1 Introduction
Before the theory of plate tectonics was developed, there was no consistent model for the geology of ocean–continent transitions (OCTs). Not much was known about the nature of the crust of the oceans and only after World War II was the structure of continental margins investigated by modern geophysical methods. The Deep Sea Drilling Project (DSDP) began in the same “annus mirabilis” of 1968 that finally established plate tectonics as a unifying principle in the earth sciences [83]. The striking positive correlation between the age of sediments resting on oceanic basement and distance from the ridge axis in the South Atlantic, established in 1969 during DSDP Leg 3, dramatically confirmed the reality of sea-floor spreading [88] (Fig. 1) and, incidentally, underscored the significance of continental margins in terms of global tectonics.
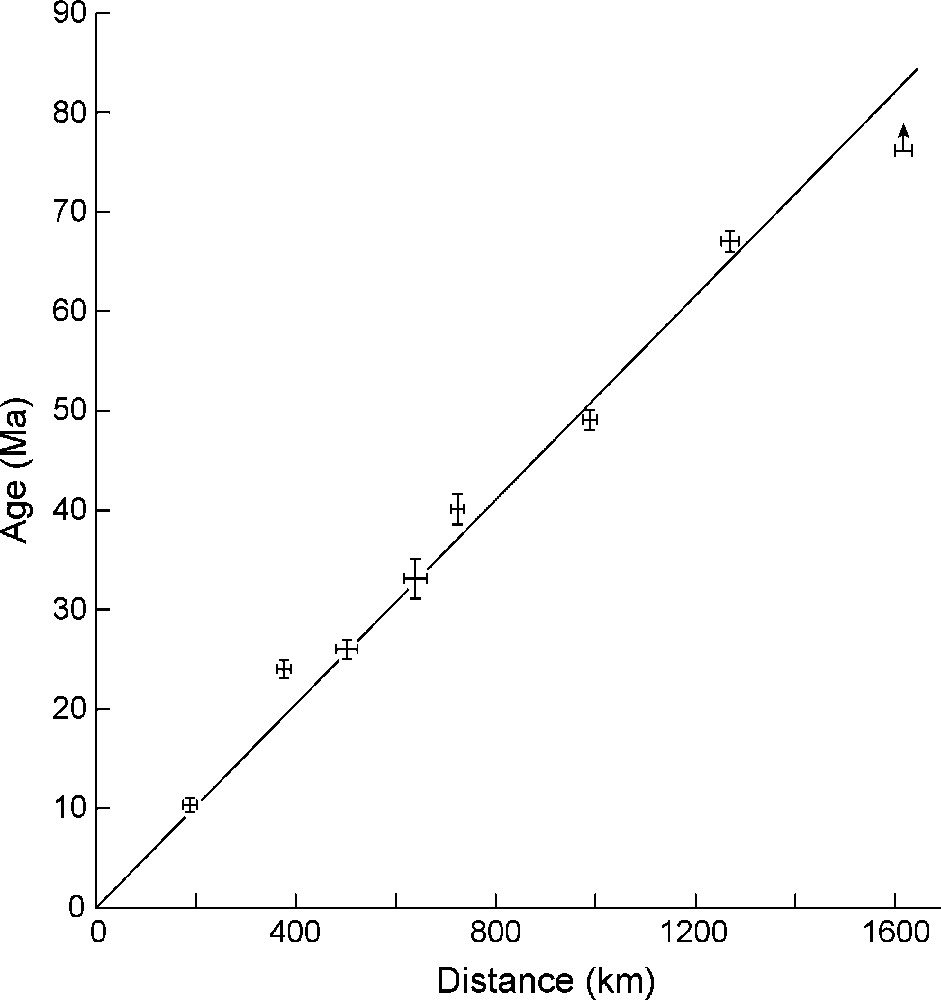
Age of the oldest sediments resting on the oceanic basement of the South Atlantic from Deep Sea Drilling Project (DSDP) cores related to the nearest distance of the drill-site to the ridge axis. From [88, p. 463].
Fig. 1. Âge des plus anciens sédiments reposant sur le socle océanique dans l’océan Atlantique Sud, à partir des carottes des forages Deep Sea Drilling (DSDP), en relation avec la plus petite distance du site de forage à l’axe de la ride. D’après [88, p. 463].
Although the Challenger Expedition (1872–1876, [98]) had dredged basaltic rocks from the deep-sea floor, prior to the introduction of the concept of isostasy as defined by Dutton [51], the fundamental difference between continental and oceanic crust was hardly recognized, a point well illustrated by the “continental flexure” model of Johannes Walther [134] (Fig. 2). During the late 19th and the early 20th century, two strands of thought developed: one that relied on the antiquity and permanence of continents and oceans and another that believed in the mobility of the Earth's crust. Most geologists working on land thought that continents and oceans formed during the early history of the Earth and, as a consequence, “the different composition and thickness of continental and oceanic crust, and the resulting isostastic response would show how improbable it would be to suppose that a continent could founder or go to oceanic depth or that ocean floor at ± 3000 fathoms could ever have been a stable land area since the birth of the oceans” [66, p. 342] (Fig. 3). By contrast, many European workers weighed the evidence of transcontinental faunal exchange in favour of vertical mobility of the Earth's crust to form former land-bridges and shallow seas. In addition, the occurrence on continents of deposits that closely resembled modern deep-sea sediments dredged in all major oceans by the Challenger Expedition [98] cast serious doubt as to the permanence of continents and oceans [119]. Obviously the two strands of reasoning could not be reconciled – at least, as long as oceans were permanent and continents fixed. Wegener's [135,136] theory of continental drift appeared to resolve the paradox, but was not generally accepted because it seemed not to obey the fundamental laws of physics [74].
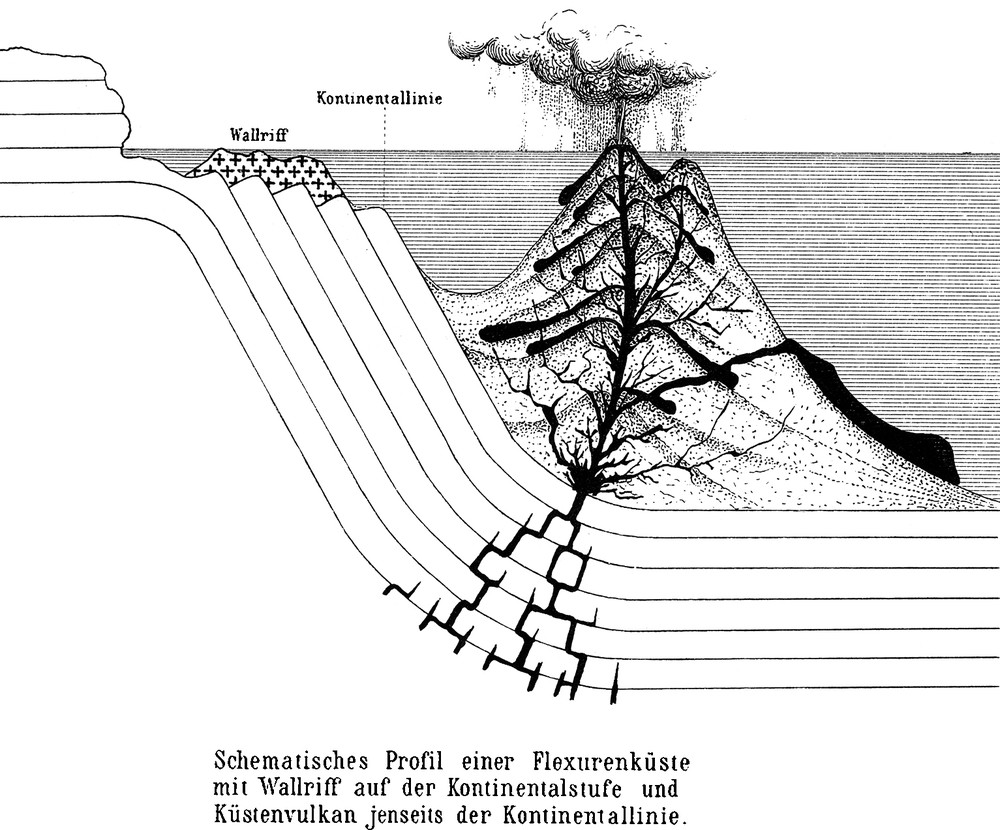
An early concept of an ocean–continent transition, the “continental flexure” of Walther [134, Plate 12]. This diagram illustrating volcanic activity is obviously inspired by a Pacific-type continental margin, but no distinction is made between continental and oceanic rocks.
Fig. 2. Concept précoce d’une transition ocean–continent : la « flexure continentale » de Walther [134 , planche 12]. Ce diagramme illustrant l’activité volcanique est à l’évidence inspiré par une marge continentale de type Pacifique, mais aucune distinction n’est faite entre roches continentales et océaniques.
