 5″. In a more recent, though preliminary, report Johnson et al. [100] estimated that only 25% of the total heat flux of the Main Endeavour Field of the Juan de Fuca Ridge should be due to diffuse vents (see also Pruis and Johnson, in review). However part of the difficulty in comparing such largely different estimates arises from the definition of “diffused flow”. For Schultz and collaborators [162,163], anything that is not a ‘black smoker’ discharging high-temperature solutions is a ‘diffuser’. In contrast, Johnson and collaborators [100,150] call ‘diffuser’ a fluid with a temperature of 100 °C or less. Another reason for the difference is the techniques used in measuring the heat flux: Johnson and collaborators used AST (Acoustic Scintillation Tomography) over large areas of the seafloor and direct measurements of a lot of diffused vents were integrated over even larger areas. Conversely, at the Endeavour Segment of the Juan de Fuca Ridge, Schultz et al. [162] deployed “a noninvasive electromagnetic flow rate sensor and temperature array directly atop of a sulfide mound to record a series of diffusely percolating effluent temperature and fluid velocity.” At the TAG hydrothermal field of the Mid-Atlantic Ridge, Schultz and collaborators [163] used three ‘Medusa’ temperature-monitoring systems that punctually measured heat flow “directly atop” of “extensive” or “vigorous diffuse flow” in close vicinity (i.e., within one to a few metres) of small black smokers.
According to Saccocia and Gillis [160], secondary mineral assemblages in hydrothermal breccias indicate a relationship between fluid chemistry and rock alteration in the up-flow zones of hydrothermal fluids through the upper oceanic crust. Based on a mineralogical and fluid inclusion study of breccia samples from the MARK area (Mid-Atlantic Ridge at 23°40′N lat.) and the Hess Deep (East Pacific Rise–Galapagos Spreading Center triple junction), Saccocia and Gillis [160] proposed a classification in two types of the breccias found in the oceanic crust.
- – ‘Type-1 breccias’ (e.g., from the MARK area), characterized by the Fe-chlorite, quartz, pyrite, and anatase assemblage would be produced by hot, high salinity fluids (i.e., 3.3–10 wt% NaCl), relatively depleted in H2S and enriched in Fe. This type of breccia is consistent with the ‘M-type alteration pipes’ containing Fe-chlorite, quartz, pyrite, and anatase of alteration pipes in the Cyprus Ophiolite complex [152].
- – ‘Type-2 breccias’ (e.g., from both the MARK area and Hess Deep) characterized by the Mg-chlorite, epidote, quartz, pyrite, and titanite assemblage would be produced by hot, low salinity fluids (<0.1–5.6 wt% NaCl), relatively rich in H2S, and enriched in Mg. The second type of breccia is not exactly related to Richard et al.'s [152] ‘P-type alteration pipes’ containing Mg-chlorite, quartz, pyrite, and anatase because the latter also contains illite (K-rich fluids?), but no epidote.
In both cases, the fluids temperature was ranging 160–370 °C according the fluid inclusion study. According to Saccocia and Gillis [160] the observed salinity variations are explained by a supercritical phase separation of seawater-derived hydothermal fluids at temperatures ranging from 450–500 °C to 550–625 °C in the Hess Deep and the Mark area, respectively. Saccocia and Gillis (1995) predicted that Type 1 alteration assemblages should exist in the up-flow zones of relatively Cl-rich and H2S-poor fluids such as that of the TAG hydrothermal system. However this prediction is not confirmed by the study of the core material drilled during ODP Leg 158 into the stockwork beneath and within the TAG mound. Honnorez et al. (see Section 6.2 [83]) observed a lower zone of earlier chloritized alteration zone characterized by a Mg-chlorite+quartz+pyrite assemblage, followed by an upper zone of later alteration zone characterized by a paragonitic phyllosilicate+quartz+pyrite+anatase assemblage without any chlorite. In contrast no paragonitic mica was observed in Saccocia and Gillis' ‘type-1’ breccias. On the other hand, epidote characterizing Soccocia's and Gillis' ‘type-2 breccias’ was not found in the Mg-rich chlorite bearing altered basalts from the lower zone of the TAG mound.
Hydrothermal fields, i.e., including black and white smokers, and ‘diffusers’ are relatively uncommonly detected on the seafloor. The need for tectonic control on large-scale permeability of the crust, such as open fractures or cataclastic zones, restricts the frequency of focused discharge of hydrothermal fluids such as ‘black smokers’. In addition, ‘black smokers’ are short lived with activity probably spanning from a few hundred to a few thousands years [108] (see next section on TAG field). Their hydrothermal precipitates progressively react with bottom seawater (anhydrite dissolves at temperatures<150 °C), whereas the associated biotas die out. Piles of gossans (‘chapeau de fer’) are found where sulphide chimneys and mounds once existed. On the other hand, ‘diffusers’ are not easily detected, because their manifestation is discreet.
The presence of any ‘cap rock’ in the case of oceanic hydrothermal systems related to unsedimented ridges is poorly documented. According to Scott's model [165–167], a cap rock or another geological trap is necessary to generate focused discharge of hydrothermal solutions, e.g., as ‘black smokers’ on the seafloor, and to pond fluids long enough to generate mineralized lenses. Zierenberg et al. [213] describe the formation of a silicified cap-rock through the silicification/precipitation of siliceous crusts covering and cementing large areas of basaltic talus associated with an active hydrothermal field. The Sea Cliff hydrothermal field is located along a rift-bounding normal fault on the northern segment of the Gorda Ridge. The maximum temperature of 247 °C of the active field was measured in chimneys made up of anhydrite, with minor sulphides and amorphous silica but most venting at the Sea Cliff field is diffuse and at much lower temperature. Two samples of strongly altered basaltic hyaloclastite collected from a talus covering a large normal-fault scarp were found to be altered by hydrothermal solutions in a mixing zone between ascending hydrothermal fluid and entrained seawater. During an initial alteration stage both crystalline and glassy basaltic clasts of the hyaloclastites were replaced by a Mg-rich smectite, i.e., stevensite, mixed-layered smectite/chlorite, and talc, at temperature of about 220 °C, as inferred from O isotope analysis. High water/rock ratio prevailed during the Mg metasomatism that lead to the extreme leaching resulting in the removal of even the least soluble elements such as Al and Ti. More advanced alteration promoted silicification of the already altered basaltic clasts. The hyaloclastite cement is mainly amorphous silica and chalcedony, with traces of barite and pyrite. ‘Ghosts’ of dissolved crystals in the cement were ascribed to anhydrite by the authors. Silicification proceeded at temperature ranging from 110 to 85 °C. Rare clasts of ‘massive sulphide’, i.e., mainly pyrite with minor sphalerite and chalcopyrite, are associated with the siliceous crusts. Finally microbial mats partly preserved in cavities near the upper surface of the crusts contain unusual sulfides and sulfosalts such as pearcite (Ag14.7−XCu1.3−XAs2S11), proustite (Ag3AsS3), besides more normal chalcopyrite and galena. It suggests that microbial activity might have precipitated Ag sulphides and sulphosalts in the siliceous crusts.
A silicified ‘cap rock’ has also been suggested in the case of the Lucky Strike hydrothermal field, in the northern Atlantic, near the Azores [61,63]. FeMn oxide crusts play the same role in hydrothermal sites of back arc spreading centers, such as the Lau Basin [62]. The presence of cap rocks in these cases is probably due to the differentiated nature and often highly vesicular texture, hence the high reactional surface of the silica- and volatile-rich lavas altered in the reactor zones of these particular hydrothermal systems.
Nevertheless it was demonstrated by ODP drilling leg 158 into the active hydrothermal TAG mound (see Section 7) that silicification of the basement rocks in the stockwork beneath the mound, and of sulphide breccias within the latter is vastly inadequate for acting as a “cap rock”, even though the TAG mound is the largest known accumulation of hydrothermal products on the present-day seafloor with about 4.5×106 tons of sulphides.
6.3 Hydrothermal circulation models with or without Layer 3?
Initially, models of ocean floor hydrothermal systems generating black smokers generally considered that hydrothermal convective cells are restricted to the upper oceanic crust (i.e., Layer 2) which includes, from to top to bottom: basaltic effusives (i.e., pillow lava, sheet and massive lava flows, and volcaniclastics such as basaltic breccias and hyaloclastites), doleritic dyke complex, and the dyke-gabbro transition (e.g., [60,113,114,116,140,141]). In these models, Layer-3 gabbros are not considered. According to such models, the ‘reactor’ of the hydrothermal water/rock interactions would be located in the dyke-gabbro transition, i.e., at the boundary between oceanic Layers 2 and 3, and crustal permeability is ascribed to voids in the volcaniclastic horizons and lava taluses, interpillow spaces, and thermal contraction cracks in the lava flows and dykes, as well as Lister's ‘cracking front’ in the roof of cooling magma chambers [113,114]. Is there any relationship between black smokers discharged on the seafloor at focused hydrothermal upwelling zones, and alteration of Layer-3 gabbros resulting from seawater derived fluids circulating in the lower crust? Are oceanic ridge hydrothermal systems rooted into Layer-3 gabbros?
