1 Introduction
The demand of water for agriculture is constantly increasing, and will continue to do so due to the rise in the world population and to the effects of climate change on rainfall and evaporative demand in many regions. However, agriculture may in the future be less a priority for water use, because of competition with other uses such as human consumption, cooling of industrial facilities or maintaining river flow. At a regional level, two choices seem possible if agricultural water is to be rationed: either to develop cropping systems and genotypes that can cope with less irrigation, or to strongly reduce, even abandon, crop production in the zones of the world where water is scarce, thereby limiting productive agriculture to fertile and wet zones. The latter solution is efficient for ‘escaping’ crop water stress, but with social, economical and ecological consequences that make it unacceptable.
At the plant level, strategies for developing plants able to grow in dry climate scenarios face essentially the same type of choices. They can consist in (i) escaping the water deficit, for example by a short crop cycle which allows plants to finish their cycle before severe water stress sets in, (ii) avoiding the water deficit, for instance by reducing transpiration or increasing water uptake, (iii) maintaining growth under water deficit, (iv) resisting severe deficit through survival mechanisms. The view developed here is that each of these strategies is associated with costs and benefits, and is best suited to a particular type of environmental scenarios. In a perspective of increased tolerance to water deficit, the solution may well be to develop strategies whose benefits are greater than their drawbacks in a particular context, rather than to find a unique and general genetic solution that confers stress tolerance.
2 Is drought tolerance limited by physical constraints?
2.1 A process with no obvious limits: plant survival under water deficit
Several recent papers suggest that manipulation of a few genes can greatly increase the plant ability to survive under water deficit. Briefly, these genes have two types of functions. (i) A first category of genes is involved in the resistance to partial cell dehydration. The effects of these genes is either to alleviate biochemical effects of stress by an accumulation of molecules that stabilise proteins, membranes and the biological structures of partially dehydrated cells [13], or that avoid the accumulation of toxic species due for instance to oxidative stress [30]. A source for such genes lies in desert ‘resurrection plants’ such as Craterostigma plantagineum, which can survive complete desiccation and resume growth after rehydration. (ii) A second category of genes is involved in stress avoidance by reducing transpiration via rapid stomatal closure or limitation of leaf growth. This can be achieved by several means, for instance manipulating the hormonal balance of the plant [18] or transcriptional activators [17]. Note that most of the transformed plants with increased tolerance or resistance have reduced growth even in well-watered conditions. Because they deplete soil water more slowly than wild types, transformed plants stay green longer and die after the wild plants during a sequence of soil drying.
These studies have led to a considerable progress in the understanding of the mechanisms that allow plants to survive severe water stress. This progress will probably continue because the accumulation of cell protections does not face obvious physical or physiological limits. Besides an obvious biological interest, it may have applications for developing plants able to colonise slopes and thus avoid erosion in Mediterranean or semi-arid climates, or to grow in very dry environments (especially for cattle use). However, they may well be of limited interest in most agricultural contexts, because they address the problem of survival rather than that of production. Plant survival is usually not relevant to agriculture, because farmers avoid sub-lethal conditions (accompanied by extremely low yields) by using an ‘escape’ strategy when choosing their cropping system (e.g., crops with a shorter cycle or pasture instead of crops). Until now, no clear demonstration has been made that the transfer into crop species of exotic genes that confer survival capacity can help to maintain yields under water deficit. Rather, there are theoretical reasons to consider that rapid biomass accumulation is linked to high water use (see below), so genetic engineering will never produce plants able to rapidly accumulate biomass in a desert.
2.2 The control of transpiration makes it possible to maintain plant water status under water deficit
Transpiration per unit biomass is several orders of magnitude greater in plants than in animals. For instance, a typical transpiration rate of 1 m2 of plant canopy in summer in southern France is 6 l d−1 m−2. This volume of water taken up by roots every day, which flows in the xylem and is transpired by leaves via the stomata, is similar to the water volume in a plant. When the water availability to roots decreases, plants tend to decrease transpiration by two means. A short-term effect consists in closing the stomata, thereby reducing the water flux through the plant. A longer-term effect consists in reducing leaf growth, which results in a smaller transpiring leaf area. These two mechanisms of transpiration reduction are adaptive processes that conserve water for later stages of plant development, but they also contribute to a partial homeostasis of the water status of shoot tissues.
