1 Introduction
The Variscan orogeny results from the collision of Gondwana and/or Gondwanian derived-microplates with the Laurussia megacontinent [27]. The early orogen history consists of nappe stacking and subsequent crustal thickening, associated with regional Barrovian metamorphism lasting until Middle Carboniferous times [27]. In contrast, the Late Carboniferous period is, for the most part, characterized by the emplacement of voluminous granites into hot migmatitic crust. This later evolution has resulted in the intense erosion of exhumed deep-crustal rocks and the formation of intracontinental sedimentary basins along normal faults. In the French Massif Central, this late evolution is generally interpreted as a result of the reequilibration of the thickened hot and weak Variscan crust, during a postcollisional extensional regime [16,25]. An extension is generally invoked for the formation of dome structures, such as the Velay granite-migmatite complex [13,24].
The Maures-Tanneron massif (MTM) (SE France) forms a part of the Crystalline Province (Fig. 1), which constitutes one of the southernmost segments of the Variscan belt in France [27]. This massif represents an intriguing problem in the frame of the Variscan belt since its tectonic and metamorphic evolution is quite different from that observed in the other parts of the belt. In particular, the obliquely convergent–transpressive character of deformation and the polyphase, compressive nature of the exhumation of hot orogenic lower crust are generally not taken into account in the current models of the Variscan belt.
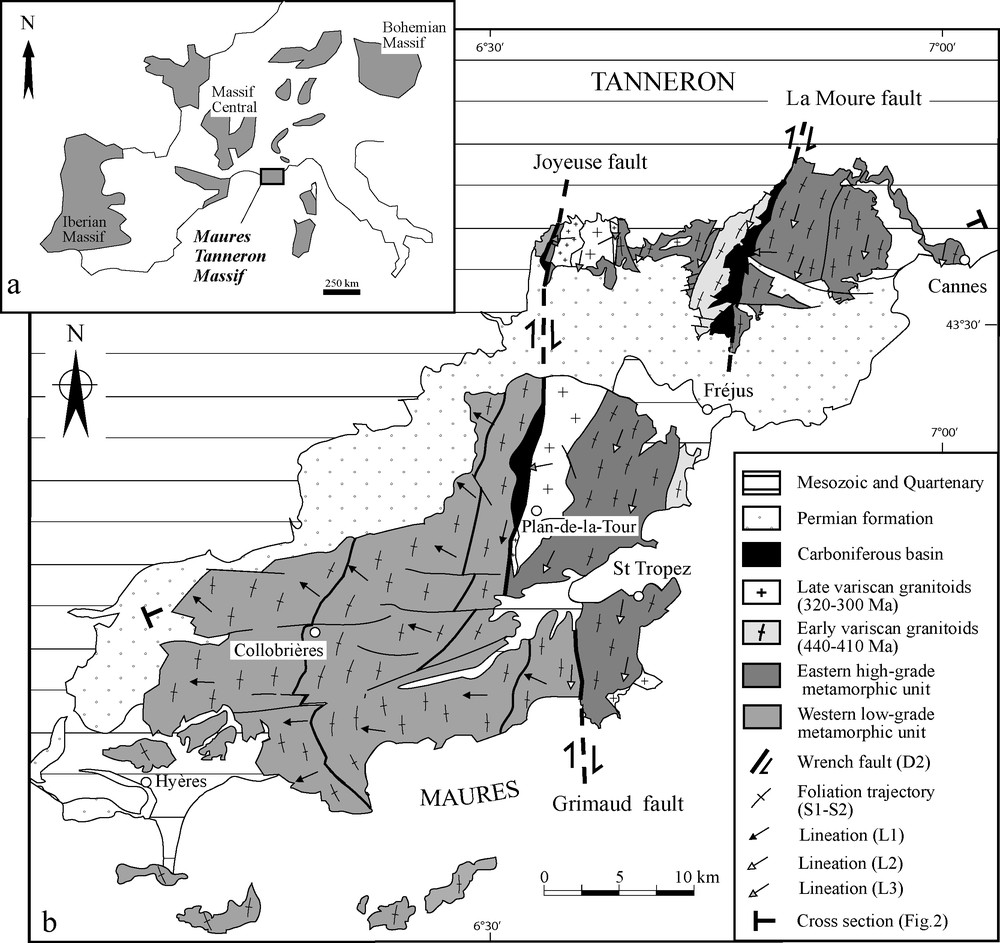
a: distribution of Pre-mesozoic units in Europe; b: simplified geological map of the Maures-Tanneron massif (MTM), modified from [8], with location of the geological cross-section of Fig. 2. Two main domains representing different crustal levels are distinguished: a western low-grade metamorphic unit separated from an eastern high-grade metamorphic unit by the Joyeuse-Grimaud strike-slip faults. The MTM is characterized by submeridian-trending major faults and fold structures. Directions of movement recorded by linear fabrics (D1–D2) have different orientations in the western and eastern compartment.