Along with common metabasalts variably metamorphosed gabbros were collected from the Mid-Atlantic Ridge, near 30°N [134,136]. The degree of ‘metamorphic change’ in the metagabbros varies from only a little chlorite, actinolite and green hornblende in some samples, to intense chloritization of clinopyroxene and plagioclase in others producing almost monomineralic rocks. In such cases, enrichments in Fe, Al and, to a lesser degree, Mg was accompanied by removal of Si, and almost total losses of Ca and alkalis. Miyashiro et al. [136] tentatively attributed the metasomatism to hydrothermal alteration of oceanic gabbros by seawater-derived solutions circulating through the ridges. These authors speculated that such a water–rock interaction should have “effects on the composition of ocean water”.
Even though they are not as frequently collected as their effusive counterparts oceanic gabbros and metagabbros are most often found in fracture zones related to transform faults associated with, or in axial valley walls of slow-spreading ridges (e.g., [69,81,94,129,192,199]). Oceanic gabbros often exhibit effects of a multistage hydrothermal alteration starting at temperatures as high as 900 °C [74,131], during plastic deformation and in mostly anhydrous conditions, and ending with temperatures lower than 300 °C, with high water/rock ratios and in static conditions.
Gabbroic rocks from the Romanche and Vema fracture zones, in the Equatorial Mid-Atlantic, have been affected by three different types of hydrothermal alteration processes besides a late-magmatic alteration and submarine weathering [192]. The hydrothermal alterations range from a slight, low temperature (i.e., <150 °C) alteration to a complete recrystallization in the conditions of amphibolite facies. The most common secondary mineral parageneses result from water–rock interactions between 300 and 650 °C corresponding to the greenschist to amphibolite facies conditions. Hydrothermal alteration at the higher temperatures is accompanied by ductile deformation (see Section 3 [81]), while alteration at temperature lower than 600 °C follows brittle fracturing of Layer 3 rocks. The degree of alteration depends on crustal permeability that allows fluid circulation. Seawater–gabbros interactions at high temperatures result in 87Sr-enriched and 18O-depleted metagabbros, which imply an 87Sr depletion and a 18O enrichment of the circulating fluids, contributing to seawater chemistry. Sr isotopic data indicate that the water/rock ratios ranges from <1 to 2 during such alterations. These values are similar to those calculated for other oceanic metagabbros (e.g., [123], in [105]). However, when calculating the water/rock ratios, one should not use the Sr isotopic value of unaltered seawater (i.e., close to 0.709), but that of hydrothermal fluids whose Sr isotopic values are much lower, sometimes almost mantellic. Mass balance calculation of chemical fluxes and budgets indicate that the hydrothermal processes are not isochemical. The metagabbros are enriched in Fe, and probably Mg and Cl, whereas Si, Ca and Ti are lost and released to the hydrothermal fluids. Such chemical fluxes inferred from metagabbros from transform faults offsetting the Mid-Atlantic Ridge probably give an upper limit for the global fluxes because slow spreading ridges are thought to be more deeply affected by crustal tectonics than fast spreading ridges.
As a consequence of the frequent recovery of gabbros and metagabbros from transform faults metamorphic transformations of oceanic gabbros have been ascribed to the peculiar tectonic setting in transform domains where the metagabbros were usually found (e.g., [81,192]). However, Mével and Cannat [131] proposed that gabbros formed at slow-spreading ridges also react with seawater-derived fluids in tectonic settings not related to transfom faults. According to these authors oceanic stretching of the gabbros starts right after their emplacement at or close to the spreading axis. Synkinematic ductile deformation generates shear planes at temperatures ranging from 800 ° to 900 °C, i.e., in anhydrous conditions similar to those of the granulite facies [74]. In axial regions of oceanic ridges moderately dipping normal faults and shear zones allow seawater to penetrate deep into the lower oceanic crust [71], near the ridge axis, when plutonic bodies corresponding to magma chambers are still very hot. Therefore as the temperature of the gabbroic bodies decreases, the lithospheric isotherms progressively sink deeper, and continuing shearing associated with synkinematic ductile cracks allow seawater to penetrate and react with the rocks at increasing water/rock ratios and temperatures ranging from 750–800° to 500–400 °C, i.e., similar to the conditions of the amphibolite facies [185,201]. Finally later and farther away from the ridge axis, ductile deformation stops when temperatures drop below 350–400 °C, and seawater locally percolates and reacts with the gabbros in the essentially static conditions of the transition between amphibolite and greenschist facies [29,94,95] along brittle deformation cracks generated by Lister's [112] ‘cracking front’ in the roof of cooling magma chambers (see Section 6.2). However, Lister modified his 1974 model when he proposed [113,114,116] that the initially single cell hydrothermal circulation related to the ‘cracking front’ is followed by a two-superimposed-and-independent-cell system: the upper ‘warm’ cell reaches maximum temperatures of about 400 °C (i.e., the transition between amphibolite and greenschist facies), whereas the temperature of the lower ‘very hot’ cell corresponds to amphibolite facies conditions (i.e., 400–700 °C) (see above). The complete sequence of recrystallizations and deformations is not present anywhere. Mével and Cannat [131] proposed that the amount of ductile shearing and related high temperature alteration in oceanic Layer 3 at slow-spreading ridges during periods of low magma supply and tectonic extension of the lithosphere may well be a function of the spreading rate. Conversely at fast spreading ridges, Mével and Cannat [131] proposed that hydrothermal alteration of Layer-3 gabbros should follow the downward progression of a cracking front at 500 °C. In such a model, crustal permeability is entirely generated by brittle fracturing, and the absence of high-temperature ductile shear zones in fast spreading ridges prevents any water–rock interaction at temperatures higher than 500 °C.
Conversely, Gillis [68] proposed that the incipient stages of hydrothermal alteration of the oceanic crust are similar at axes of fast and slow spreading ridges. However, there are differences in the secondary mineral assemblages of the incipient alteration and their distribution in the Layer 3 gabbros. As the crust cools and migrates away from the spreading axis the evolution of hydrothermal alteration varies significantly with the spreading rate, because alteration is intimately related to the ridge magmatic budget and tectonic activity. Mapping from submersible the fault scarps which bound the Hess Deep, Equatorial East Pacific, allowed Gillis [86] to reconstruct the spatial distribution of hydrothermal alteration of a crustal section generated at the East Pacific Rise, and ranging from surficial basaltic lavas (200–300 m thick), through a sheeted dyke complex (350–750 m thick), and down to a few 100s of m of plutonic rocks representing the upper portion of the Layer 3. The dyke-lava transition occurs over a 500 m thick interval, whereas the dyke-gabbro transition is 200–300 m thick. The total thickness of the reconstructed study crustal section of the Hess Deep ranges from 1800 to 2400 m. The overlying gabbros and subjacent gabbronorites are thought to represent solidified magma lenses and underlying crystal mushes, respectively, of old axial magma chambers. Theoretical models predict that a conductive boundary layer (which governs the depth of the transition from brittle to ductile deformation in the crust) of tens to hundreds of meters in thickness separates the magma chamber from the overlying hydrothermal system [117]. According to Brikowski and Norton [35] the conductive boundary would migrate deeper into the crust once the magma lens solidified in the magma chamber (i.e., within 1 to 4 km of the ridge axis) as the crust moved away from the axial region. Gillis [68] proposed the following sequence of water–rock interactions between the crustal rocks of the Hess Deep and seawater-derived hydrothermal solutions.