When stomata partially close, thereby decreasing transpiration, the leaf water potential (the physical variable that represents the degree of tissue hydration) increases, i.e. leaves become more hydrated. This mechanism allows leaves to maintain their water status in a narrow range. Roots have a signalling role in this process via the circulation of hydraulic and chemical messages to leaves when they begin to sense water depletion [34]. This is exemplified in Fig. 1, which represents a sequence of soil drying and its consequences on a field-grown maize canopy [36]. As the non-irrigated soil dried, stomatal conductance (a physical variable whose variations represent the degree of stomatal aperture) decreased and the concentration of a stress hormone (abscisic acid, which controls stomatal conductance) increased, but the water status of leaves (day-time water potential) remained essentially the same in well-watered and drought-affected plants. These results changed our views concerning the effect of water deficit on growth and transpiration: the cells of drought-affected plants experienced the same level of ‘stress’ as those of well-watered plants in terms of their water potential.
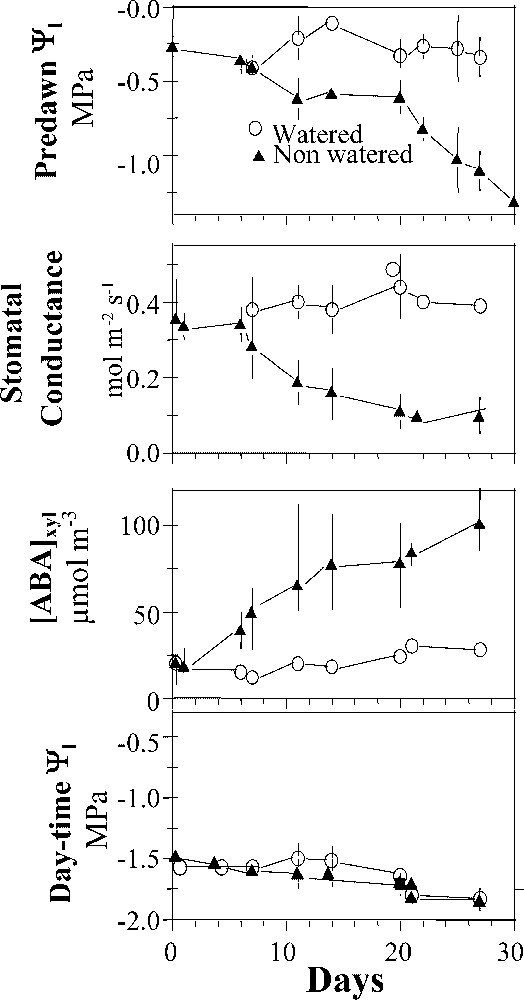
Maintenance of leaf water status of maize during a drying scenario in the field. Non-watered plants were subjected to an increasingly dry soil (a, pre-dawn leaf water potential closely linked to soil water status). Stomatal conductance of drought-affected plants decreased (b) and the concentration of abscisic acid in the xylem sap increased (c, [ABA]xyl). However, the daytime leaf water status remained essentially stable and did not differ significantly between well-watered and drought-affected plants (d, water potential of leaves). Redrawn from Tardieu et al. [34].
Maintien de l'état hydrique foliaire du maïs pendant un scénario de dessèchement du sol au champ. Les plantes non irriguées ont été soumises à un sol de plus en plus sec (a, Potentiel hydrique foliaire mesuré à l'aube, étroitement relié à l'état hydrique du sol). La conductance stomatique des plantes non irriguées a diminué (b) et la concentration de la sève xylémienne en acide abscissique a augmenté (c, [ABA]xyl). Cependant, l'état hydrique foliaire diurne évalué par son potentiel hydrique est resté approximativement stable et ne différait pas significativement entre les plantes irriguées et non irriguées (d, potentiel hydrique). Réélaboré d'après de Tardieu et al. [34].