Fig. 1. a : localisation des unités ante-Mésozoïque d’Europe ; b : carte simplifiée du massif des Maures-Tanneron (MTM), modifiée d’après [8], avec la localisation des coupes géologiques de la Fig. 2. On distingue deux grands domaines correspondant à des niveaux crustaux différents : une unité métamorphique occidentale de bas-degré, qui est séparée d’une unité métamorphique orientale de haut-grade par la faille décrochante de Joyeuse-Grimaud. Dans l’ensemble du MTM, les plis et les failles ont une orientation subméridienne. Les directions de transport enregistrées par les fabriques linéaires (D1–D2) présentent des orientations différentes dans le compartiment occidental et le compartiment oriental.
The aim of this paper is to present a synthesis of new structural, petrological and geochronological data from the MTM (Fig. 1) and to compare them with those reported from Sardinia, Corsica and in the External Crystalline Massifs of the western Alps. Finally, a model of the tectonic evolution of the southeastern part of the Variscan belt is proposed.
2 The Maures-Tanneron Massif
Located near the Mediterranean Sea, the MTM is a segment of Paleozoic crust, mainly composed of high-grade metamorphic rocks intruded by Carboniferous granitoids [1,8]. The MTM is crosscut by an east–west trending Permian basin (Fig. 1).
2.1 Structural pattern
The major faults and fold structures and ductile fabrics of the MTM display a submeridian trend (Figs. 1 and 2). Regarding the metamorphic grade of rocks, the MTM is subdivided into two main domains, representing different crustal levels separated by the Joyeuse-Grimaud fault:
- • the eastern domain is representative of a deep-crustal level, marked by amphibolite facies metamorphism, associated with pervasive melting. This domain exhibits migmatites crosscut by several generations of synorogenic granitic intrusions;
- • the western domain preserves a prograde Barrovian metamorphic sequence, ranging from slates, chlorite-muscovite schists in the west to staurolite, kyanite and sillimanite bearing schists in the east. These show, in places, scattered signs of partial melting close to the Joyeuse-Grimaud fault. We therefore interpret the prograde sequence as reflecting crustal burial and subsequent tilting of the crustal column.
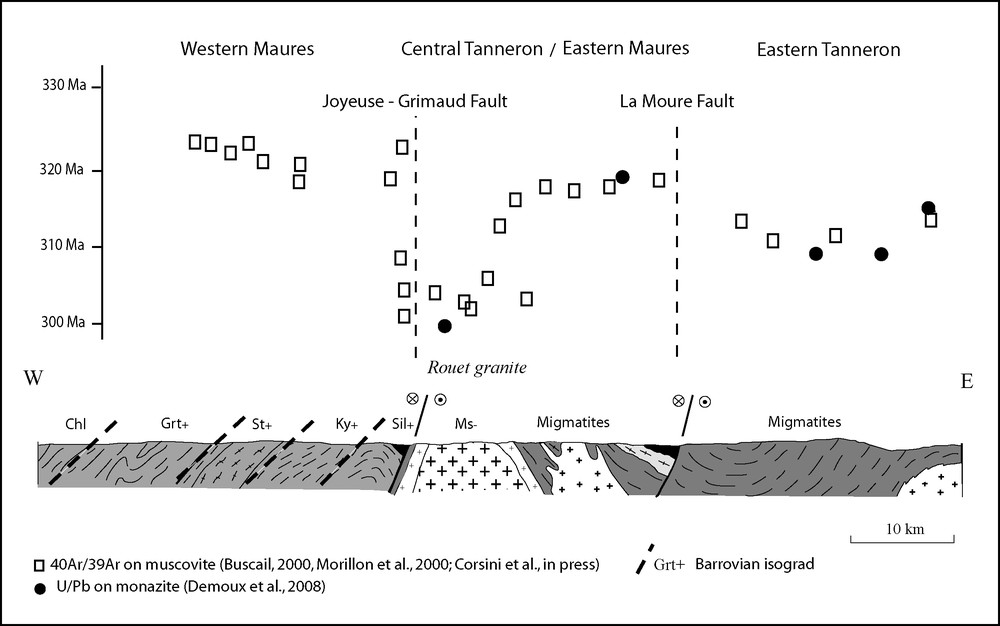
Schematic cross-section and ages distribution across the Maures-Tanneron massif (MTM) showing the three different stages of exhumation of individual blocks, limited by strike-slip zones, location on Fig. 1. The distribution of 40Ar/39Ar ages on muscovites along the transect reveal a heterogeneous cooling path of the lower crust during the Late Carboniferous from ca. 325 to ca. 315 Ma. Three different stages of exhumation of individual crustal blocks are controlled by major strike-slip faults. In the central part of the MTM, the ages decrease from 318 Ma to 303 Ma approaching the Rouet granite. This age distribution can be explained by the occurrence of a thermal structure associated with the magmatic complex.