Hydrothermal systems initiated at the fast-spreading East Pacific Rise axis where they were driven by magma chambers at about 2 km depth. Hydrothermal alteration started as soon as the crust was emplaced at the ridge axis and, as the crust moved off-axis, it was repeatedly affected by recurrent dyke injections before it could completely cool off. Consequently, the effects of the initial high temperature hydrothermal alteration were overprinted by a later, lateral heterogeneity in the alteration degree. The ‘reactor’ at the base of the hydrothermal system at the ridge axis is thought to have been located in the root zone of the dykes within the upper gabbros representing the top of axial magma chambers at about 2 km depth. This is where the conductive boundary is supposed to have been located during the peak of the hydrothermal activity. Alteration temperature increased across the lava-dyke transition from<50 to 180 °C (i.e., a geothermal gradient of 26 °C/100 m). In the sheeted dyke complex hydrothermal alteration was highly heterogeneous, with temperatures varying laterally from 150–300 °C to 400 to 450 °C (i.e., minor greenschist facies mineral assemblages to incipient alteration in amphibolite facies conditions) in the upper dykes, whereas pervasive replacement in amphibolite facies conditions on a scale of tens of meters occured in the lower dykes. The highest temperature alteration of the dykes, with temperature maxima up to 600–800 °C, is related to repeated dyke injections that generated short-lived hydrothermal circulations. The transition between the sheeted dykes and the gabbros corresponds to an increase in temperature of up to 200 °C over a ca. 200 m thick interval in the crust, i.e., a strong thermal gradient of 100 °C/100 m. Compared to the dykes, the gabbros are more uniformly altered in the amphibolite facies conditions with a slight greenschist facies ‘retrometamorphism’. The thermal boundary migrated down the crust through the gabbros, into the lower gabbronorites as soon as the magma lens solidified within the magma chamber but, by that time, the intensity of the hydrothermal circulation had already decreased. Hence, when the solutions penetrated into the gabbros along widely spaced microfractures the reaction temperatures ranged from 450 to 650 °C, with locally restricted maxima near 800 °C. The underlying gabbronorites representing the lowermost crust sampled along the faults scarps of the Hess Deep are “typically quite fresh with alteration restricted to phases adjacent to microfractures” [68]. Subsequently the lower crust cooled uniformly, but both the sheeted dyke complex and the gabbroic crust experienced little retrogade alteration, and there is no evidence for a later, off-axis hydrothermal circulation. In addition, the lavas were only affected by a minor low temperature alteration. If the study samples are representative, one can conclude that the temperature in the lower crust of the Hess Deep remained above 650 °C between replenishments of the magma chambers or dyking events. It should also be noticed that no sample of plutonic rock from the fault scarps of the Hess Deep has undergone subsolidus, ductile deformation, and that cataclastic deformation is rare.
Study of altered gabbros recovered from drill holes 894 F and G in the Hess Deep, during ODP Leg 147, provided constraints on the role played by seawater/gabbro interactions in the roots of the hydrothermal system at a fast spreading ridge. It was demonstrated [121] that “The vein-controlled (hydrothermal) metamorphism common in the gabbros of site 894 is entirely a brittle phenomenon” because no evidence of plastic deformation present in the slow spreading ridge gabbros is observed. In the Hess Deep metagabbros, generated at the East Pacific Rise, i.e., a fast-spreading ridge, hydrothermal metamorphism appears to be related to fracture formation, fluid circulation along open space fractures, fracture filling, and alteration of adjacent host rocks [121]. Several generations of crosscutting veins are observed. Alteration starts with the amphibolite facies conditions (i.e., 600–750 °C) leading to a microscopic vein network of amphibole (continuously ranging in composition from Al, and/or Ti-rich brown, to blue-green magnesio-hornblende, to Al-poor actinolite and cummingtonite)+secondary plagioclase, clinopyroxene and magnetite. These early veins are responsible for the pervasive (i.e., 10–50%) alteration of host gabbros into an amphibolite facies mineral assemblage with temperatures in the range of 750 to 600 °C. Rare, later macroscopic veins of amphibole crosscut by the microscopic amphibole veins (with the same range in composition as the amphiboles) are filled with mineral assemblages indicating transitional conditions between lower amphibolite and greenschist facies with temperatures ranging from the 600 to 450 °C. The first two generations of veins do not have preferred orientation. Crosscutting both types of amphibole, later veins of chlorite+epidote and/or prehnite (locally with actinolite, titanite or albite), and chlorite+smectite (or chlorite-smectite mixed layer) are abundant and reflect solutions circulating in another set of cracks, at lower temperatures corresponding to the greenschist facies conditions (i.e., 250–450 °C). The veins show strong preferred east-west orientation with moderate to steep dips. Wall rock alteration halos, in average 3 mm thick but up to 10 mm thick, are associated with the later veins. The halos are characterized by the partial replacement of the primary plagioclase by secondary ones (albite?) and epidote, the clinopyroxene by actinolite, and the olivine and orthopyroxene by chlorite, actinolite and magnetite. The veins often exhibit successive multiple openings and fillings. The chlorite+smectite veins formed at <300 °C are the most abundant macroscopic veins (generally<1 mm in thickness). However, up to several mm thick alteration halos sporadically exist in the wall rocks adjacent to these veins, with chlorite and smectite strongly replacing the clinopyroxene, whereas olivine and orthopyroxene are pseudomorphed by clay minerals. The phyllosilicates were not exactly identified but preliminary microprobe analyses indicate that they are probably smectite–chlorite mixed layered. The last veins are rare; they contain an unidentified zeolite or calcite (exceptionally both), and correspond to very low temperature fluids that did not alter the host rocks.
Lecuyer and Reynard [110] propose a 2 stage hydrothermal interaction between the gabbros from ODP hole 894G in the Hess Deep. An early ‘cryptic’ alteration at temperatures>500 °C was limited to minute amphiboles ‘lamellae’ in the gabbro pyroxenes, whereas the primary Ca-plagioclases remained stable. Water/rock ratios calculated on the basis of Sr isotope studies are very low, i.e., 0.1–0.5, and slightly underestimated as compared with the water/rock ratio inferred from oxygen isotopes which ranges from 0.2 to 1 [110]. A second, late stage of alteration generated mineral assemblages representative of the conditions of the amphibolite-greenschist facies transition, mainly characterized by green amphiboles. Significant elemental fluxes were associated with the second alteration stage, contrary to the first one.
Theoretical considerations combined with their observations lead Manning and McCulloch et al. [122,123] to infer a temperature of up to 750 °C for the onset of brittle failure of crustal gabbros by high temperature thermal contraction. Manning et al. [122] assumed that secondary hornblende and plagioclase equilibrated at a mean temperature of 716±8 °C. The authors also inferred that hydrothermal reactions spanned<60 °C. When combined with thermal models of fast spreading ridges this implies that ‘metamorphism’ was rapid, i.e., <6000 yr, and occurred 1–4 km off-axis.
The onset temperature is much higher than that proposed by Lister [112] or Mével and Cannat [131], but is consistent with the inferred brittle-ductile transition of 700–800 °C in the oceanic crust ([17,137,204]; in [121]). The authors also propose that the veins formed under amphibolite facies conditions within about 60 000 yrs, and a few (i.e., from 1 to 3) km from the crust emplacement at the axis of the East Pacific Rise. Both micro- and macroscopic amphibolite veins, and associated amphibolite to transitional facies alterations of the host rocks were separated by a relatively short time interval, and probably formed under roughly the same stress regime. It is suggested that the later east–west chlorite-bearing veins precipitated in newly opened cracks when the crust formed at the north–south oriented East Pacific Rise was rifted by the Cocos-Nazca east–west spreading centre, when the latter propagated westward to form the Hess Deep. This event may have occurred several hundreds of thousands of years after the gabbros formed, and tens of kilometres away from their emplacement locale. Because the early microfractures are the most widely distributed, they were interconnected and formed an early pore network with high interconnectivity. They allowed more extensive hydrothermal alteration of the gabbros at high temperature than the later macroscopic amphibole veins. Fluid fluxes might have been larger and faster, and along the veins but they were much less numerous and, hence, much less interconnected. Therefore, the macroscopic amphibole veins had less significant chemical effect on the crust. Conversely, the altered gabbros associated with the chlorite-bearing veins only represent less than 6% in volume of the rock despite the great abundance. Hence the contribution of this low temperature hydrothermal alteration process to the overall chemical budget of Layer 3 is small.
According to Manning and MacLeod [121], the large ranges of hydrothermal alteration temperatures of the secondary mineral assemblages filling the different generations of variously oriented fractures appear to indicate that, at a fast spreading ridge, microfracturing does not result from a homogeneous isothermal ‘cracking front’ in the roof of a magma chamber as in Lister's model [112,114–116]; rather, they develop heterogeneously in both space and time.