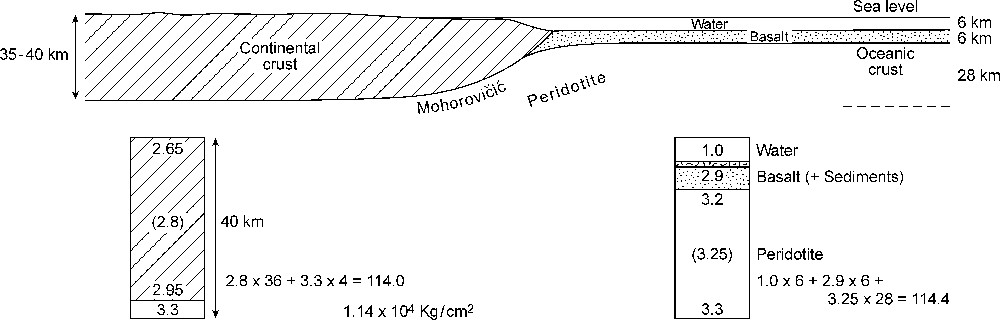
“Diagram to show typical continental and oceanic sectors of the earth's crust”. The numbers refer to densities chosen for the inferred rock-types and indicate that the mass of a column through the continental crust above 40 km depth (from the Mohorovičić Discontinuity to the Earth's surface) is the same as for a corresponding but thinner oceanic column and that hence substantial vertical movement is “improbable”. From [66, Fig. 8].
Fig. 3. Diagramme pour montrer des secteurs continentaux et océaniques typiques de la croûte terrestre : les chiffres renvoient aux densités choisies pour les roches types impliquées et indiquent que la masse d’une colonne de croûte continentale au-dessus de 40 km de profondeur (de la discontinuité de Mohorovičić jusqu’à la surface de la Terre) est la même que pour une colonne océanique correspondante mais moins épaisse et qu’il en résulte qu’un mouvement vertical conséquent est « improbable ». D’après [66, Fig. 8].
Amongst the “odd rocks of mountains belts” [55] that differed so much from the rocks found in and on the stable continents, the rocks of the Steinmann Trinity [10,11], that is, the association of serpentinized peridotites, basalts and radiolarites were going to play a major role in the interpretation of the Alpine geological record. Such rocks had been dredged from the oceans [112]. However, only the results of the DSDP and detailed marine geological research [19–21] allowed the interpretation of certain Alpine lithological associations in the actualistic context of an OCT [15,87]. The following account describes some of the controversies that evolved around this suite of rocks. First, however, the concept of the geosyncline that came to dominate geological thinking during the first half of the 20th century is examined. Table 1 summarizes the most important steps in the historical evolution of the different concepts.
Chronology of research on ophiolites [2,10–12,22–26,28–33,36,38,46–48,58,64,69,81,86,93,99,101,116,120,127–128], geosynclines [5,7,8,18,35,43,45,52,60–62,77,107,115,121–124,137], and mobilist concepts [6,9,16,17,41,42,49,63,68–70,73,83,89,95,108,109,113,117,126,132,135,136,138].
Tableau 1 Chronologie de la recherche sur les ophiolites [2,10-12, 22-26, 28-33, 36, 38, 46-48, 58, 64, 69, 81, 86, 93, 99, 101, 116, 120, 127-128], les géosynclinaux [5,7-8, 18, 35, 43, 45, 52, 60-62, 77, 107, 115, 121-124, 137] et les concepts mobilistes [6,9, 16-17, 41-42, 49, 63, 68-70, 73, 83, 89, 95, 108-109, 113, 117, 126, 132, 135-136, 138].
2 The geosyncline
By the early 19th century, European authors had recognized that sediments in mountain belts were commonly much thicker and/or of different facies with respect to coeval deposits on the stable continents [52]. In North America, Hall [61] genetically connected the thick accumulations of shallow-water sediments of the Appalachian foreland with orogeny and Dana [35] coined the term “geosyncline” for the trough in which these sediments were deposited. We shall not go into the “adventures” this concept underwent, but refer to previous detailed reviews [7,8,44,60,72,110,111]. These depressions, filled by shallow-water [35,61] or deep-water deposits [52,62,125], were considered as zones of crustal weakness from which geanticlines and finally mountain belts would develop, either between more stable continental areas [62,121,124] or between continents and oceans [35,107]. However, the basement of the geosyncline was, with very few exceptions, always thought to comprise continental crust and was hence “ensialic” (rich in Si and Al), not “ensimatic” (rich in Si and Mg) in Wells’ [137] terminology [59,130]. In Haug's [62, Fig. 1] map of the old continents and young mountain belts, the geosynclines coincide exactly with Mesozoic–Cenozoic mountain belts (Fig. 25 in [14]). The deterministic concept of the geosyncline, whereby it would inevitably initiate “a tectonic cycle” and evolve into a mountain belt, dominated geology for nearly a hundred years and the “theory” was praised as “a great – probably one of the greatest – unifying principles in geologic science” [78, p. 651].
The theory of the geosyncline appeared to respect the permanence of continents and oceans, restricting the troughs to narrow zones “lying between cratons, whether higher continental or lower oceanic cratons” [76, p. 1289] and thus seemed to reconcile permanence with the evidence of deep-water or even oceanic sediments in mountain belts: indeed, only the geosyncline appeared to explain the presence of deep-water deposits on land [92, p. 626]. However, Bertrand [17] and Suess [126] had shown that the structures of old and young mountain belts could be correlated across entire oceans like the Atlantic (Fig. 4) and speculated that, because former oceanic deposits could be uplifted to form mountain ranges, continents could also sink to oceanic depths. Admittedly, however, Bertrand and Suess primarily referred to vertical movements to explain the origins of oceans. Consequently, the paradox of old continents and young oceans could only be solved by moving continents [49,50] (Fig. 4).
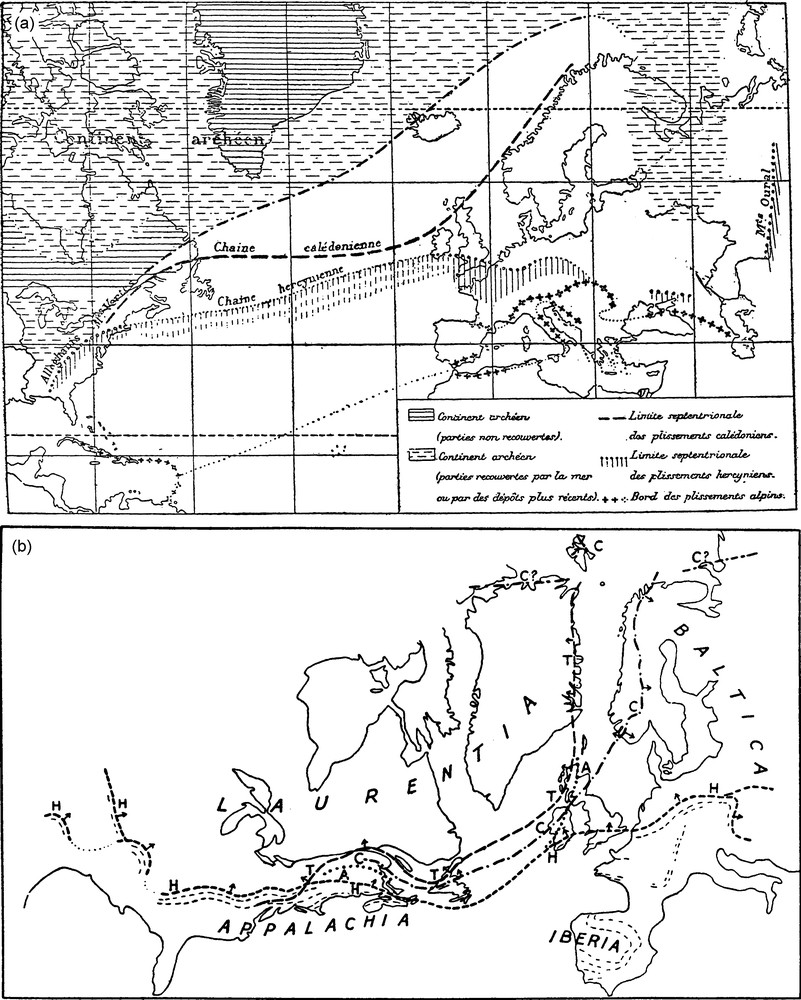
Correlation of the Palaeozoic orogenic fronts across the Atlantic, (a): by Bertrand [17]; (b): by Du Toit [49]: A: Acadian, C: Caledonian, H: Hercynian–Appalachian, T: Taconian. In (a) the dash–point–dash line, running northeast from the Allegheny Mountains, indicates the boundary of the Archean continent.