The above observations, among others, resulted in a multi-species model involving water and hormone fluxes in the xylem, the control of stomatal conductance and the changes in water potential of different organs of the plant [34,35]. The model was recently tested in transgenic plants [4] and extended to predict CO2 fluxes [11]. Briefly, any change in transpiration rate is accompanied by a change in the gradient of water potential between roots and leaves with a mechanism similar to the Ohm's law. In this way, fine-tuning of stomatal conductance via root messages, in particular abscisic acid, allows plants to keep a partial homeostasy of leaf water status. In most circumstances of water deficits compatible with agriculture, the leaf cells therefore avoid severe dehydration via a reduction in transpiration. Conversely, there is a constitutive link between high transpiration rate (a ‘favourable’ trait linked to photosynthesis) and low leaf water potential (an ‘unfavourable’ trait associated with tissue dehydration).
2.3 The ‘water for carbon’ and ‘water for temperature’ trade-offs
Stomatal aperture and leaf area, which are the main determinants of plant transpiration, are also the main determinants of carbon accumulation by plants because CO2 flows to photosynthetic sites via the stomata. This leads to well-documented relationships between light interception, transpiration, photosynthesis, and biomass production [21]. There is therefore a ‘built-in’ contradiction between stress avoidance via a reduction of transpiration and biomass accumulation. The latter can be temporarily buffered by carbon storage in the stem [2] or by changes in carbon metabolism [20], but fast biomass accumulation depends in the longer term on leaf area and stomatal conductance.
A second contradiction exists between avoidance of water stress and avoidance of heat stress. During a sunny summer day, the major part of the incident solar energy is dissipated via transpiration, so non-transpiring leaves are warmer than transpiring leaves by up to 10 °C [19]. A reduction in transpiration rate via stomatal closure can therefore be accompanied by heat stress, and its consequent effects on development time and growth.
2.4 Physical limits of drought tolerance and contradictions between ‘favourable’ traits
Overall, the above suggests that manipulating genes involved in cell protection, which increases the survival of severely stressed plants, may have less influence than frequently believed. This is because of a partial homeostasy of leaf water status under water deficit linked to a fine-tuned reduction in transpiration via the controls of stomatal aperture and leaf growth. These reductions in transpiration, which have a positive effect on plant water status, have two negative effects, namely a reduction in photosynthesis and a higher risk of heat stress. This suggests that tolerance to water deficit might be regarded as an optimising process limited by several physical constraints and trade-offs, rather than as a classical resistance process driven by a series of genes.
3 Possible avenues for progress
If tolerance to water deficit is considered as the ability of a genotype to produce an acceptable yield under water deficit, it is useful to place traits of interest in a framework of analysis that splits the yield into several determinants, and then to consider the genetic progress on individual terms [1,26]. In the literature, two equations have been used for that, which express the processes by which crops achieve their yield in a drought-prone environment. The first one considers the water used by the crop as the key process of yield formation [22]
(1) |
(2) |
3.1 Escaping water deficit through manipulation of the plant phenology (terms ET in Eq. (1) and PPFD in Eq. (2))
An efficient way to obtain plants that can cope with water deficit is to adapt their cycle to expected climate conditions. In particular, reducing the duration of the crop cycle reduces the total soil water depletion, so that crucial stages such as flowering or grain filling occur in a soil with higher soil moisture, resulting in an acceptable yield. The drawback of this strategy is that the total amount of light intercepted by plants is reduced, and so is the potential accumulation of biomass (Eq. (2)). By using a genotype with a shorter cycle, the farmer therefore obtains an acceptable biomass and yield in exchange for a limitation of the maximum yield that can be expected.
3.2 Maintenance of transpiration via a more efficient root system (term ET in Eq. (1))
A way to maintain transpiration and photosynthesis without negative effects on the plant water status is to increase the ability of the root system to take up water. This can be achieved either by improving the soil tillage [32], or by selecting genotypes with increased root growth or branching [39]. Spectacular results have been achieved in this way [38], but it should be mentioned that they apply essentially to cases in which there is a soil water reserve that is not exploited by roots (e.g., water table or deep soil). In cases where plants grow on a limited amount of water (e.g., shallow soil), improving the root system ability to take up water is of little interest or even counter-productive [26]. This may explain a counter-intuitive result in which the selection of maize lines with the highest yields under drought conditions resulted in a reduction of the carbon investment in the roots [3].