Hart et al. [72] discuss the contrast between volcanic and plutonic regimes of fluid circulations in Layer 2 and Layer 3, respectively, of oceanic crusts generated at slow spreading ridges. Their study is based on the Sr content, Sr and oxygen isotopic compositions of carbonate veins in Layer 3 gabbros from ODP hole 735B near Atlantis II fracture zone (in the SE Indian Ocean), and in Layer 2 basalts from three ODP drill sites in the Ninety East Ridge (in the Indian Ocean) compared to literature data from other oceanic locales, such as DSDP holes 504B, 417 A and D. Carbonate veins in the upper oceanic crust basalts formed at the lowest temperature (i.e., <150 °C) and late, i.e., within 10–15 My after crust formation at ridge axis. They precipitated from solutions containing a basaltic component of very high Ca/Sr, i.e., >30 times that of black smoker fluids. On the other hand, veins in gabbros from ODP hole 735B formed at both relatively high (i.e., >150 °C) and very low (i.e., <10 °C) temperatures. The higher temperature carbonate veins in the gabbros reflect a large basaltic contribution (as indicated by low 87Sr/86Sr) with low Ca/Sr ratio. They probably formed relatively early, i.e., before the unroofing (i.e., 1 My after the crust formed about 11–12 My ago), and uplift (i.e., 2 My after crustal formation) of the Layer 3 gabbros. The present basement surface has thus been directly exposed to cold seawater for the past 9 My. It is confirmed by the very low temperature veins of carbonate in the gabbros which contain a minor basaltic component (low 87Sr/86Sr), and formed after the unroofing of the gabbros. Almost all of the carbonate veins from the oceanic crust have lower Sr content than expected if they had precipitated from pure seawater; this indicates a basaltic contribution in the solutions from which the calcite veins precipitated in both Layers 2 basalts and Layer 3 gabbros. However, the nature of this basaltic component varies systematically with depth in the oceanic crust and, hence with the solution temperature. Low temperature fluids in shallow crust (i.e., both Layer 2 basalts and uplifted Layer 3 gabbros) have a very high Ca/Sr ratio (i.e., >5000), whereas higher temperature solutions in deeper crustal environments (i.e., Layer 3 gabbros) have much lower Ca/Sr (<500). Hot black smoker vent solutions represent only the low Ca/Sr end member of fluids recorded by the carbonate veins, and therefore cannot describe the totality of the seawater-oceanic crust Ca/Sr exchange budget.
6.4 Elemental fluxes: what comes out, what stays behind?
Two main complementary approaches to the study of submarine hydrothermal systems are required: fluid geochemistry can be compared to a photographic snapshot supplying the chemical composition of the fluid discharged at the seafloor at a given time; this information measures ‘what comes out’ and is as transient as the fluid analyzed. On the other hand, altered rocks correspond to time-lapse photographs because they record the successive interactions between the crustal material and the fluid. Petrological studies of ‘what is left behind’ in altered rocks allow the reconstruction of the evolution of the system through time and space. Numerous chemical analyses of hydrothermal fluids have been published (e.g., [202,203]), but in Table 2 are reported only the compositions of hydrothermal ‘black’ and ‘white smoker’ fluids from the TAG mound, and the East Pacific Rise. As compared to bottom seawater, hydrothermal fluids are hot (ranging from 350 up to 400 °C), reduced (H2S-rich), acid (pH ranges from<3 to 4), Mg-poor and depleted in Ca. On the other hand, they are enriched in metals, alkalis (more particularly K and Li), and silica. During discharge at the seafloor, hot hydothermal fluids mix with cold, alkaline, oxygenated seawater, and consequently temperature drops, pH rises, and the fluids become saturated with respect to transition metal sulfides and quartz. These minerals immediately precipitate to form ‘black smoker’ chimneys, and the particulate matter suspended in the ‘black smoker’ plumes. The latter readily settles around the hydrothermal chimneys, whereas methane, Mn++, and 3H% are the main chemical anomalies in the upper most portion of hydrothermal plumes, as compared to seawater. Almost real-time analyses of these chemical tracers have been used by Bougault and his collaborators [32–34] to locate the plumes associated with hydrothermal activity and discover new hydrothermal fields along ridge axes across the major world oceans.
‘White smokers’ are also observed in present-day hydrothermal sites. As their name indicates, the fluids which are responsible for the formation of their white chimneys and suspended particulate matter in the plumes are rich in Ca- or Ba-sulfates, i.e., anhydrite or barite, respectively. Their chemical composition is quite different from that of ‘black smokers’ (e.g., they are Zn-rich and Cu-poor vs. the other way around for the black smoker fluids, see Table 2) as a result of their lower temperature, ranging from 220–300 °C. As a consequence ‘white smokers’ precipitate anhydrite which is insoluble at temperatures>150 °C, along with sphalerite and pyrite, whereas ‘black smokers’ mainly precipitate chalcopyrite or isocubanite, associated with pyrite or pyrrhotite. The difference in temperature and fluid chemistry between the ‘black’ and the ‘white smokers’ reflects the difference in evolution of two types of fluids: ‘black smoker’ fluids adiabatically rise form the deep ‘reactor zone’ to the seafloor [20], whereas ‘white smoker’ fluids result from the mixing of black smoker type of fluids with entrained seawater which lowers their temperature, brings SO42− and oxidizes H2S (see below in Section 8 on TAG hydrothermal mound setting).
As fluids percolate through and react with the oceanic crust, secondary phases replace the basaltic minerals and fill up the primary pore spaces (vesicles, miarolitic voids, and open fractures), hence reducing the formation permeability. At any given time, the alteration of the oceanic crust is a vertically zoned process, controlled by a depth related thermal gradient due to formation permeability contrasts and hence crustal lithostratigraphy. Accordingly, the distribution of secondary precipitates is also zoned with depth. To illustrate the vertical alteration zonation concept with different degrees of alteration intensities let us compare two case histories, i.e., on the one hand, DSDP/ODP Hole 504B, which corresponds to the diffuse and non-pervasive alteration of the upper oceanic Layer 2, complemented by the gabbros of DSDP/ODP Hole 735B representing the deeper Layer 3, and, on the other hand, the stockwork beneath and within the TAG active hydrothermal mound which corresponds to the pervasive and intense hydrothermal alteration.
7 The case of the 2-km-thick section of oceanic crust at DSDP-ODP Hole 504B
DSDP/ODP Hole 504B is presently, and will probably remain for a long time, the only reference section in the modern ocean crust and, in the next section, its alteration history will be compared to that of the basement under the TAG active hydrothermal mound. Let us summarize our observations in DSDP Hole 504B [7].
The record penetration depth of 2111 m into the oceanic basement was reached in DSDP Hole 504B as the result of drilling efforts during DSDP legs 69, 70, and 83, and ODP legs 111, 137 and 148. The oceanic crust of this site was generated 5.9 My ago by the Costa Rica Ridge, an intermediate spreading ridge with a 3.25 cm/y half spreading rate, located in the East Equatorial Pacific (see Fig. 3a). Heat flow measured on the seafloor at the site is about 200 mW/m2 corresponding with the temperature of 59 °C measured at the sediment/basaltic basement interface [10] under a 274.5 m thick cover of pelagic sediment. As a consequence, abnormally precocious cherts and limestones are present in the lowermost portion of the relatively young sediment cover. Under the sediments, the basaltic basement is formed by, from top to bottom (see Fig. 3b): 571.5 m of pillow lavas with few massive flows and intraflow basaltic breccias, grading through a 209 m thick lithologically transitional zone (in which effusive basalts are progressively replaced downward by doleritic dykes), and ending with a 1056 m thick sheeted dyke complex (100% dykes). No gabbro was recovered from Hole 504B. On the other hand, various seismic experiments, down hole loggings, and physical property measurements indicate that the transition between crustal Layers 2 and 3 seismic velocities is located at about 1500 m bsf±200 m, i.e., near the middle of the sheeted dyke complex [53]. Hence, it was demonstrated for the first time, that the Layer 2–Layer 3 boundary in the oceanic crust does not correspond to a lithological transition from basalts and dolerites to gabbros, but to a change in the physical properties of the crust (e.g, seismic wave velocities) probably related to secondary mineral formation due to hydrothermal alteration. In hole 504B the boundary between Layers 2 and 3 is therefore an alteration front.
The alteration history of a given section of the oceanic crust between the time of its emplacement to that of its sampling by drilling or dredging, could be reconstructed by deciphering the superimposed depositional sequences of secondary mineral assemblages in altered crustal rocks. The uppermost 310 m of basaltic basement was affected by halmyrolysis, a low temperature (i.e., <30 °C) oxidizing alteration process by the down-going seawater of a possible diffuse recharge limb [80]. During this alteration, the estimated water/rock ratios were large, i.e., several 10s. Seawater percolated down through the highly permeable lavas of the upper oceanic crust by following open fissures, microfractures, and interpillow spaces. The secondary minerals characteristic of the ‘submarine weathering’ of surficial oceanic basalts are celadonite-glauconite, saponite and Fe-oxihydroxides (the latter two minerals often combine to form a mineraloid called ‘iddingsite’) which replaces most of the primary olivine, whereas basaltic plagioclase and augite are usually unaltered. Primary titanomagnetite was already partly replaced by maghemite during an earlier stage of alteration, occurring probably while and/or briefly after the lavas erupted on the seafloor (see discussion in [106,209–211]). As a consequence of the oxidative alteration, the basalt cores display red halos, a few mm to a few cm in thickness, concentric with exposed surfaces such as open fissures. The latter were filled with the following secondary mineral sequence forming veins: saponite along the walls, then phillipsite, and calcite or aragonite at centre. Minor pyrite and marcasite specks are sometimes aligned in the host rock, just beyond the oxidative front of the red halos. Fresh basaltic glass was found in pillow lava rims down to the lithological transition to the dykes where effusive rocks disappear.