Fig. 4. Corrélations entre les fronts orogéniques paléozoïques au travers de l’océan Atlantique, (a) : par Bertrand [17] et (b) : Du Toit [49] : A : Acadien, C : Calédonien, H : Hercynien-Appalachien, T : Taconien. Dans (a), la ligne tiret–point–tiret allant du Nord à l’Est des montagnes Allegheny indique la limite du continent archéen.
3 The Steinmann Trinity
In 1905, Steinmann [116] noted the close association of serpentinized peridotites, diabase (basalt/dolerite) and radiolarian cherts along the western boundary of the Eastern Alps, where they appeared to occur always in the same tectonic unit. Steinmann was not the first to notice the consistent co-occurrence of these rocks that were going to bear his name [10,11]: by the early 19th century, Brongniart [26,27] had assembled serpentinites (“ophiolithe commun”), gabbro (“ophiolithe diallagique” and “euphotide”), basalt (“variolithe”) and chert (“jaspe”) under the name of ophiolite (the Greek synonym for serpentine; Fig. 1 in [1]). Brongniart [26] at first made no distinction between igneous and metamorphic rocks but, in his later classification [27] in which he distinguished the two groups, he placed the ophiolites with the igneous rocks. Cherts he thought to be metamorphic in origin, a view that prevailed through much of the 19th century, until the discovery of included radiolarian tests required a sedimentary origin [101].
Recognition of Brogniart's ophiolite association became commonplace among Italian geologists of the late 19th century [85,86,101] and the occurrence of “greenstones”, “roches vertes”, “pietre verdi” or “Grünschiefer” in orogenic belts became well known. It fell to Steinmann, however, to interpret this “consanguineous association” in geodynamic terms. Although he thought that all ophiolites, including diabase, spilite and “variolite” (variolitic pillow lava) were intrusive rocks distinctly younger than the overlying deep-water radiolarites and pelagic limestones, he emphasized their characteristic association with these deep-sea sediments [116,119]. He thus considered the ophiolites as characteristic for suboceanic environments from where these magmas had ascended during early folding of the oceanic sediments (Fig. 5). However, he considered the ophiolites of the Alps [116] and of the Apennines [120] to be passively involved in later thrusting and nappe emplacement, anticipating the concept of far-travelled ophiolite nappes. His vision of the ophiolites as a palaeotectonic element of prime importance led to the discovery of the nappe structure of the northern Apennines [117,118] and was a key element in unravelling the structural complexities of the Alpine mountain chains. Steinmann's general concepts survived, albeit in a somewhat different form, into the 1950s with Stille's [122] concept of initial (geosynclinal) magmatism.
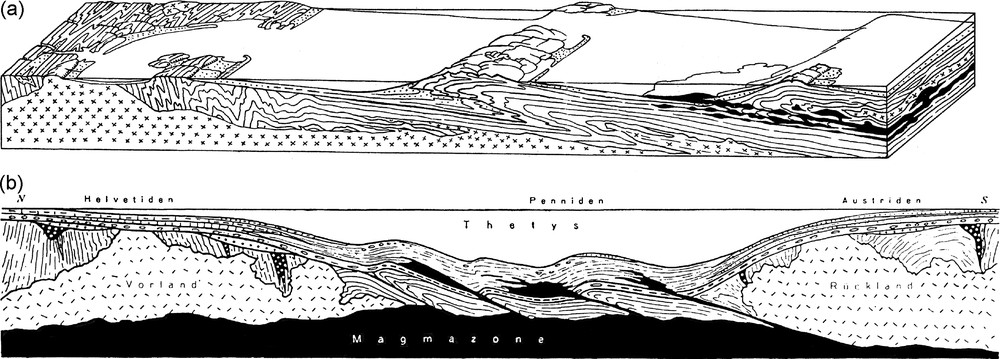
Cross-sections across the Alpine geosyncline. Steinmann's papers [116,120] do not include any illustrations; however, the profiles drawn by Argand [5, Plate 3] (a) and Staub [115, Fig. 41] (b), obviously influenced by Steinmann [116], well illustrate his early views, in particular the intrusion of the ophiolites along “embryonic” thrusts during early phases of Alpine orogeny, as postulated, for example, by Suess [127, p. 646]. Later, Steinmann [120] abandoned Suess’ notion of intrusion of ultramafic and mafic magmas along thrust planes. Masquer
Cross-sections across the Alpine geosyncline. Steinmann's papers [116,120] do not include any illustrations; however, the profiles drawn by Argand [5, Plate 3] (a) and Staub [115, Fig. 41] (b), obviously influenced by Steinmann [116], well illustrate his early ... Lire la suite
Fig. 5. Coupes au travers du géosynclinal alpin. Les articles de Steinmann [116,120] ne comportent pas d’illustrations, cependant, les profils dessinés par Argand [5, planche 3] (a) et Staub [115, Fig. 41] (b), influencés à l’évidence par Steinmann [116], illustrent les premières idées de ce dernier, en particulier l’intrusion d’ophiolites le long de charriages « embryonnaires » durant les phases précoces de l’orogénie alpine, ainsi que le postule Suess [127, p. 646]. Plus tard, Steinmann [120] abandonnera la notion d’intrusion le long de plans de charriage de Suess. Masquer
Fig. 5. Coupes au travers du géosynclinal alpin. Les articles de Steinmann [116,120] ne comportent pas d’illustrations, cependant, les profils dessinés par Argand [5, planche 3] (a) et Staub [115, Fig. 41] (b), influencés à l’évidence par Steinmann [116], illustrent les premières ... Lire la suite
Later, in 1927, Steinmann [120] modified his views on the emplacement of the ophiolites, postulating that they intruded as huge “plakoliths” between the continental crust and the geosynclinal sediments, whereby peridotites, gabbros and “diabase” were derived from the same ophiolitic magma. Like Lotti [84,86], he recognized their mutual age relationships, the older peridotites being cut by gabbroic dykes and the gabbros themselves by basaltic dykes. However, because he tried to explain the inter-relationships between the gabbroic dykes and the peridotites by intrusions of the former from below, he had to assume a rather complicated sequence of emplacement [75, pp. 33–34, Fig. 2]. Steinmann [116,120] also failed to appreciate the now obviously extrusive and subaqueous character of pillow lavas, recognized early in the 20th century [40]. Interestingly, even before the observation of basaltic pillows in the making [2], the observation of radiolarites as inter-pillow sediment [101] and the “curious fact that… diabase with a spherical or ellipsoidal structure” was “associated with [radiolarian] chert” had pointed to “submarine and possibly deep-sea lavas” [128, pp. 563–564].
4 Further evolution of the ophiolite concept
Like Steinmann, the majority of European and Alpine authors interpreted the ubiquitous co-occurrence of peridotites, gabbros and basalts, the latter commonly pillowed, as derived from one and the same ophiolitic magma [86,114]. In contrast to Steinmann [116,120], most Italian authors thought that ophiolites represented one single extrusive body, a sort of huge submarine lava flow that differentiated and crystallized on the sea floor [85,86, p. 272]. A similar interpretation was, apparently independently, advocated by French authors, notably Louis Dubertret and Jan H. Brunn. Also, Dubertret [46–48] and Brunn [28–30,32] considered the large ophiolite complexes of northern Syria and Greece as huge outpourings of basaltic magma that had moved along flexures separating a “craton” from the oceanic “geosyncline” [32, p. 117]. The basaltic magma was assumed to have differentiated by fractional crystallization within a huge “pluto-volcanic” body into peridotites, gabbros and more felsic rocks with a carapace of pillow lavas forming the quenched surface of these enormous extrusions (Fig. 6). However, the ratio of ultramafic to mafic rocks, estimated by Steinmann [120] in the Apennine ophiolite to be 5:2 and by Moores [93] in the Vourinos ophiolite (Greece) at about 4:1, could not be explained by normal differentiation of a basaltic magma [133].