3.3 Water-use efficiency and carbon isotope discrimination (term WUE in Eq. (1))
Although biomass accumulation and transpiration are intrinsically linked, one might expect that their ratio can be manipulated to improve the amount of CO2 captured by the plant per transpired unit of H2O (intrinsic water-use efficiency). Farquhar et al. [12] showed that there is a striking similarity between, on the one hand, the equations of water and carbon transfer in the leaf and, on the other hand, the laws of discrimination against the heavy isotope of carbon, 13C, in relation to 12C during the process of photosynthesis. This results in a strong relationship between carbon isotope discrimination (termed ) and the intrinsic water use efficiency. This (negative) relationship has been observed experimentally in a large number of species, with the notable exception of plants such as maize, sorghum, or sugarcane, which have a special photosynthesis metabolism. Because can easily be measured with a mass spectrometer, agronomists and breeders have an efficient way to indirectly measure water use efficiency and carry out breeding programmes to improve it.
Selecting for increased water-use efficiency alone often results in decreased biomass accumulation and yield, with a detrimental effect that reaches a maximum under well-watered conditions [8]. This is part of the ‘water for carbon trade-off’ theory presented above: water-use efficiency increases when the stomata tend to close, reducing both transpiration and photosynthesis. Selecting for high water-use efficiency alone therefore results in selecting for slow growth and low yield. However, interesting results were obtained when special care was taken to overcome this contradiction [23]. Fig. 2 shows that, when tested in a series of experiments with varying rainfall, wheat lines selected for high water-use efficiency had an increased yield under very dry conditions, while the effect was negligible under milder water deficits. The most likely explanation was, again, that these lines had reduced transpiration and conserved soil water for the end of the crop cycle. It is noteworthy that both yields and rainfall were very low in these experiments (1 to 3 t ha−1, i.e. one third of the yields classically observed in Europe), although they are typical of Australian production conditions.
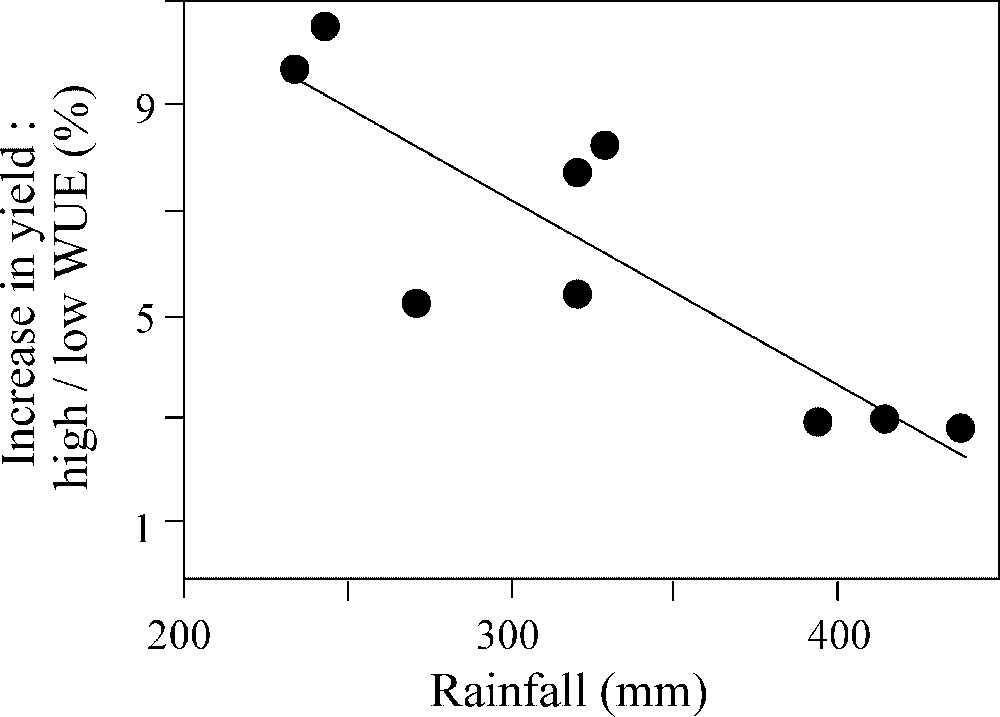
Grain-yield advantage of wheat genotypes selected for high water-use efficiency (WUE) in relation to genotypes with low efficiency, in nine field experiments with varying rainfall. High WUE was advantageous in very dry climates, but had a negligible effect in moderately dry climates. Redrawn from Rebetzke et al. [23].