A intermediate alteration zone is found in the lowermost lava section (i.e., about 250 m thick) lying between the overlying zone of halmyrolyzed basalts, and the underlying hyrothermally altered dolerites (see next paragraph). The main secondary minerals in the lavas of the intermediate alteration zone are, sequentially: saponite, pyrite and calcite filling up open fissures and vesicles, and forming the cement of basaltic breccias. Saponite is also found in the basalts replacing olivine and glass. Such an assemblage reflects relatively low temperature (i.e., <60 °C), anoxic conditions during alteration at low water/rock ratios.
The lowermost kilometre of the doleritic dyke complex contains the higher temperature secondary minerals characteristic of reducing hydrothermal alteration at variable but generally low water/rock ratios (i.e., < or = 1) by upwelling hydrothermal fluids [5,82]. The latter pervaded the rock permeability which is much smaller in the dykes than in the surficial basalts altered at lower temperature. Hydrothermal fluids also have to follow open fractures if one wants to explain the high fluid flows measured in black smokers which adiabatically discharge the solutions on the seafloor at rates of several metres per second. The secondary minerals include albite partly replacing primary plagioclase, chlorite totally replacing olivine, actinolite and chlorite partly to totally replacing augite, and titanite totally replacing titanomagnetite. The secondary mineral assemblage corresponds to the conditions of the greenschist facies of regional metamorphism, and oxygen isotope analyses [7] indicate alteration temperatures ranging from 220 to 380 °C. However, primary mineral relicts (more particularly plagioclase and augite) are common, showing that equilibrium was not reached during alteration even though replacement is sometimes almost complete in the lowermost dyke section.
The downhole variation in the secondary phyllosilicate mineralogy (see Fig. 3b) appears to be related to both alteration temperature and altering fluid chemistry. The halmyrolyzed lavas of the upper low temperature alteration zone contain celadonite and saponite, but in the transitional alteration zone is found saponite which progressively becomes, with increasing depth, a saponite-chlorite irregular mixed layer mineral. Finally, in the dyke section, pure chlorite is dominant but minor irregular mixed layer saponite-chlorite is often observed. The regular saponite-chlorite mixed layer corrensite is rare and was observed in only 8 samples from lower half of Hole 504B (Alt, unpublished; [179]).
From the study of Hole 504B, one can conclude that, at any given place and time, two circulation cells with opposite directions generated three superimposed alteration zones in the oceanic crust, i.e., from basement-sediment interface to the deepest known portion of dolerite dyke complex:
- (1) The surficial layer, a few 100s of m thick, of upper crust basalt halmyrolyzed by down-welling cold seawater;
- (2) The deepest section of dolerites hydrothermally altered by upwelling hot hydrothermal solutions;
- (3) An intermediate basalt section 250 m tick where anoxic alteration probably resulting from the mixing of hydrothermal fluids ascending from the ‘reactor zone’, with downwelling seawater derived fluids after they reacted at low temperature with the surficial basalts.
As the crust spreads away form the ridge axis, it cools down and new permeability is created, hence progressively lower temperature secondary mineral assemblages successively precipitate, mainly in veins filling up open cracks [5,7]. The secondary mineral sequences include: saponite, phillipsite and calcite in the halmyrolyzed upper section of the crust; saponite, pyrite and calcite in the transitional alteration zone; and, in the doleritic section, chlorite, actinolite and locally quartz, sulfides or minor epidote, during a first high temperature hydrothermal alteration stage, followed by zeolites, prehnite and calcite during a progressively decreasing temperature alteration stage. The latter is probably still active at the present time, as both the cooling of the crust in a conductive regime, and today's temperature of 193 °C at the bottom of Hole 504B, result from a reheating due to the sealing of the basaltic basement by the sedimentary cover.
8 The TAG hydrothermal field and mound
The geologic setting of the TAG (Trans-Atlantic Geotraverse) hydrothermal field and active mound was reviewed by Humphris et al. [92], and will be only briefly summarized here. The TAG hydrothermal field is located at 26°N on the Mid-Atlantic Ridge (i.e., a slow spreading ridge with a 1.25 cm/y spreading rate), and covers an area of 25 km2 near the base of the east wall of the median valley (see Fig. 4a). Low temperature hydrothermal activity, with Mn-oxihydroxides and nontronite precipitates, was discovered in 1972 [154,168] between 2400 and 3100 m water depth on the east wall of the rift valley. Two inactive, partly oxidized sulfide deposits also occur on the lower east wall NE of the median valley, i.e., the MIR and ALVIN zones in Fig. 1. U/Th dating indicates that the inactive deposits range in age from 5×103 to 14×103 years [108]. The active TAG mound, discovered in 1985 [158], is located at 26°08′N and 44°49′W at a water depth of about 3600 m (Fig. 1). It lies 1.5–2.0 km east of the spreading axis, on a crust that is about 100×103 years old. The mound is circular (see Fig. 4b), roughly 200 m in diameter and 30–50 m in height (see Fig. 2 after Kleinrock et al., [104]), and consists in 4 to 5×106 tons of pyrite-, quartz-, and anhydrite-rich breccias. The mound is composed of two terraces: an upper terrace at ∼3644 m and a lower terrace at ∼3650 m. A complex of several chalcopyrite-rich black smoker chimneys discharging hydrothermal fluids at temperatures of up to 368 °C is located to the NW of the centre of the upper terrace. On the lower terrace, the Kremlin white smoker complex is comprised of several small (1–2 m) anhydrite, pyrite and sphalerite-rich, but chalcopyrite-poor, chimneys that eject 260–300 °C fluids. These fluids are thought to be derived from black smoker-type fluids cooled by mixing with seawater, and conductive cooling within the mound [59,96,132,197]. As a consequence of the mixing (see Section 6.2) pyrite, quartz-opal, and anhydrite precipitate within the mound where large fractions of Cu are lost, whereas most of the Zn precipitates as sphalerite close to the mound-seawater interface [96].
The pyrite+silica+anhydrite breccias are thought to result from the repeated mass wasting of highly unstable black smokers chimneys, and the recurrent hydrofracturing of previous hydrothermal precipitates [90]. Both matrix- and clast-supported breccias are observed. Steep slopes around the upper terrace are the result of mass wasting which has exposed material rich in silica (quartz and amorphous silica)+pyrite+Fe-oxihydroxides, and formed an apron which consists of mixtures of pelagic sediment and hydrothermal detritus. Diffuse flow of low-temperature (up to 50 °C) fluids, i.e., ‘diffusers’, occurs through much of the mound surface and the surrounding apron [133] whereas locally, e.g., on the northwest side of the mound, low heat flow suggests recharge of cold seawater into the mound [16].
Such a large accumulation of hydrothermal precipitates as the TAG mound is the result of the long lasting but intermitting discharge of hydrothermal fluids focused in a restricted locale. This, in turn, requires that upwelling hydrothermal fluids follow the same, or recurrently opened pathways, to the seafloor. The TAG mound is thought to be located at the intersection between axis-parallel faults and transverse or oblique faults [102,104]. In addition, U/Th dating of sulfide samples from the TAG mound suggests that hydrothermal activity has occurred episodically over a period of 40 000 to 50 000 years [108]. Such a long duration of hydrothermal activity at the TAG deposit is explained through recurrent reactivation of the faults through seafloor spreading related tectonic activity. The fracture permeability allowing repeated hydrothermal discharges at the same location over periods of time of tens of thousand years such as TAG, are thought to be maintained by fault propagation and interaction [48]. A near-bottom magnetic survey of the TAG area indicated that a magnetic low lies directly beneath the mound which was interpreted as a cylindrical alteration pipe or stockwork dipping to the south, and marking the shallow hydrothermal up-flow zone [196].
During ODP Leg 158, 17 holes were drilled into the TAG mound down to a maximum sub-bottom depth of 125 m. Petrographic and mineralogical studies [83] of drilled cores clearly show (see Fig. 4b) that alteration of basement under and in the mound resulted in a vertically and laterally zoned stockwork which is approximately 100 m in diameter and extends at least 125 m below the mound surface. Hydrofracturing of the basaltic basement by hydrothermal solutions was (and probably still is) the main mechanism, which brecciated the basaltic basement, creating new pathways for the fluids to rise to the surface. The cores recovered by drilling beneath and within the TAG mound do not appear to have been drilled through massive lavas but through a pile of angular basaltic debris or a talus.