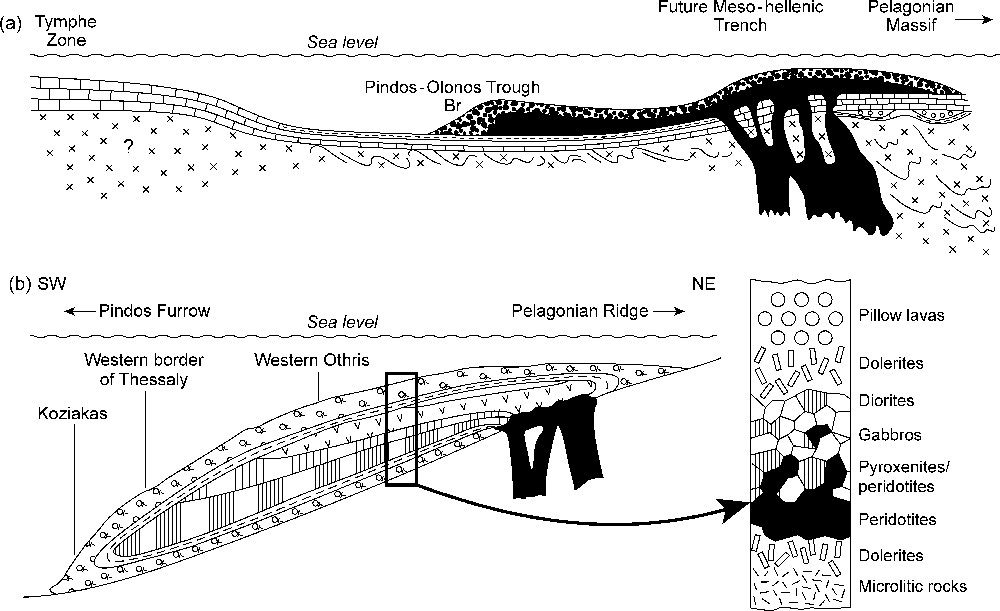
The “pluto-volcanic” complex of Brunn [30,32]. (a): schematic profile across the pluto-volcanic complex of northern Greece. Mafic magma is extruded along the flexure between the oceanic geosyncline (Pindos Trough) and the “cratonic” Pelagonian continental basement. Black and white speckled area on top of the ultramafic and mafic rocks (black) represents the early lithified microlithic and doleritic crust; Br signifies ophiolitic breccias developed at the front of the flow. The feeder dykes of the pluto-volcanic complex are purely hypothetical; no such feeder dykes have ever been observed within the Pelagonian basement. Redrawn from Brunn [32, Fig. 1]; (b): internal differentiation of the pluto-volcanic complex, redrawn from [8, Figs. 36 and 37]. Masquer
The “pluto-volcanic” complex of Brunn [30,32]. (a): schematic profile across the pluto-volcanic complex of northern Greece. Mafic magma is extruded along the flexure between the oceanic geosyncline (Pindos Trough) and the “cratonic” Pelagonian continental basement. Black and white speckled ... Lire la suite
Fig. 6. Complexe « volcano-plutonique » de Brunn [30,32]. (a) : coupe schématique au travers du complexe volcano-plutonique du Nord de la Grèce. Le magma mafique est extrudé le long de la flexure entre le géosynclinal océanique (Pindos Trough) et le socle continental « cratonique » pélagonien. La zone mouchetée blanc et noir au sommet des roches ultramafiques et mafiques (en noir) représente la croûte doléritique et microlithique lithifiée de manière précoce ; Br signifie brèches ophiolitiques développées au front de l’extrusion. Les dykes d’alimentation du complexe volcano-plutonique sont purement hypothétiques ; aucun dyke d’alimentation de ce type n’a jamais été observé dans le socle pélagonien. Redessiné d’après Brunn [32, Fig. 1] ; (b) : différenciation interne du complexe volcano-plutonique, redessiné d’après [8, Figs. 36 et 37]. Masquer
Fig. 6. Complexe « volcano-plutonique » de Brunn [30,32]. (a) : coupe schématique au travers du complexe volcano-plutonique du Nord de la Grèce. Le magma mafique est extrudé le long de la flexure entre le géosynclinal océanique (Pindos Trough) et le socle continental ... Lire la suite
Like Steinmann, Lotti had recognized the same “stratigraphy” and cross-cutting relationships within the ophiolites, but interpreted the latter as an essentially autochthonous extrusion on the “Eocene” sea floor [86, p. 272]. Because the sediments overlying the “diabase” had to be younger (Fig. 7a), he came into conflict with Steinmann [118], for whom the oceanic stratigraphy of Jurassic radiolarites and Cretaceous pelagic limestones and shales represented the host rocks of the intrusive (Cretaceous) ophiolites (Fig. 7b). For Steinmann [120], the lack of feeder dykes was a further argument for the allochthonous nature of the Ligurian ophiolites and the nappe structure of the Apennines. At about the same time, the ophiolites of the Semail (Oman) were interpreted as a huge thrust sheet or nappe [81]. With time, ophiolites, particularly serpentinites, became the petrological signatures of the central zones of orogens [67] and finally, within the context of plate tectonics, of oceanic sutures in zones of continental collision where they represented relics of oceanic lithosphere obducted onto continental margins [33,41,80].
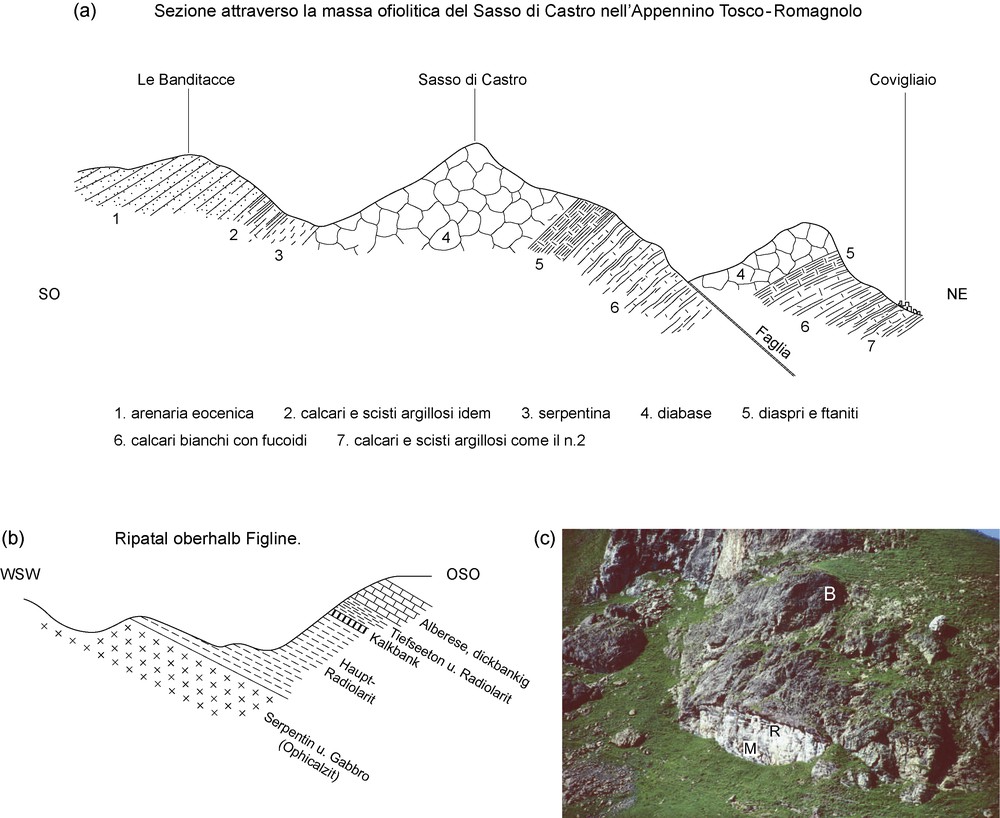
(a): in this tectonically inverted section, Lotti [86, Fig. 27] interpreted the ophiolites (3: “serpentina” and 4: “diabase”) as extrusions emplaced on the Eocene seafloor (1: “arenaria” [presumably Macigno, Oligocene flysch] and 2: “calcari e scisti argillosi” [Alberese of the Subligurian nappe]). As a consequence of this anti-mobilist interpretation, the overlying sequence of pelagic sediments was also required to be Eocene in age (5: “diaspri e ftaniti” = radiolarites, Upper Jurassic; 6: “Calcari bianchi a fucoidi” = Maiolica, Lower Cretaceous, 7: “Calcari e scisti argillosi come il no. 2” = Argille a Palombini, Lower Cretaceous). This stratigraphy is obviously in contrast to Steinmann's [118, Fig. 1] stratigraphy in (b) that shows the ophiolites (“serpentine and gabbro, ophicalcite”) to be overlain by radiolarites, deep-sea clay (Upper Jurassic) and Alberese (here limestone with Calpionella = Maiolica, Lower Cretaceous). Steinmann interpreted the contact between the ophiolites and the radiolarites (wrongly) as intrusive, although he could find no indications of metamorphism; (c): tectonically inverted sequence of Maiolica-type pelagic limestones (M: Lower Cretaceous) and radiolarites (R: Middle to Upper Jurassic) overlying basaltic pillow lavas and breccias (B) along a depositional contact. Val Savriez (Sursés, eastern Switzerland). Such tectonically inverted sequences might have led Steinmann [116,120] to suggest intrusion of the ophiolites into the deep-sea sediments of the geosyncline. Masquer
(a): in this tectonically inverted section, Lotti [86, Fig. 27] interpreted the ophiolites (3: “serpentina” and 4: “diabase”) as extrusions emplaced on the Eocene seafloor (1: “arenaria” [presumably Macigno, Oligocene flysch] and 2: “calcari e scisti argillosi” [Alberese of the ... Lire la suite
Fig. 7. (a) : dans cette coupe tectoniquement inversée, Lotti [86, Fig. 27] a interprété les ophiolites (3 : « serpentina » et 4 : « diabase ») comme des extrusions sur le plancher océanique éocène (1 : arenaria [probablement Macigno, flysch oligocène] et 2 : calcari e scisti argillosi [Alberese de la nappe sub-ligure]). En conséquence de cette interprétation antimobiliste, la séquence de sédiments pélagiques doit être nécessairement d’âge Éocène (5 : « diaspri e ftaniti » = radiolarites, Jurassique supérieur ; 6 : « Calcari bianchi a fucoidi » = Maiolica, Crétacé inférieur ; 7 : « Calcari e scisti argillosi comme no 2 » = Argille a Palombini, Crétacé inférieur). Cette stratigraphie est à l’évidence en opposition avec celle de Steinmann [118, Fig. 1] (b) en ce qu’elle montre les ophiolites (« serpentine et gabbro, ophicalcite ») recouvertes par des radiolarites, des argiles d’eau profonde (Jurassique supérieur) et Alberese (ici, calcaire à Calpionella = Maiolica, Crétacé inférieur). Steinmann interprétait les ophiolites, de manière erronée, comme étant intrusives, bien qu’il n’ait pu trouver aucune indication de métamorphisme ; (c) : séquence tectoniquement inversée de calcaires pélagiques de type Maiolica (M : Crétacé inférieur) et radiolarites (R : Jurassique moyen à supérieur) recouvrant les pillow lavas et les brèches basaltiques (B) le long d’un contact de dépôt. Val Savriez (Sursés, Suisse orientale). De telles séquences tectoniquement inversées pouvaient avoir conduit Steinmann [116,120] à suggérer l’intrusion des ophiolites dans les sédiments de mer profonde du géosynclinal. Masquer
Fig. 7. (a) : dans cette coupe tectoniquement inversée, Lotti [86, Fig. 27] a interprété les ophiolites (3 : « serpentina » et 4 : « diabase ») comme des extrusions sur le plancher océanique éocène (1 : arenaria [probablement Macigno, flysch oligocène] et 2 : calcari e scisti ... Lire la suite
5 The case of the (serpentinized) peridotites