Augmentation de rendement chez des génotypes de blé sélectionnés pour une efficience de l'eau élevée (WUE) par rapport au rendement chez des génotypes ayant une efficience faible, dans neuf expériences au champ avec des différences de pluviométrie. Une efficience de l'eau élevée a été avantageuse dans des climats très secs, mais ont eu un effet négligeable en climats modérément secs. Réélaboré d'après Rebetzke et al. [23].
3.4 Maintaining growth (terms ET in Eq. (1), in Eq. (2) and HI in both equations)
A reduction in leaf expansion rate occurs before any reduction in photosynthesis or in other biochemical processes [5,27]. Growth maintenance can therefore be viewed as a physiological mechanism per se with several components. Cell turgor is a positive pressure in cells (usually ranging from 0.3 to 1 MPa), which is the driving force in tissue expansion. It is at least partially maintained when leaf water potential decreases. Because water cannot be forced into cells in the absence of biological pumps, plants use an indirect mechanism that consists in a build-up of solutes in the cell, which causes water to enter into the cell (osmotic adjustment). In situ measurements of turgor suggest that osmotic adjustment is already very efficient, with near-perfect turgor maintenance under water deficit [31], so there may be little room for genetic improvement of this characteristic. On the other hand, many breeding groups have improved osmotic adjustment with positive effects on yield [40], although this has been questioned recently [28]. Other mechanisms involved in the reduction of growth under water deficit are essentially reduced cell division rate [15] and cell wall stiffening, which blocks cell expansion, even with maintained turgor [9]. These mechanisms are themselves controlled by the plant hormonal balance and by the expression of several families of genes.
It can be argued that increasing growth under water deficit makes plants more susceptible to water deficit because of increased transpiration and soil water depletion. This is certainly true in very dry climates such as those presented in Fig. 2. However, several lines of reasoning suggest that this is not the case in moderately dry climates.
- • If growth response to water deficit is a physiological process per se, it is expected that the growth of several organs be affected by the same genetic variability, including that of the reproductive organs that are harvested. A ‘success story’ of plant breeding for tolerance to water deficit has consisted in selecting maize plants with a rapid initial growth of the reproductive organs under drought, indirectly observed by the time elapsed between male and female flowerings [3]. Selecting for improved silk growth under stress resulted in increased maize yields in moderately dry conditions [6], via an increase of the term HI in Eqs. (1) and (2).
- • The water-saving strategy consisting in a reduction of the leaf area is not as efficient as was expected in moderately dry climates, because of the direct evaporation from the soil when it remains partly wet [8].
Maintaining growth, either of the leaves to maintain photosynthesis, or of the reproductive organs to increase the assimilate storage in harvested organs, can therefore be an efficient strategy. Because a genetic change in growth rate cannot have lethal consequences (unlike manipulations of hormonal balances or of signal transduction), there is a considerable natural genetic variability of the degree of growth maintenance under water stress for both reproductive organs [7,25] and for leaves [24]. An example is presented in Fig. 3, in which the elongation rate of maize leaves is plotted against two components of water deficit, either in the air or in the soil, in several experiments. Two genotypes are presented, which have consistent differences in response to water deficit over several experiments. The existing genetic variability of growth maintenance under water deficit can therefore be exploited for developing genotypes with reduced sensitivity. However, it should be stressed again that such genotypes are expected to have a reduced tolerance to very severe water deficits because of faster soil water depletion. A farmer using such genotypes would therefore trade a higher potentiality of biomass accumulation under mild water deficits for an increased sensitivity to very severe water deficits.