We reconstructed the sequence of alteration processes of crustal rocks beneath and within the mound [83]. The first stage of hydrothermal alteration is the chloritization of the basaltic basement beneath the mound as a result of interaction with Mg-bearing hydrothermal solutions or mixtures of seawater with the ‘zero Mg’ hydrothermal fluids similar to that which is presently discharged by the black smoker complex, near the centre of the mound. Chloritization occurred at temperatures ranging from 250 to 370 °C. In the deepest part of the stockwork underlying the central part of the mound, i.e., only 25 m away from the centre of the upwelling zone of hot fluids, the basalt is almost completely chloritized with minor quartz and pyrite. Cr-spinel is the only unaltered primary mineral. Beneath the mound margins, i.e., less than 70 m away from the hot fluid upwelling zone, the chloritization process is restricted to mm- to cm-thick halos parallel to the exposed surfaces of basalt fragments. The second stage of hydrothermal alteration is the progressive replacement of basalt and chloritized basalt by paragonite+quartz+pyrite. At the end of this stage, all of the primary minerals are replaced. Paragonite forms, via replacement of chlorite in the stockwork host rocks, and as a result of direct precipitation from hydrothermal fluids in interstitial spaces of the quartz+pyrite breccias. As a result of the recurrent silicification and pyritization, basaltic minerals and textures are progressively obliterated until completely replaced by mixtures of ‘dirty’ quartz (i.e., quartz containing submicroscopic to microscopic inclusions of paragonite, anatase, etc.), pyrite and interstitial paragonite. The last stage of hydrothermal mineralization consists of anhydrite precipitating in open spaces in the breccias such as veins and voids.
Among the metabasites recovered in the cores drilled into the TAG Mound during ODP Leg 158, can we distinguish between the products of hydrothermal alterations resulting from distal vs. proximal water/rock interactions, respectively? Are these processes related to one and the same hydrothermal system as we can see it today? Before answering these questions, we will briefly describe the major types of metabasites recovered from the TAG Mound.
- – Stage 1 chloritized metabasalts: Chloritized metabasalt fragments were recovered from several drill sites in the active TAG hydrothermal mound, both from deep holes located beneath the central part of the mound (and hence close the focused discharge of hot hydrothermal fluids), and shallow holes located at the rim of the mound (and hence about 100 m away form the central discharge). The chloritized metabasalts recovered in the lower part of the deepest hole under the central part of the active mound have been totally replaced by a chlorite+quartz+pyrite assemblage, and the Cr-spinel is the only relict primary phase. Chlorite is by far the dominant secondary mineral replacing the groundmass clinopyroxene, the olivine and plagioclase microphenocrysts, and only partly the plagioclase microlites. Chlorite also filled up vesicles, miarolitic voids, and thin open fractures. Quartz mainly replaced the plagioclase microlites. The primary intersertal structure of the parental sparsely phyric basalt is preserved. Veins of chlorite+quartz+pyrite (in sequential order of deposition) ranging from <1 mm to >10 mm in thickness crisscross the metabasalts. On the other hand, chloritized green halos, only <1–2 cm in thickness, were observed parallel to the exposed surfaces of basalt fragments in cores from distal drill holes into the margin of the mound, i.e., less than 70 m away from the hot fluid upwelling zone. Quartz is not present in these halos, and pyrite is almost completely oxidized, giving the halos a brown hue, and indicating that chloritization was followed by oxidative halmyrolysis by cold seawater. The rock from the inner part of these basalt fragments does not contain any hydrothermal minerals, but exhibits evidence of halmyrolysis: olivine microphenocrysts are partly replaced by smectite (saponite?) sometimes associated with Fe-oxihydroxides (iddingsite) whereas plagioclase microlites and the augite rich groundmass appear to be unaltered. Chlorite under the SE mound margin is Mg-rich and formed via the reaction of basalt with heated seawater. In one core basaltic glass shards were totally replaced by the most Mg-rich chlorite known from the ocean floor [83]. On the other hand chlorite under the NW margin of the mound and in the deep, central chloritized stockwork is poorer in Mg and formed from black smoker-like hydrothermal fluids mixed with small amounts of seawater [3,83,193,194].
Hence, during the first alteration stage, similar hydrothermal processes affected the basaltic basement at TAG both close to and away from the main upwelling zone of hydrothermal solutions, but they varied in intensity, and in the chemistry of the secondary products, i.e., chlorite, varied according to the composition of the altering fluids and, hence the distance to the up-flow zone of hydrothermal fluids. Under the central part of the hydrothermal mound the chloritization process lead to the total replacement of the basalt, whereas under the mound margins the chloritization process only slightly affected the outer portion of the basalt fragments. The variable chemistry of chlorite reflects the variable degree of mixing between Mg-poor black smoker fluid and Mg-rich seawater. Both the variable intensity of chloritization and variable composition of chlorite seem to be related to the distance from the focused discharge pathway of the hydrothermal solutions.
- – Stage 2 paragonite+quartz+pyrite replacement of basalts: A detailed X-ray diffraction and transmission, and TEM study [84] indicates that the TAG paragonitic mica is a mixture between a pure paragonite and an ordered irregular mixed-layer paragonite/dioctahedral smectite. This mixed-layer phyllosilicate has never been identified before. Paragonitic micas reflect the particularly high Na/K ratio of the present-day hydrothermal fluids discharged by the TAG black smokers at temperatures of up to 370 °C [37,59,65]. The TAG paragonitic mica often contains Cr and Ti but contamination by microscopic to submicroscopic inclusions of anatase (identified by X-ray diffraction) and Cr-oxides could be responsible for the high Cr and Ti contents measured by electron microprobe. The paragonite+quartz+pyrite assemblage, which characterizes the second stage of hydrothermal alteration, is clearly overprinted onto the already chloritized metabasalts resulting from the first alteration stage, and is restricted to the central part of the mound. Therefore the second hydrothermal alteration stage coincides with the main upwelling of undiluted, high temperature hydrothermal fluids generating the black smokers at the top of the mound. Therefore we can conclude that, at TAG, both chloritization and paragonitization are two successive hydrothermal alteration processes. Chloritization affected a much larger volume of basement than paragonitization. Chlorites seem to result from interactions between basalts and hydrothermal fluids which can be mixed with seawater, at temperatures in the lower part of the 250–370 °C range. On the other hand paragonite formed later at the highest temperatures, and from undiluted black smoker fluids. It is restricted to the central zone of hydrothermal fluid upwelling.
According to Saccocia and Gillis [151] secondary mineral assemblages in hydrothermal breccias indicate a relationship between fluid chemistry and rock alteration in the up-flow zones of hydrothermal fluids through the upper oceanic crust. Based on a mineralogical and fluid inclusion study of breccia samples from the MARK area (Mid-Atlantic Ridge at 23°40′N lat.) and the Hess Deep (East Pacific Rise-Galapagos Spreading Center triple junction) Saccocia and Gillis [151] proposed a classification in two types of the breccias found in the oceanic crust:
- ‘Type 1 breccias’ (e.g., from the MARK area) characterized by the Fe-chlorite, quartz, pyrite, and anatase assemblage would be produced by hot, high salinity fluids (i.e., 3.3–10 wt% NaCl), relatively depleted in H2S and enriched in Fe. This type of breccia is consistent with the ‘M-type alteration pipes’ containing Fe-chlorite, quartz, pyrite, and anatase of alteration pipes in the Cyprus Ophiolite complex [152].
- ‘Type 2 breccias’ (e.g., from both the MARK area and Hess Deep) characterized by the Mg-chlorite, epidote, quartz, pyrite, and titanite assemblage would be produced by hot, low salinity fluids (<0.1–5.6 wt% NaCl), relatively rich in H2S, and enriched in Mg. In both cases, the fluid temperature was ranging 160–270 °C according the fluid inclusion study. The second type of breccia is not exactly related to Richards' et al. [152] “P-type alteration pipes” containing Mg-chlorite, quartz, pyrite, and anatase, because the latter also contains illite (K-rich fluids?), but no epidote. According to Saccocia and Gillis [151], the observed salinity variations are explained by a supercritical phase separation of seawater-derived hydothermal fluids at temperatures ranging from 450–500 °C to 550–625 °C in the Hess Deep and the Mark area, respectively. The authors predicted that Type1 alteration assemblages should exist in the up flow zones of relatively Cl-rich and H2S-poor fluids, such as that of the TAG hydrothermal system. However, this prediction is not confirmed by our study of the core material drilled during ODP Leg 158 into the stockwork beneath and within the TAG mound. Honnorez et al. ([83], see this article, Section 6.2) observed a lower zone of earlier chloritized alteration zone characterized by a Mg-chlorite+quartz+pyrite assemblage, followed by an upper zone of later alteration zone characterized by a paragonitic phyllosilicate+quartz+pyrite+anatase assemblage without any chlorite. In contrast, the paragonitic mica observed by Honnorez et al. [83] is not present in Saccocia's and Gillis's ‘type 1’ breccias, whereas the epidote characterizing Saccocia's and Gillis's ‘type 2 breccia’ was not found in the Mg-rich chlorite bearing altered basalts from the lower zone of the TAG mound.