The observed predominance of (serpentinized) peridotites in Alpine-type ophiolites seemed to call for the existence of peridotitic magmas: consequently, Alpine-type peridotites [12] were considered to be intrusions emplaced during early orogenic movements. Whereas most European authors emphasized the Steinmann Trinity, that is, the close association of the peridotites and gabbros with the volcanic and sedimentary rocks [114], many Anglo-Saxon writers thought that the peridotites were unrelated to the volcanics [12] and Hess [67, p. 393] cautioned that “mapping of the ophiolite group as a unit has often obscured critical relationships of its members to the tectonic cycle”, even though he had previously stressed the close spatial association of serpentinites, volcanics and sediments [64]. Either way, until the early 1960s, peridotites were generally considered to be magmatic, and the importance of metamorphic tectonite fabrics was only recognized in the late 1960s [37,93,94].1 However, unnoticed by many European authors, Bowen [23,24] had shown experimentally, on the one hand, “that such magmas are not possible” under reasonable conditions in the crust and that, on the other hand, serpentine minerals were unstable above 550 °C: he therefore argued that peridotites formed chiefly by fractional crystallization of basaltic magma and gravitational segregation as in large stratiform mafic–ultramafic complexes. In spite of Bowen's early experiments and although practically all authors agreed that there were (with perhaps the exception of the ophicalcites [116,120]) no indications of thermal metamorphism at the contacts of peridotites or serpentinites with their presumed host rocks, the close association, commonly in one and the same outcrop, with volcanic and sedimentary rocks was taken as evidence for a shallow intrusive origin [64,67]. Bailey and McCallien [10,11] even called for “serpentine lavas”, although Bowen and Tuttle [25] had shown by experiments that no such serpentine magma could exist. A fundamental controversy developed between Hess, who pleaded for a “hydrous ultramafic magma” (that would crystallize at lower temperature [64]) and who “cast his vote with the field geologist” [67, p. 391] and Bowen who, in order to overcome the theoretical difficulties, envisaged solid-state, “cold” intrusion of peridotites [25]. The controversy lasted for years [139] and faded only after Bowen's death in 1956 when it was recognized that: peridotites were the (partly hydrated) residues of partial melting of the mantle that had produced the igneous rocks of the ocean crust; that the reaction of circulating sea water with young oceanic lithosphere produced the serpentinization of the peridotites [68] and that the ultramafic rocks of the ophiolite suites were fragments of the Earth's mantle tectonically emplaced into orogens [38] (Table 2).
Interpretation of ophiolites from the time of Steinmann [116,120] to the present day [14].
Tableau 2 Interprétation des ophiolites du temps de Steinmann [116,120] jusqu’à nos jours [14].
Steinmann (1905,1927) | Today | Today |
Ocean–continent transition | Spreading ridge | |
Ophiolites are a consanguineous association, derived from an ophiolitic magma | Mantle and magmatic rocks are genetically unrelated | Magmatic rocks are the result of partial melting of the sub-oceanic mantle |
Peridotites are magmatic rocks | Peridotites are tectonically exhumed subcontinental mantle rocks (± modified by mantle fluids) | Peridotites are more or less depleted melt residues |
Pillow lavas are intrusive rocks | Pillow lavas are submarine extrusions | Pillow lavas are submarine extrusions |
Ophiolites intruded as huge “plakoliths” between sial and oceanic sediments | Ophiolites are an association of subcontinental mantle and igneous rocks derived from MORB-melts | Ophiolites are asthenosphere-derived oceanic lithosphere |
Granites are tectonic slivers of continental crust transported with the ophiolite nappes | Granites are typically continental basement rocks, tectonically emplaced on exhumed mantle rocks | Plagiogranites are magmatic differentiates |
Ophicalcites are the product of contact metamorphism related to ophiolite intrusion | Ophicalcites are tectono-sedimentary breccias related to tectonic mantle exhumation along: | |
low-angle detachment faults | transform faults and normal faults along slow-spreading ridges | |
Deep-sea sediments are the bottom of the “geosyncline” intruded by the ophiolites | Deep-sea sediments are interbedded with pillow lavas and/or stratigraphically overlie the oceanic basement | |
6 The geosyncline revisited: actualistic models
In the early 20th century, the concept of the geosyncline became increasingly confusing. Beside the classical orthogeosyncline with its miogeosyncline–eugeosyncline couplet [123], many special cases, including non-orogenic geosynclines, were distinguished because their sedimentary basins did not fit into the classical model with the non-volcanic miogeosyncline accompanied by the eugeosyncline containing prominent igneous rocks [76]. However, whereas the distinction of different types of non-orogenic geosynclines “within cratons” resulted in a type of basin classification (Table 3), the miogeosyncline–eugeosyncline couplet resisted an actualistic interpretation for many years. At an early stage, European workers had compared the Alpine geosyncline to the island arcs and deep troughs of Indonesia [62,71,91]. In the wake of the Dutch studies in this area [131,110], the Indonisian island arcs and the intervening deep troughs appeared finally to provide an actualistic model both for the American geosyncline [65,67,76], equating its igneous rocks to those of island arcs, and for the European geosyncline that emphasized trenches and deep-water sediments [129].
Approximate correlation of the historical geosynclinal nomenclature with current terminology.
Tableau 3 Corrélation approximative de la nomenclature historique du géosynclinal avec la terminologie courante.
Geosyncline | Current terminology |
Taphrogeosyncline/Aulacogen | Rift |
Autogeosyncline | Cratonic or Intracratonic basin |
Miogeosyncline | Passive or Atlantic-type margin |
Paraliageosyncline | Delta-dominated passive margin |
Leptogeosyncline | Starved oceanic deep-water basin |
Eugeosyncline (European tradition) | Oceanic crust and sediments (“Steinmann Trinity”) |
Eugeosyncline (American tradition) | Island arc/Active margin systems |
Epi-Eugeosynclines | Fore-arc and back-arc basins |
With the rapid progress of marine geological and geophysical exploration of oceans and continental margins after World War II, a reevaluation of the geosynclinal concept could be undertaken. Equating the thick shallow-water deposits underlying the Atlantic continental shelf of the United States with the miogeosyncline, the deep-water sediments under the continental slope and rise with the eugeosyncline, Drake et al. [45] could compare this sedimentary system directly with that of the Appalachians, as reconstructed by Kay [77]. The modern analogue included also a basement high below the shelf break that was correlated with an apparently similar feature between the miogeosyncline and the eugeosyncline of Kay [77]; the only misfit appeared to be the lack of modern igneous activity, the distinctive ingredient of the Appalachian eugeosyncline (Fig. 8a–c).