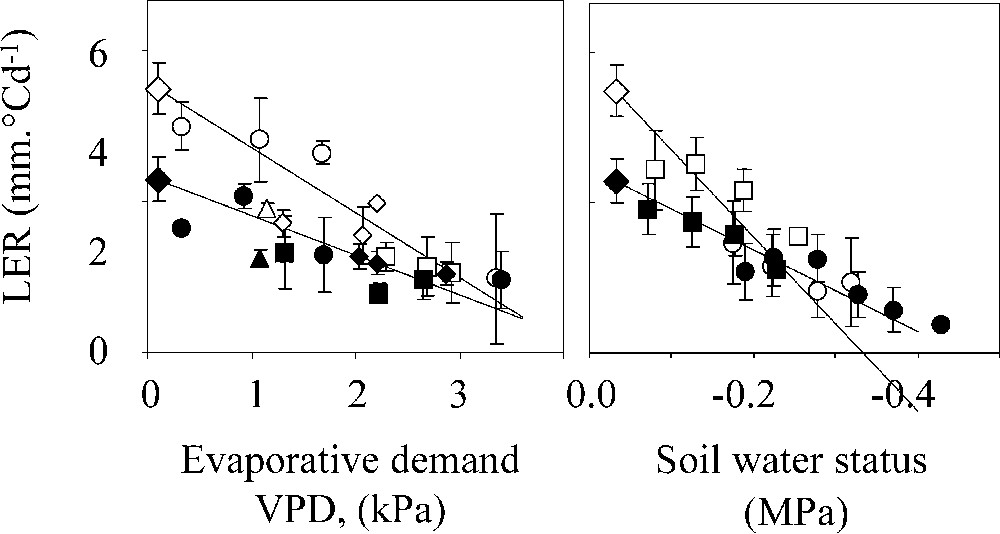
Responses of leaf elongation rate (LER) to evaporative demand and soil water status in two maize genotypes (open and filled symbols). (a) LER per unit thermal time (i.e. corrected for the effect of temperature), plotted against evaporative demand estimated by meristem to air–water vapour pressure difference (VPD) in well-watered plants. (b) LER per unit thermal time during night periods, plotted against pre-dawn leaf water potential (an estimate of soil water status). Each symbol, one coupled value of LER vs VPD. Right panel: experiments in the field (□, ◊) or in the greenhouse (▵, ○). Left panel: experiments in the greenhouse (□, ○, ◊). Redrawn from Reymond et al. [24].
Réponse de la vitesse d'élongation foliaire (LER) à la demande évaporative et au déficit hydrique du sol chez deux génotypes de maïs (symboles pleins et ouverts). (a) LER par unité de temps thermique (corrigé pour les effets de la température) en fonction de la demande évaporative (estimée par la différence de pression partielle de vapeur d'eau entre le méristème et l'air) chez des plantes irriguées. (b) LER par unité de temps thermique pendant les périodes nocturnes, en fonction du potentiel hydrique foliaire mesuré à l'aube (indicateur de l'état hydrique du sol). Chaque symbole représente un couple LER VPD. Panneau de droite, expériences au champ (□, ◊) ou en serre (▵, ○). Panneau de gauche, expériences en serre (□, ○, ◊). Réélaboré à partir de Reymond et al. [24].
4 Which genetic strategies for improving drought tolerance?
4.1 Transfer into crop plants of genes conferring resistance to water stress
Although I have cast doubt on the value of transferring into crops a series of single genes that would enable them to survive the stress, more elaborate strategies of genetic engineering are now feasible. In particular, it is conceivable to transfer favourable alleles of several genes already present in the studied species, or to manipulate the expression of genes under water deficit by manipulating transcription factors. The application of this strategy to the case of water deficit remains to be tested. The main problem will probably be the prediction and the test of the combined effects of the changes in the expression of several genes, within the complex framework described above, characterised by contradictory effects and physical limitations. A possible way of tackling it could be to place the effects of all genes known to be involved in the genetically engineered function into a regulatory network that simulates the effects of fluctuating environmental conditions on their transcript levels, on the amount and the activities of corresponding proteins, and finally on the resulting phenotype. Plant transformation would therefore follow in silico research selecting the most interesting combinations of genes and alleles. This strategy, already used for simulating the behaviour of prokaryotes as a function of their environment [14], has been considered suitable for simulating the effects of water deficit on plants [10].
I have argued elsewhere that this is not feasible yet and may well face theoretical limits that make it impossible in the near future [33]. (i) Our understanding of controls and of involved genes is still poor. Only a small proportion of the genes involved in the response to water deficit is known, and conversely genes with almost any function may have a role in this control. (ii) It is virtually impossible to simulate the interaction between genes in complex systems. A study of only 15 genes with three alleles each would generate 14 million genotypes, an impossibly high number to analyse in enough detail to avoid misinterpretations [33]. (iii) In contrast to existing examples of regulation networks, the phenotype is usually quantitative (i.e. with an infinite number of possibilities) and cannot be interpreted without at least an implicit model. It is therefore proposed that a ‘bottom-up’ strategy consisting in a transfer of genes of known functions is not an appropriate strategy in the search for water-stress tolerance [16,29,36], although it is essential for analysing functions involved in the plant responses to water deficit (e.g., [37]). Strategies based on available genetic diversity would therefore be the most likely to succeed.