9 White micas from other oceanic locales and geotectonic settings
Illite and illite/smectite mixed-layer clay minerals appear to be common in land-based volcanogenic massive sulfide deposits and associated stockworks where the phyllosilicates are generally interpreted as the hydrothermal replacement products of rock fragments (e.g., [101,152]). The common occurrence of illite and illite/smectite (I/S) mixed-layer clay minerals in oceanic sediments is interpreted as resulting from diagenesis and/or burial metamorphism, or hydrothermal alteration of smectite which progressively recrystallizes according the classic sequence: smectite → random illite/smectite mixed-layer→regular illite/smectite mixed-layer→illite [183,184]. The proportion of illite and the degree of ordering in the I/S phyllosilicates are thought to increase with temperature.
In contrast occurrences of ‘sericitized’ metabasalts have rarely been described from active and inactive submarine hydrothermal fields in the present-day ocean. The name ‘sericite’ is placed between quotation marks because, in most cases, the mineralogy of the fine-grained, white mica is poorly defined. Na- and or K-rich micas have been identified in stockworks beneath a few volcanogenic massive sulfides deposits on land [198]. On the other hand, several occurrences of ‘sericitized’ metabasalts were found where K-rich white micas are associated with ophiolite-hosted sulfide deposits (e.g, [152,212]). In ancient crust both epidosites and ‘sercitized’ metabasalts appear to be proximally located with respect to hydrothermal fluid up-flow zones (see Section 2).
Paragonitic micas were documented in oceanic basalts of the Izu-Bonin-Mariana fore-arc region, in the western Pacific [8]. The lowermost basement of the 41 My old crust drilled during ODP Leg 125 is made up of an altered boninite-andesite sheeted dyke complex. The rocks are variably recrystallized to chlorite, smectite, chlorite/smectite mixed-layer minerals (including corrensite), quartz, albite, K-feldspar, and rare magnetite. The 5 m thick section of basement, near the bottom of the drill hole is intensely hydrothermally altered and mineralized, but it does not contain smectite or chlorite/smectite mixed-layer minerals. It contains ‘sericitized’ 1–1.5 cm thick halos adjacent to quartz veins where the rock is replaced by “mixtures of paragonite, mixed-layer mica-smectite, and pyrophyllite”, associated with quartz, albite, K-feldspar, and minor chlorite. Microprobe analyses of the mica mixtures indicate dioctahedral aluminous micas containing small amounts of Mg, and subequal amounts of K and Na. It is not certain whether these white mica mixtures consist of submicroscopically intergrown K- and Na-rich phases, or metastable intermediate NaK mica [8]. Pyrite containing small inclusions of pyrrhotite, chalcopyrite and sphalerite is disseminated in both the host chloritized rocks and the sericitized halos.
Metabasalts containing white micas diversely called ‘sericite’, ‘illite’, or ‘muscovite’ have been rarely described from active and inactive submarine hydrothermal fields in the present-day ocean and, in general, they only represent minor or trace constituents of the altered basalts An ‘illitic mineral’ was found to partly replace plagioclase in basalt fragments associated with the Green Seamount suflide deposit, 12 km off the East Pacific Rise [6]. Mg-rich chlorite, beidellite or saponite also participated to the replacement of the basalt with titanite and pyrite, but a single clay mineral was present within any individual basalt fragment. Rectorite, a regular illite/smectite mixed-layer clay mineral (I/S) was identified in the inactive massive sulfide deposit from the Green Sea Mount where it fills pore spaces between quartz and sulfide where it was followed by opal [2]. The I/S phyllosilicate represents on average 3% (maximum 18%) of the modal composition of the quartz+sulfide+phyllosilicate ore samples. It was interpreted as having directly precipitated from hydrothermal solutions. The I/S phyllosilicate with R1-type ordering contains 25–45% smectite. One sample also contained discrete smectite. In addition I/S replaces plagioclase phenocryts in metabasalt fragments (see above [6]). O isotope analyses indicate that the I/S formed at temperatures of 220 or 270 °C assuming formation from 0% seawater or a +2% enriched hydrothermal fluid, respectively. On the same basis, prior quartz and later opal would have formed at temperatures of 230 or 320 °C and 70 or 170 °C, respectively [6]. Green Sea Mount I/S phyllosilicates have similar chemical composition to those related to Kuroko massive sulfide deposits (i.e., they fall on the muscovite-pyrophyllite side of Hower and Mowatt, [87], ternary diagram), but different from those of diagenetic origin in marine sediments (i.e., the latter are more ‘celadonitic’ and fall near the centre of Hower and Mowatt, [87], ternary diagram). Authigenic clay particles with a ‘sericitic’ chemical composition have been identified in the altered host rock of a chloritic vein in metabasalts from DSDP Hole 504B [109]. The white mica was associated with albite partially replacing the primary plagioclase phenocysts, whereas the olivine phenocrysts were replaced by chlorite. In the last two cases ‘sericite’ or ‘illite’ were not identified by X-ray diffraction but on the basis of the chemical compositions obtained by electron microprobe analyses.
Well crystallized boehmite and anatase, and irregular mixed-layer chlorite-Al-rich (most likely a bedeillitic) smectite along with discrete chlorite and smectite were respectively identified from outer and inner 0.2–1 mm thick alteration crusts replacing two basalt samples dredged from the Gorda Rise [86]. In the inner crust minor quartz and phillipsite, possibly lizardite and kaolinite, and traces of talc were also found. The Al-rich secondary minerals are thought to result from the residual Al-enrichment (not the precipitation from Al-rich hydrothermal fluids) during the formation of the crusts. Minor amounts of boehmite were also identified in hydrothermal precipitates and surficial alteration crusts on basalts from the same general area [85], and from 8 km south of this area [42]. Unfortunately, all of the basalt samples studied by these authors were dredged from taluses which crumbled from the east wall of the ridge axial valley. Hence their age is unknown, even though the authors think that they are relatively young because of the freshness of the glassy pillow rims [85]. Mg-rich minerals, i.e., the discrete chlorite and smectite, and a now disappeared mixed-layer chlorite–smectite, are considered to have formed during an initial hydrothermal alteration stage of the basalts in seawater-dominated conditions. Then, during a second stage, the temperature of the fluid rose and leaching by the extremely low pH solutions prevailed. As a result, extensive leaching of all elements except Al and Ti promoted the formation of boehmite and anatase in an outer crust, whereas a beidellitic smectite–chlorite mixed-layer clay mineral replaced the first formed clays in the inner crust. However, the relationship between the study samples and any active hydrothermal vents was not demonstrated. The high temperature conditions of alteration were inferred from the similarity with other cases of Al-rich clay minerals described in the literature as resulting from high temperature hydrothermal alteration. They were mainly compared to the Al-rich phyllosilicates identified in alteration crusts on oceanic basalts collected from the East Pacific Rise [73]. Two samples were collected by submersible from an hydrothermally altered outcrop about 1.5 km away from an active black smoker vent field, near the axis of the East Pacific Rise, at 21°N. One sample was coated with X-ray amorphous material, whereas the other one was covered by a 0.5–0.75 mm thick crust of white phyllosilicate whose surface was stained by Fe-oxide compounds and coated with a scattering of tiny pyrrhotite crystals. Detailed mineralogical study indicated that the mineralogy of the hydrothermal alteration layer changes from impure, partly dehydrated beidellitic smectite adjacent to the basalt to a mixture of beidellitic smectite and randomly interstratified Al-rich chlorite/beidellite (90% smectite, 10% chlorite mixed layer), plus some discrete chlorite and possible minor amesite in the exterior of the crust. The basalt from the interior of the sample, i.e., away from the alteration crusts, appeared to be fresh but its18O value indicates a slight, low-to-moderate temperature. According to Haymon and Kastner [73] the hydrothermal clay minerals formed by complete replacement of the basaltic glass and plagioclase microphenocrysts within 1 mm of the surfaces exposed to the venting of black smokers. The hydrothermal fluids were extremely reducing, acidic, and Mg-poor, and they interacted with the basalt and normal seawater. The Al-rich phyllosilicates formed at temperatures estimated to be in the 290–360 °C range, and may be considered as residual phases remaining after intense basalt leaching.