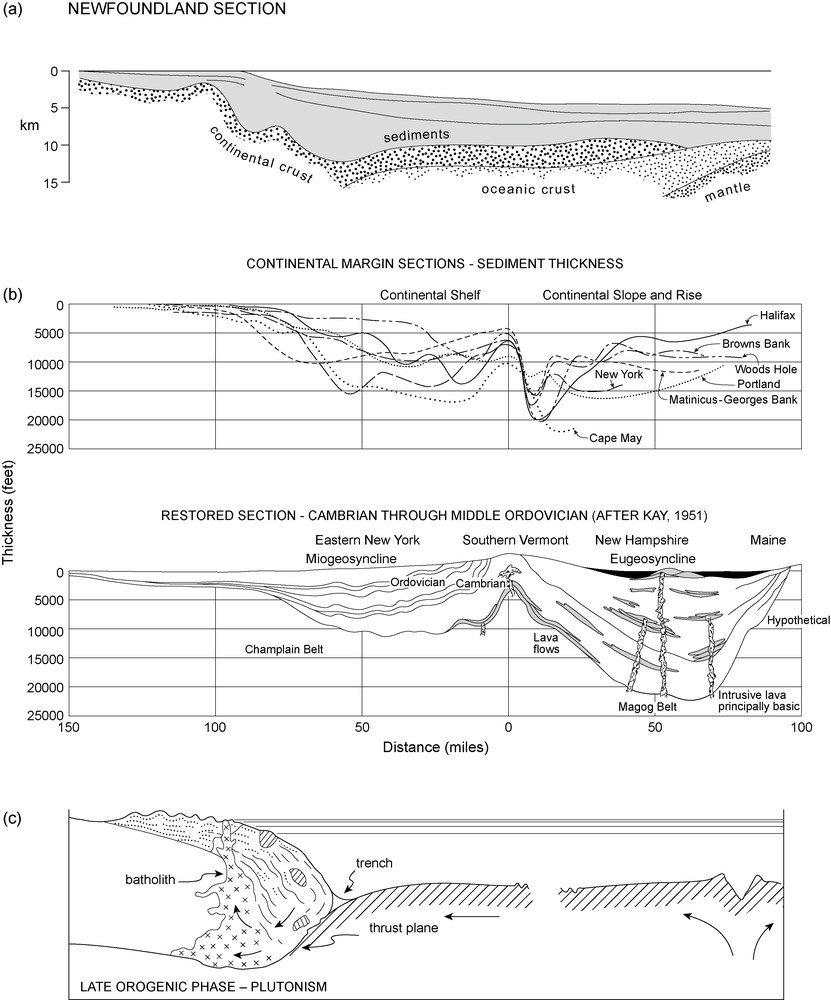
(a)–(c): the miogeosyncline–eugeosyncline couplet in the interpretation of Drake et al. [45]: a: cross-section of the Atlantic continental margin, Newfoundland from seismic refraction measurements [45, Fig. 35]; (b) and (c): comparison between sediment thickness distribution across the Atlantic margin (b) and the restored geosynclinal section of Kay [77] (c), both from [45, Fig. 30]; (d): the actualistic concept of geosynclines of Dietz showing the “geosyncline” during its late orogenic or plutonic period [43, Fig. 1D].
Fig. 8. (a)–(c) : le couple miogéosynclinale-eugéosynclinale dans l’interprétation de Drake et al. [45]. a : coupe dans la marge continentale atlantique de Terre-Neuve, à partir de mesures de sismique réfraction [45, Fig. 35] ; (b) et (c) : comparaison entre la distribution des épaisseurs sédimentaires au travers de la marge atlantique (b) et paléocoupe du géosynclinal de Kay [77] (c), les deux selon [45, Fig. 30] ; (d) : concept actualiste des géosynclinaux de Dietz montrant le géosynclinal au cours de sa période orogénique ou plutonique tardive [43, Fig. 1D]. Masquer
Fig. 8. (a)–(c) : le couple miogéosynclinale-eugéosynclinale dans l’interprétation de Drake et al. [45]. a : coupe dans la marge continentale atlantique de Terre-Neuve, à partir de mesures de sismique réfraction [45, Fig. 35] ; (b) et (c) : comparaison entre la distribution des épaisseurs ... Lire la suite
In a number of papers, Dietz [43] developed this concept further (with, however, the notable exception of Tethyan examples “where the collision of sialic blocks seems to have dominated the scheme”, p. 315). He also correlated the characteristic eugeosynclinal greywackes with modern continental-rise turbidites of Atlantic-type margins. With time “the continental rise is compressed, folded, and thrust against the continental raft, being marginally added to it.” [43, p. 316] (Fig. 8d): only later would a trench and batholiths form. However, in the frame of plate tectonics, “miogeo[syn]clines form on trailing edges of diverging continents, whereas orthogeo[syn]clines (i.e. the American eugeosynclines) form near active leading edges of converging lithospheric plates” [44, p. 1]. Although a number of authors tried to keep the geosyncline alive, with the arrival of the “New Global Tectonics” [73], the concept had obviously become obsolete [34].
7 From the Steinmann Trinity to sea-floor spreading
A first step towards a mobilist understanding of the ophiolites was the extension of the geosyncline to oceanic dimensions. Following Argand [6, p. 299], Bailey [9, p. 1719] placed the Steinmann Trinity into the context of continental drift: “E. Argand has developed Steinmann's view and has pictured geosynclines as determined by stretching, by continental drift apart, which attenuates the Sial layer and eventually allows Sima to reach the bottom of the sea at bathyal or abyssal depth. If such drift-separation continues, a new ocean bed may be developed, covered with the products of submarine eruptions” (Fig. 9). These almost prophetic words were hardly noticed at Bailey's time, but anticipated the statement made nearly 30 years later by Hess [69, p. 331]: “Dredging on oceanic ridges has recently brought to light the suite of rocks characterizing this environment… This suite is very familiar to me: it is Steinmann's Trinity of the Alpine ophiolite belts, that is characteristically serpentinized peridotite, pillow lavas often spilitic, and radiolarian cherts.”
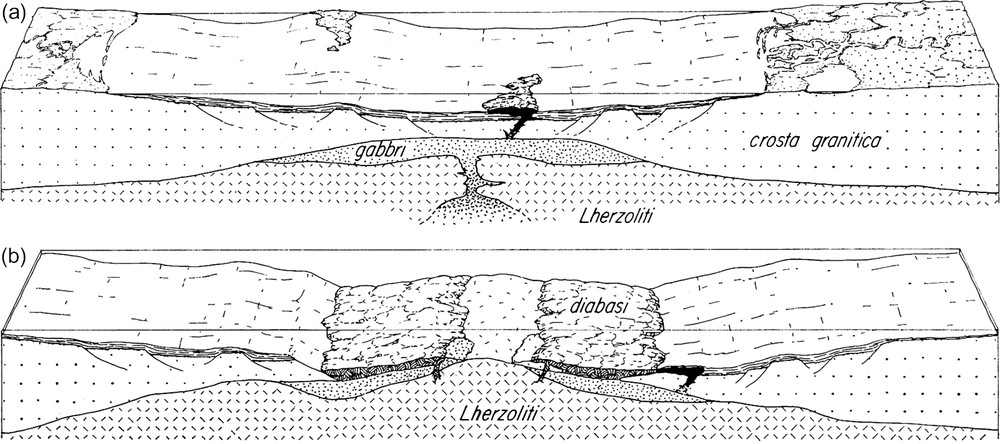
“Early stages of geosynclinal evolution”; above, from Triassic to Middle Jurassic times (a); below, in the Late Jurassic (b) after continental break-up, from [53, Fig. 3]. These cross-sections across the “geosyncline” of the northern Apennines, which conform to modern views of ocean–continent transitions, can be compared with the views of Argand [6] and Bailey [9]: extension of the continental crust (crosta granitica) along oceanward-dipping normal faults leads to the exhumation and submarine exposure of mantle rocks (lherzoliti) and mafic intrusives (gabbri), the associated basalts (diabasi) forming “a new seabed… covered with products of submarine eruptions”, as proposed by Bailey [9, p. 1719]. Masquer
“Early stages of geosynclinal evolution”; above, from Triassic to Middle Jurassic times (a); below, in the Late Jurassic (b) after continental break-up, from [53, Fig. 3]. These cross-sections across the “geosyncline” of the northern Apennines, which conform to modern views ... Lire la suite
Fig. 9. « Stades précoces de l’évolution géosynclinale » ; en haut, du Trias au Jurassique moyen (a) ; en bas à la fin du Jurassique (b), après le break-up continental, d’après [53, Fig. 3]. Ces coupes au travers du « géosynclinal » du Nord des Apennins, conformément aux idées modernes sur les transitions océan–continent, peuvent être comparées aux idées d’Argand [6] et Bailey [9] : l’extension de la croûte continentale (crosta granitica), le long de failles normales à pendages vers l’océan conduit à l’exhumation et à l’exposition sous-marine de roches du manteau (lherzoliti) et de roches mafiques intrusives (gabbri), les basaltes associés (diabasi) formant une nouvelle couche marine recouverte de produits issus d’éruptions sous-marines (a new seabed… covered with products of submarine eruptions), comme le propose Bailey [9, p. 1719]. Masquer
Fig. 9. « Stades précoces de l’évolution géosynclinale » ; en haut, du Trias au Jurassique moyen (a) ; en bas à la fin du Jurassique (b), après le break-up continental, d’après [53, Fig. 3]. Ces coupes au travers du « géosynclinal » du Nord des ... Lire la suite
A comparison between the ophiolites of the eastern Mediterranean and the rocks dredged from the mid-Atlantic Ridge had already been made by Brunn [31], although within the context of his pluto-volcanic extrusion model [30,32]. However, in the very area of Brunn's field work, where the Vourinos ophiolite complex crops out, Moores [93] made a critical distinction between a harzburgite tectonite mantle and an only weakly deformed magmatic sequence of ultramafic cumulates and gabbros, overlain by dolerite dykes and lavas and finally capped by pelagic sediments. He recognized that “the general suite of rocks is similar to that found in oceanic ridge regions today, implying that the ophiolites were derived in the same manner” [93, p. 60, Fig. 12]. However, he was led astray by major figures in Alpine geology to write that “most evidence suggests that the Tethyan mountain system developed on continental crust [8,130] and that true oceanic conditions were never really involved”, not recognizing the allochthonous position of the ophiolite [13,140].