Fig. 2. Coupe géologique schématique et répartition des âges à travers le massif des Maures-Tanneron (MTM), localisation sur la Fig. 1. La distribution des âges 40Ar/39Ar sur muscovites le long de la coupe montre un refroidissement hétérogène de la croûte inférieure au cours de la fin du Carbonifère, entre environ 325 et 315 Ma. On distingue trois stades différents d’exhumation des blocs crustaux, contrôlés par les grandes failles décrochantes. Dans la partie centrale du MTM, les âges diminuent de 318 Ma à 303 Ma à l’approche du granite du Rouet. Cette répartition des âges peut être expliquée par l’apparition d’une structure thermique associée au complexe magmatique.
Three main phases of deformation are identified. A first phase of ductile deformation (D1) is characterised by WNW-directed thrusting, parallel to a strong stretching and mineral lineation in the western domain. These structures have been preserved due to a low degree of later overprint in this area. In the eastern domain, ductile structures related to the D1 deformational phase are not preserved and it is assumed that they are entirely transposed by a deformation (D2) characterised by isoclinal and sheath folds, with a penetrative flat lying foliation S2. The S2 foliation bears a north–south trending L1W horizontal stretching and mineral lineation. Kinematic indicators suggest a top-to-the-south sense of shear. The phase D2 has evolved into orogen-parallel shearing along north–south trending, ductile dextral strike-slip shear zones. These zones of intense deformation are connected to the development of large-scale concentric folds and axial planar cleavage. During this transcurrent phase, two major faults (Joyeuse-Grimaud and La Moure faults) separated the massif into crustal-scale blocks with different exhumation histories [7,30]. The last phase of deformation (D3) is characterised by the growth of crustal anticlines and peraluminous granite (Plan-de-la-Tour–Rouet) and tonalite (Prignonet-Reverdit) intrusions. The Joyeuse-Grimaud and La Moure faults bound the eastern side of dissymmetrical Carboniferous basins, which are subvertical along the fault zone, while the detrital material lies unconformably with low angle on the basin's western side. Normal shearing-related fabrics are developed along limbs of crustal-scale antiforms and on the basins margins [7,11]. It is suggested that this shearing represents local accommodation structures reflecting vertical, probably diapiric, uplift of buoyant partially molten lower crust [7,34]. Simultaneous growth of marginal synclines occurred during and after basin infill. Therefore, during this late Variscan phase, often interpreted as a result of widespread extensional regime in the centre of the Variscan belt, the MTM exhibits ongoing east–west shortening, contemporaneous with strike-slip displacements along transcrustal faults.
2.2 Metamorphic and magmatic evolutions
The metamorphic evolution of the massif includes a HP–LT phase represented by eclogite relicts, largely overprinted by a MP–MT phase. This last is represented by Barrovian metamorphism in surrounding micaschists and migmatites, containing amphibolite lenses [1,8]. Geochronological investigations in the MTM suggest that the high-pressure metamorphism occurred at ca. 430 Ma (U-Pb zircon in eclogite [31]; U-Pb apatite in orthogneiss [11]), which is also in agreement with HP-subduction related metamorphism ages in the French Massif Central [27]. The metamorphic evolution of the MTM, related to the Variscan collisional event, is started by MP–MT Barrovian regional metamorphism that developed during the nappe-piling process and which occurred between 350 and 330 Ma (U-Pb monazite in migmatitic orthogneiss [11]; Rb-Sr whole rock [26]; U-Pb zircon on intrusives [31]). This event is followed by LP–HT regional metamorphism associated with the growth of migmatitic domes in the eastern part of the MTM. As in most of the Variscan belt, the uplift of lower-crustal levels is ascribed to thermal relaxation and reequilibration of isostatic potential following crustal thickening [24].