All of these seafloor occurrences of Al-rich phyllosilicates have been interpreted as being related to black smokers hydrothermal activity, and having formed from relatively high temperature (i.e., in the 220–360 °C range) solutions with a high degree of supersaturation. The Al-phyllosilicates resulted either from the replacement of basalt fragments or direct precipitation with other hydrothermal minerals such as pyrite, quartz and opal. Such cases confirm the mobility of Al and Ti during rock/water interactions in hydrothermal conditions when solutions are very acidic and reducing. However one should keep in mind that such phyllosilicates are probably metastable phases which disappear after the hydrothermal activity which generated them ceases.
Zierenberg et al. [213] describe the formation of a silicified cap-rock through the silicification/precipitation of siliceous crusts covering and cementing large areas of basaltic talus associated with an active hydrothermal field. The Sea Cliff hydrothermal field is located along a rift-bounding normal fault on the northern segment of the Gorda Ridge. The maximum temperature of 247 °C of the active field was measured in chimneys made up of anhydrite, with minor sulfides and amorphous silica but most venting at the Sea Cliff field is diffuse and at much lower temperature.
Two samples of strongly altered basaltic hyaloclastite collected from a talus covering a large normal-fault scarp were found to be altered by hydrothermal solutions in a mixing zone between ascending hydrothermal fluid and entrained seawater. During an initial alteration stage both crystalline and glassy basaltic clasts of the hyaloclastites were replaced by a Mg-rich smectite, i.e., stevensite, mixed-layered smectite/chlorite, and talc, at temperature of about 220 °C as inferred from O isotope analysis. High water/rock ratio prevailed during the Mg metasomatism that lead to the extreme leaching resulting in the removal of even the least soluble elements such as Al and Ti. More advanced alteration promoted silicification of the already altered basaltic clasts. The hyaloclastite cement is mainly amorphous silica and chalcedony, with traces of barite and pyrite. ‘Gosts’ of dissolved crystals in the cement were ascribed to anhydrite by the authors. Silicification proceeded at temperature ranging from 110 to 85 °C. Rare clasts of ‘massive sulfide’, i.e., mainly pyrite with minor sphalerite and chalcopyrite, are associated with the siliceous crusts. Finally microbial mats partly preserved in cavities near the upper surface of the crusts contain unusual sulfides and sulfosalts such as pearcite (Ag14.7−XCu1.3−XAs2S11), proustite (Ag3AsS3), besides more normal chalcopyrite and galena. It suggests that microbial activity might have precipitated Ag sulfides and sulfosalts in the siliceous crusts.
10 Conclusions
In this paper we have proposed that ocean floor metamorphism and hydrothermal alteration are two end members of a wide range of water–rock interactions under hydrothermal conditions, with varying degrees of intensity and under different conditions. They respectively correspond to distal and non-pervasive, vs. proximal and pervasive cases of hydrothermal reactions between oceanic crustal rocks and discharges of seawater-derived solutions.
Two main case histories were chosen to illustrate the two end-members, and they were compared to other published examples of alteration processes generating similarly altered oceanic rocks. On one hand the studies of metabasalts and metadolerites in the more than 2 km long drill Hole 504B of DSDP/ODP, and of metagabbros in ODP Hole 735B represent the reference studies of the non-pervasive and distal hydrothermal alteration (i.e., so-called ‘hydrothermal metamorphism’) through Layer 2 and Layer 3 of the oceanic crust, respectively. Hydrothermal processes diffusively affect Layer 2 rocks of the upper oceanic crust, at temperatures ranging from 400 to 150 °C, or locally transform the gabbros of the deeper oceanic Layer 3 along fissures, at temperatures ranging from 800 to 450 °C. Metabasalts and metagabbros are ubiquitous components of the oceanic crust resulting from such non-pervasive hydrothermal processes. Such metabasites are so commonly and largely widespread on the present-day sea floor that they probably form an important part of the oceanic crust.
On the other hand strongly replaced basalts (e.g., epidosites, sericitized or chloritized basalts), and gabbros (e.g., rodingites), are scarcely found in the oceanic crust. The hydrothermal alteration process which pervasively and intensively affects the crustal rocks at temperatures ranging from 250 up to 370 °C, is located in close vicinity of hydrothermal fluid up-flow zones. Therefore hydrothermally replaced basalts are probably not significant crustal constituents because they are too restricted in space to the immediate vicinity of focused discharges of hydrothermal waters. The horizontally and vertically zoned distribution of hydrothermal alterations within and beneath the TAG active hydrothermal field and mound, near the axis of the Mid-Atlantic Ridge, illustrates a typical example where the basaltic stockwork beneath a 4.5 Mton sulfide deposit has been totally hydrothermally replaced by quartz+pyrite+paragonite assemblages within 25 m of the up-flow zone of the active black smokers, and strongly chloritized deeper and farther away form the upflow zone. Analogous alteration zonations are well known around land-based VMS (Volcanic-hosted Massive Sulfide deposits) that are mined all over the world as Cu, Zn, Au and/or Pb ores.
In summary major differences exist between the metabasalts or metagabbros affected by the weaker and widespread ‘ocean-floor metamorphism’, and the intensely replaced and spatially restricted rocks collected near active or fossil upwelling zones of hydrothermal discharges, but all result from various degrees of intensity of hydrothermal interactions between oceanic crustal rocks and seawater derived hydrothermal solutions.
Even though the secondary mineral assemblages of hydrothermally altered oceanic rocks are very similar to their regional metamorphic counterparts, Eskola's concept of metamorphic facies cannot be applied to hydrothermally altered oceanic rocks because they are almost always the products of transient open systems whose temperature, solution chemistry and flow (hence the water/rock ratio) keep rapidly evolving through time. Metastable assemblages corresponding to two or more successive generations of secondary minerals can often be observed within the same hydrothermally altered rocks, and they generally coexist with primary basaltic relicts.
The degree of high temperature alteration of crustal rocks by hydrothermal fluids, and the amount of ductile deformation may be related to the spreading rate of the oceanic ridge that generates the crust. At fast spreading ridges, the absence of high temperature ductile shear zones prevents any water/rock interactions at temperatures higher than that of Lister's ‘cracking front’, i.e., 500 °C, generating permeability through brittle fracturing. In contrast ductile synkinematic deformation in slow spreading ridges allows water/rock interactions at temperatures up to 800 °C in the gabbros forming the deeper Layer 3 of the oceanic crust.
Oceanic metabasalts exceptionally exhibit deformation and schistosity, indicating that static conditions generally prevailed during the hydrothermal process. It also confirms that hydrothermal alteration is dominated by circulation of fluids along primary permeability pathways such as thermal contraction cracks, interpillow or inter-clast voids, etc, or as a result of hydrofracturing. On the other hand, oceanic metagabbros can display textures due to synkinematic ductile deformations without metamorphic recrystallization, in anhydrous conditions, at temperatures ranging from 900 to 800 °C. They grade into gneissic amphibolites resulting from recrystallizations in presence of high water partial pressure, at temperatures ranging from 750 to 500 °C. Finally, when lower crustal temperatures are reached (i.e., <300 °C), the gabbros are deformed in brittle conditions, and secondary minerals of the greenschist facies assemblages similar to those of the metabasalts form in open fissures and their host rocks. It appears that layer 2 is mainly cooled by hydrothermal convection which alters the basalts and dolerites at medium to low temperatures, under static conditions. Conversely layer 3 is cooled by conduction and its gabbros are altered, at first, at high temperature, during plastic deformation, and later at medium temperature, under static conditions.
Finally, later fissures opened as the plate moved become the path-ways of seawater dominated solutions from which carbonate-rich veins are precipitated at temperatures <150 °C in the superficial basalts within 10–15 My of the crust formation. In the gabbros recovered in DSDP/ODP Hole 735B, carbonate-rich veins formed at temperatures >150 °C when the crust was unroofed, and then at 10 °C when the crust was uplifted by tectonic movements related to the near Atlantis Transform Fault. The carbonate veins are not properly hydrothermal in nature.
The ‘reactor’ at the base of the hydrothermal system at the ridge axis is thought to be located in the root zone of the dykes within the upper gabbros representing the top of axial magma chambers at about 2 km depth, i.e., in the transition crustal region between layers 2 and 3. This is where the conductive boundary is supposed to have prevailed during the peak of the hydrothermal activity.
The various cases of hydrothermal alteration of oceanic crustal rocks presented in the paper do not include metabasites from transform faults which can be strongly deformed and recrystallized into mylonites, ultramylonites and phyllonites during recurrent polymetamorphic events related to shearing along transcurrent fracture zones such as the Romanche Transform, in the Equatorial Atlantic. In addition, hydrothermal processes, end-products and systems discussed in the present paper are restricted to oceanic crust generated at or near spreading axes occurring in present-day oceans. Ancient crustal material (i.e., ophiolites) and present-day off-axis processes (e.g., hot spots) were not considered in the review.