The final break-through regarding the oceanic interpretation of the ophiolites happened in Cyprus where Gass and Masson-Smith [58] interpreted the huge positive gravity anomaly covering the island as being the geophysical expression of an extensive mantle slab, derived from an oceanic Mediterranean and imbricated with African crust. The Troodos Massif, occupying the centre of the gravity anomaly, was interpreted as an oceanic island, whose plutonic core was exposed in its centre. The authors wrote that the plutonic complex “might in fact represent the sub-Mohorovičić peridotitic material [upper mantle] which has partially fused to provide the volcanic material of the Sheeted Intrusive Complex and the Pillow Lava Series”. Five years later, Gass [57] asked “is the Troodos Massif of Cyprus a fragment of Mesozoic ocean floor?” This question was finally answered in the affirmative by Moores and Vine [94], who documented the residual oceanic mantle sequence and the overlying magmatic oceanic crust. They correlated the ophiolite stratigraphy with the oceanic layers 2 to 4 of Raitt [104] and placed the Mohorovičić Discontinuity at the boundary between the ultramafic cumulates (Layer 4) and the gabbros (Layer 3), equating the ophiolites with oceanic lithosphere. The Troodos ophiolite of Cyprus thus became the first studied piece of oceanic lithosphere exposed on land. Ever since, “by repeatedly drilling through abyssal sediments into mafic basement, the recent DSDP has vindicated the European [i.e., Argand's and Bailey's] view” [44, p. 2].
8 Conclusions: the Steinmann Trinity in the context of ocean–continent transitions
It appears that Gustav Steinmann never saw a “complete” ophiolite as defined by the 1972 Penrose Conference on Ophiolites [4]. Most, if not all, occurrences of the Steinmann Trinity in the Alps and Apennines are “incomplete ophiolites” and can now be related to fossil OCT's (Table 2, Fig. 10) [15,39] and/or to an incipient slow-spreading ridge [79]. These “ophiolites” are very different from those of the eastern Mediterranean (e.g., Vourinos, Cyprus) and the Middle East (Oman) that are interpreted as derived from fast-spreading lithospheric segments produced along a mid-ocean ridge [94,100] or in a supra-subduction zone setting [90,102]. The Alpine–Apennine examples are dominated by serpentinized peridotites; gabbros form only relatively small shallow intrusions within them and no relics of a sheeted-dyke complex are found; furthermore, there exists no genetic link between the peridotites and the magmatic rocks [39,105,106]. Indeed, the mantle material, although affected by the infiltration of Mid-Ocean Ridge Basalt melts [97], has proved to be derived from the subcontinental mantle lithosphere [96,103], whence it was transported to the ocean floor. Pillow lavas and oceanic sediments stratigraphically overlie the serpentinized mantle rocks exhumed along a system of detachment faults exposed on the sea bed (Fig. 9, Fig. 11a) [39,53,56,82]. The traces of these faults on the sea floor are marked by tectono-sedimentary breccias, Brongniart's [26] ophicalcites, which find their counterparts along the Iberian ocean–continent transition (Fig. 10c, e). Indeed, the occurrences of the Steinmann Trinity in the Alps find an almost perfect analogue in the Iberian margin of the Cretaceous Atlantic [87]: in both areas, extensional allochthons of continental crust and pre-rift sediments overlie the exhumed mantle rocks along low-angle detachments [87] (Fig. 11b).
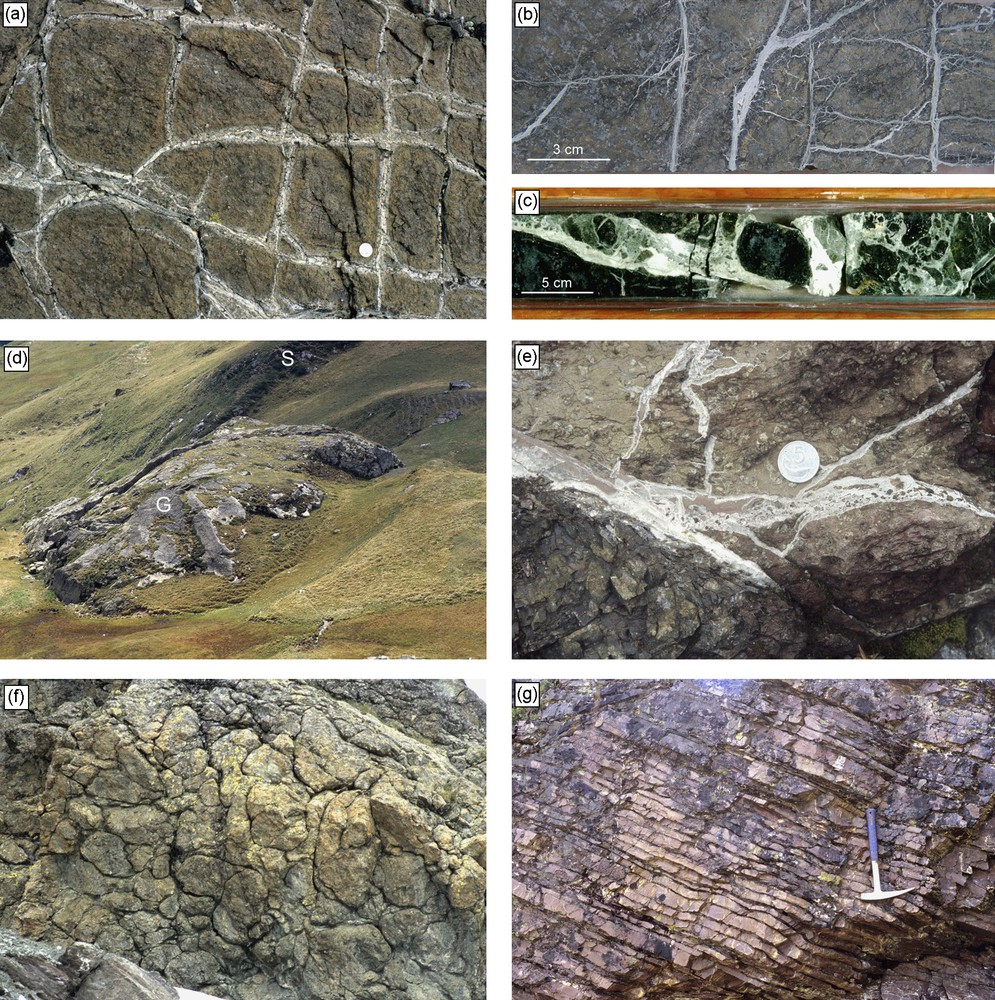
The rocks of ocean–continent transitions: (a), (b): peridotite mylonites with a mantle foliation cut by serpentine veins: (a): Totalp nappe, Totalp (Davos, eastern Switzerland), diameter of coin: 20 mm; (b): Iberia Abyssal Plain, ODP Leg 173, Site 1068A, Core 24-1, 69–84 cm; (c), (e): ophicalcites: (c): serpentinized peridotite with fractures filled by serpentinite fragments and calcite cement, ODP Leg 149, Site 897C, Core 65R1, 28–61 cm; (d): small, shallow intrusion of gabbro (G) into partially serpentinized peridotite (S), exhumed by differential weathering, Middle Jurassic (161 Ma), Platta nappe, Val da Natons (Sursés, eastern Switzerland); the intrusion is about 120 m across; (e): typical fabric of Alpine ophicalcite: replacement of serpentine minerals is illustrated by relics of pyroxene crystals floating in a red microsparitic limestone matrix, reflecting the original texture of the exhumed and tectonically fractured mantle rock. Replacement has been followed by sea-floor carbonate cementation and subsequent geopetal infilling by red pelagic and/or diagenetic sediment, Totalp nappe, Totalp (Davos, eastern Switzerland), diameter of coin: 20 mm; (f): basaltic pillow lava, Middle Jurassic, Arosa zone, Flimspitz (Paznauntal, western Austria), pillows are several decimetre to about 1 m across; (g): ribbon chert (radiolarites, Middle to Upper Jurassic), Arosa zone, Verborgen Wäng, Arosa, eastern Switzerland), length of hammer: 33 cm. Masquer
The rocks of ocean–continent transitions: (a), (b): peridotite mylonites with a mantle foliation cut by serpentine veins: (a): Totalp nappe, Totalp (Davos, eastern Switzerland), diameter of coin: 20 mm; (b): Iberia Abyssal Plain, ODP Leg 173, Site 1068A, Core 24-1, 69–84 cm; (c), (e): ophicalcites: ... Lire la suite