4.2 Analysis of the available genetic diversity
In many programmes of crop breeding, germplasm that is better adapted to dry conditions have been developed and released by using conventional high-throughput, long-term selection strategies that emphasise yield alone. There are fewer cases of selecting for drought performance by selecting for specific physiological traits. Two interesting cases are described here above: water-use efficiency [23] and growth maintenance [6]. In both cases, the genetic strategy was based on the search for favourable alleles associated with the traits, in the existing genetic material. Briefly, the most common strategy consists in crossing parents with different phenotypes and genetic backgrounds. In this way, one obtains a large offspring population whose genome is recombined, i.e. in which each chromosome consists of portions of the chromosomes of each parent. An increasingly common approach is to use statistical analysis to associate one allele at a given position of the genome with a phenotypic trait (quantitative trait loci, QTLs). Then, a molecular marker for the trait can be selected directly from seedlings, without having to grow the plants to yield. This labour-intensive technique has the advantage of associating a plant trait of interest with a locus in the genome, even though the underlying cellular-level mechanisms are not known. Its main disadvantage is that this association is statistical, and frequently varies with environmental conditions, i.e. a trait of interest is associated with different parts of the genome in different experiments.
4.3 Towards virtual plants: combining ecophysiological modelling and quantitative genetics
The above strategy can be combined with ecophysiological models that integrate numerous functions and their rapid changes with environmental conditions. Ecophysiological modelling allows prediction of plant behaviour in fluctuating conditions, potentially making it possible to predict the behaviour of a plant in any pedoclimatic conditions. Such models are based on both physical equations, representing fluxes of mass and energy, and control equations that represent the plant response to environmental conditions. The value and weakness of these equations are described elsewhere [33]. Briefly, the fact that the response curves are common to several experiments (Fig. 3) allows one to consider them as ‘meta mechanisms’ at the plant level, although the underlying molecular mechanisms are not known. We have recently proposed such a combination of genetic and ecophysiological models applied to the genetic variability in leaf growth under water deficit [24]. Briefly, a genetic analysis was carried out on the slopes of the response curves shown in Fig. 3, thereby associating alleles with either increased or decreased responses to water deficit. Theoretically, this makes it possible to predict the behaviour of virtual genotypes with any combination of alleles. This possibility was tested by analysing genotypes whose behaviour was either simulated or measured in an experiment with varying environmental conditions (Fig. 4). The model predicted the general trends of the changes in leaf elongation rate with environmental conditions, but also the differences in elongation rates observed between genotypes that were known only by their genetic characteristics.