Fig. 10. Roches des transitions océan–continent : (a), (b) : mylonites péridotitiques avec foliation du manteau recoupée par des veines de serpentine : (a) : Nappe de Totalp (Davos, Suisse orientale), diamètre de la pièce : 20 mm ; (b) : Plaine Abyssale Ibérique, Leg ODP 173, Site 1068A, Carotte 24-1, 69–81 cm ; (c), (e) : ophicalcites : (c) : péridotite serpentinisée, avec fractures remplies de fragments serpentineux et ciment calcaire, Leg ODP 149, Site 897C, Carotte 65R1, 28–61 cm ; (d) : petite intrusion peu profonde (sur ± 120 m) de gabbro (G) dans une péridotite partiellement serpentinisée (S), exhumée par altération différentielle, Jurassique moyen (161 Ma), nappe de Platta Val da Natons (Sursés, Suisse orientale) ; (e) : fabrique typique de l’ophicalcite alpine : le remplacement de minéraux serpentineux est illustré par des reliques de cristaux de pyroxène flottant dans une matrice rouge de calcaire microsparitique, reflétant la texture originelle des roches mantelliques exhumées et fracturées tectoniquement. Le remplacement a été suivi d’une cimentation calcitique, puis d’un remplissage géopète par un sédiment rouge, pélagique ou diagénétique, nappe de Totalp (Davos, suisse orientale), diamètre de la pièce : 20 mm ; (f) : pillow lava basaltique, Jurassique moyen, zone d’Arosa (Paznauntal, Autriche occidentale), les pillows ont une taille de quelques décimètres à 1 m ; (g) : chert rubané (radiolarites, Jurassique moyen à supérieur), zone d’Arosa, (Verborgen Wäng, Arosa, Suisse orientale). Longueur du marteau 33 cm. Masquer
Fig. 10. Roches des transitions océan–continent : (a), (b) : mylonites péridotitiques avec foliation du manteau recoupée par des veines de serpentine : (a) : Nappe de Totalp (Davos, Suisse orientale), diamètre de la pièce : 20 mm ; (b) : Plaine Abyssale Ibérique, Leg ODP 173, Site 1068A, Carotte 24-1, ... Lire la suite
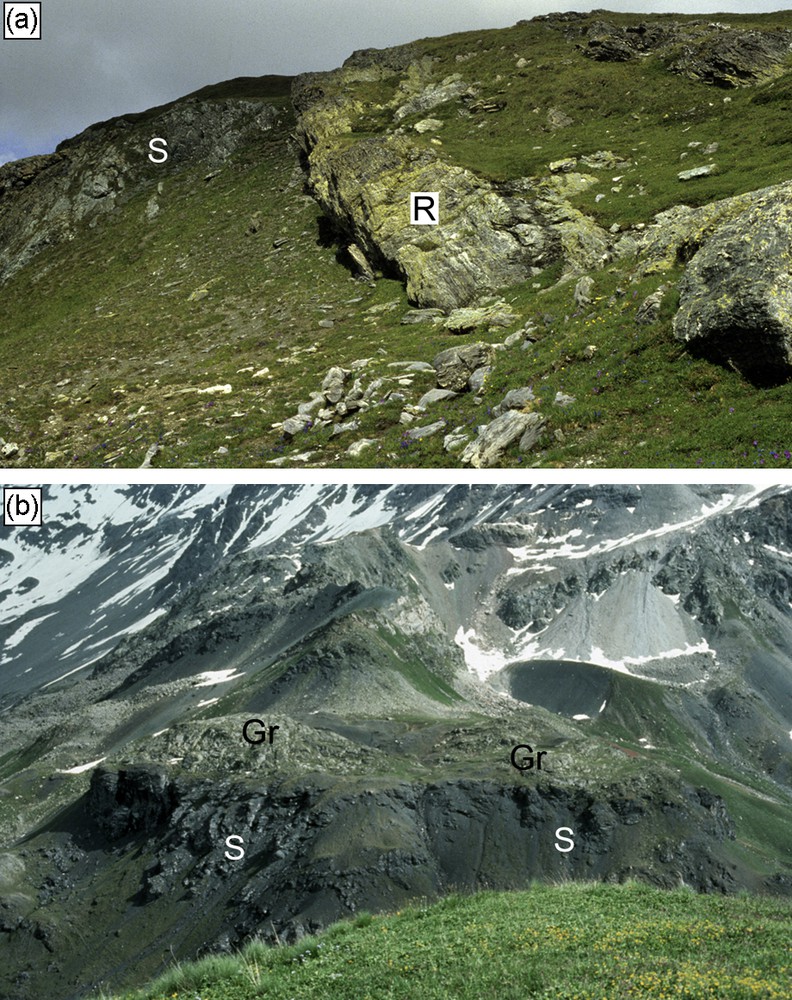
(a): radiolarites (R) stratigraphically overlying serpentinized peridotites (S). The Radiolarite Formation (Middle to Upper Jurassic) includes sedimentary breccias with serpentinite and dolomite clasts, the latter derived from prerift sediments in extensional allochthons, Plang Tguils (Sursés, eastern Switzerland); (b): an extensional allochthon of cataclastic post-Variscan granite (Gr) overlies exhumed subcontinental mantle rocks (serpentinized peridotites: S) of the Platta nappe along a tectonic contact of Jurassic age, Parsettens (Val d’Err, eastern Switzerland).
Fig. 11. (a) : radiolarites (R) recouvrant stratigraphiquement des péridotites serpentinisées (S). La Radiolarite Formation (Jurassique moyen à supérieur) comporte des brèches sédimentaires à clasts de serpentinite et dolomie, ceux-ci dérivant de sédiments prérift d’allochtones en extension, Plang Tguils (Sursés, Suisse orientale) ; (b) : allochtone d’extension d’un granite cataclastique post-varisque (Gr) recouvrant des roches mantelliques subcontinentales (péridotites serpentinisées : S) de la nappe de Platta le long d’un contact tectonique d’âge Jurassique, Parsettens (Val d’Err, Suisse orientale).
In retrospect, it appears that around 1930 most geological ingredients were available for a modern, mobilist view of the Earth's tectonic evolution. Isostasy and the, admittedly scarce, dredgings of almost exclusively mafic and ultramafic rocks from the deep-ocean floor showed the crust of continents and oceans to be fundamentally different; faunal endemism and palaeobiogeography showed that connections had existed between major land masses; the fit of the continents was compatible with their formerly having been contiguous [49,135,136]; radiogenic heat production supported the possibility of mantle convection [70] and the extension of the geosyncline to oceanic dimensions [6,9,116,119] called for the disappearance of large oceanic areas. However, as in the case of the ultramafic rocks, “the problem remained unsolved, some vital piece of evidence was still missing” [67, p. 402]. This “vital piece” was the recognition that (serpentinized) peridotites were either pieces of exhumed subcontinental lithosphere or the refractory residues from partial melting during the process of sea-floor spreading: a process that was only recognized by the potent mixture of experimental petrology and investigation of the modern ocean by geological and geophysical means.
Acknowledgments
We thank the Académie des sciences and J. Dercourt, P. Huchon, G. Manatschal and G. Péron-Penvidic for their kind invitation and the opportunity to present this paper at the Ocean–Continent-Transition Meeting, September 19th to 21st 2007 in Paris. G. Manatschal kindly provided Figs. 10b, 10c and 11b. We thank Enrico Bonatti and an anonymous reviewer for their constructive critiques. We are grateful to Hélène Paquet for the translation of the abstract and the figure captions and to Marina Jimenez for much help in editing the paper.
1 Indeed, the occurrence of solid-state deformation had been observed in various peridotites already in the 1930s [100, p. 5], albeit in subcontinental mantle rocks [3] or mantle xenoliths in basalt [54].