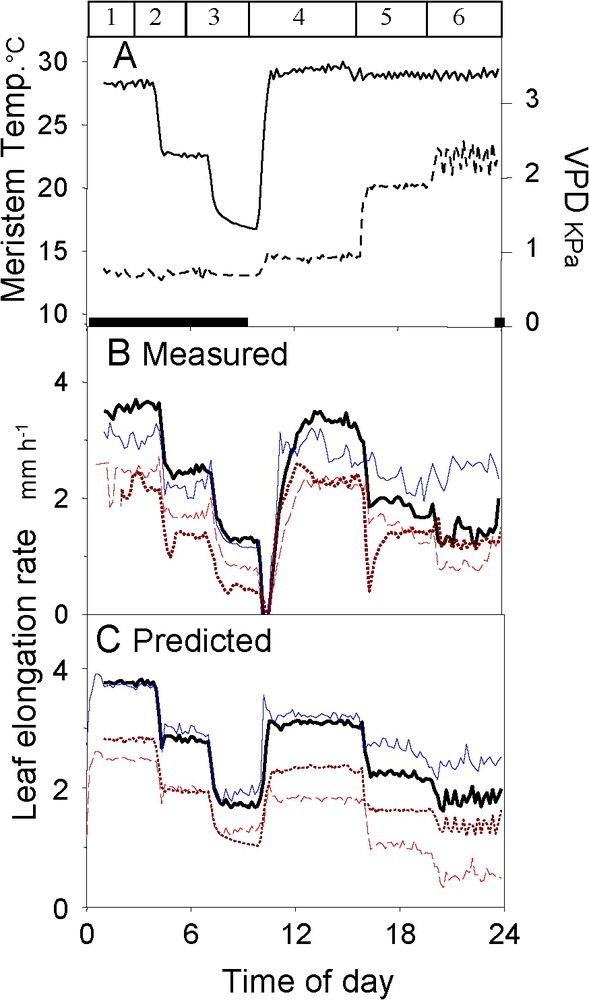
Change with time in measured and modelled leaf elongation rates of maize genotypes in a climatic scenario imposed in the growth chamber. Five genotypes are presented, which were known by their genetic characteristics only. Plants were subjected to a climatic scenario with varying temperature and evaporative demand (panel A). The modelled values were obtained from the ecophysiological model, whose parameters were calculated as a sum of QTL effects in the genetic model. The leaf elongation rates had similar time courses in measured (panel B) and in modelled data (panel C). It decreased in three stages during the night, simultaneously with temperature and, at a constant temperature during the day, it decreased with evaporative demand. Note that the genotype with smallest sensitivity to evaporative demand in simulations behaved in the same way in measured data. Panel A: plain lines, meristem temperature; dotted lines, vapour pressure deficit. Black bars at the bottom of the panel indicate the night periods. Panels B and C: each line style represents a maize genotype. Redrawn from Reymond et al. [24].
Évolution des vitesses d'élongation foliaire mesurée et simulée dans un scénario climatique en chambre de culture. Cinq lignées sont représentées, qui n'étaient connues que par leurs caractéristiques génétiques. Les plantes ont été soumises à un scénario climatique comprenant des variations de température et de demande évaporative (panneau A). Les valeurs simulées ont été obtenues avec le modèle écophysiologique, dont les paramètres ont été calculés comme des sommes d'effets de QTLs dans le modèle génétique. Les vitesses d'élongation foliaire mesurées (panneau B) et simulées (panneau C) ont connu des évolutions similaires. Elles ont diminué en trois étapes pendant la nuit, simultanément avec la température du méristème et, pendant le jour à température constante, elles ont décrû avec la demande évaporative. Noter que la lignée qui était prévue comme la moins sensible à une demande évaporative forte s'est révélée comme telle dans les données mesurées. Panneau A : ligne continue, température du méristème ; ligne pointillée déficit de saturation de l'air en vapeur d'eau. Les barres noires en bas du panneau indiquent les périodes nocturnes. Panneaux B et C : chaque trait représente une lignée de maïs. Réélaboré d'après Reymond et al. [24].
This study, carried out on a simple function, leaf growth, and in a simple genetic population, might be extended to more complex situations and open the way to an in silico prediction of virtual genotypes, thereby allowing a re-engineering of plants. Only the best genetic combinations would then be obtained genetically and tested in the field.
5 Conclusion
The view presented here is that seeking tolerance to water deficit is limited by physical laws and faces several ‘built-in’ contradictions. The link between transpiration and control of water status, and the ‘water for carbon’ and ‘water for temperature’ trade-offs cannot be overridden by simple genetic engineering. This is the reason why genetic manipulations have failed to provide commercially available genotypes that tolerate water deficits, in contrast to what occurs with some other biotic or abiotic stresses. However, progress is possible, as shown by several ‘success stories’ reported here. Those are based on long-term analyses of the existing genetic variability and have brought limited but economically valuable progress. I have stressed in all cases that the trait that has been improved had a favourable effect in a given climate scenario, but may have negative effects in other scenarios. Future progress may well consist in improvements of the plant responses in given drought scenarios, but not in all of them simultaneously. This generates a need for the study of a very large number of combinations genes × site × environmental conditions, which can only be addressed by testing in silico the behaviour of combinations of alleles in each climate scenario.
Acknowledgments
S. Chapman (CSIRO, Australia) critically read the MS of this paper and significantly improved it. I am also thankful for the comments provided by two anonymous reviewers, whose remarks were also very helpful.