However, the most specific tectonometamorphic phenomenon observed here is the vertical and passive amplification of antiforms typical of diapiric growth of the migmatitic lower crust, which always occurs in the general context of east–west shortening [34]. Heat and magma transfer were amplified by crustal scale shear zones – the Joyeuse-Grimaud and La Moure faults that are believed to have controlled the late metamorphic evolution. The shearing must have been transpressive and highly partitioned, as the high-grade dome structures are found along the fault trend, in association with synconvergent tonalitic magmatism. This late event is dated by 40Ar/39Ar cooling ages on amphiboles, biotites and muscovites [2,7,30], which highlight the presence of three crustal blocks and indicate a distinct cooling history from 330 to 300 Ma. The distribution of cooling ages in three domains limited by crustal faults reflects heterogeneous exhumation controlled by the major ductile faults and the propagation of exhumation towards the east (Fig. 2). The western dip of the S1 foliation and the distribution of metamorphic isograds in the western unit (Fig. 2) are ascribed to an overall tilting of the upper-middle crust during D2 and possibly also D3. This is corroborated by contemporaneous exhumation of the orogenic lower crust in the adjacent eastern unit (Fig. 2).
Several magmatic events have been recognized and an evolutionary model is proposed, which fits magmatism into the general tectonic template. An early magmatic event at 440 to 410 Ma [11], related to the Silurian subduction stage, is characterized by the emplacement of granodioritic melts. It is followed by the intrusion of anatectic granitoids around 340 Ma [31] corresponding to crustal melting during the thickening stage. This event is replaced by an incipient anatectic melting and emplacement of leucogranites at 320 to 315 Ma, related to an active tectonic exhumation and subsequent isothermal decompression following a phase of crustal thickening [7,11]. Finally syn- to late-kinematic granites, such as the Plan-de-la-Tour (Maures massif) and the Rouet (Tanneron massif) granites, are related to a dextral strike-slip shear zone at ca. 300 Ma [7,11]. This later stage is contemporaneous with the deposition of Stephanian detrital sediments.
3 Correlation with Sardinia, Corsica and the external crystalline massifs of the western Alps – East Variscan Shear Zone
The structure and metamorphism of the MTM, described above, reflects the presence of shallower-crustal levels in the western domain compared with the eastern deeper crustal domain to the west of the Joyeuse-Grimaud Fault. As mentioned above, this main crustal-scale shear zone shows a transcurrent motion, with a vertical component that exhumed the migmatized lower crustal rocks of the eastern domain (Figs. 2 and 3).
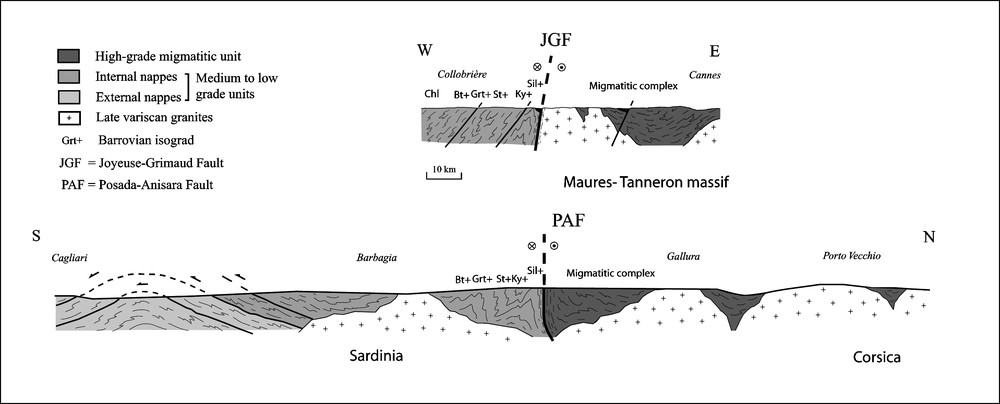
Comparison of cross-sections across Maures-Tanneron and Corsica-Sardinia, modified from [4,23]. This figure shows identical high-grade migmatitic zones structurally above the domain with Barrovian zonation indicating a continental underthrusting template. The Joyeuse-Grimaud fault, which separates the two crustal panels in the MTM, corresponds to the Posada-Anisara fault in Sardinia.
Fig. 3. Comparaison des coupes à travers Maures-Tanneron et Corse-Sardaigne, modifiée d’après [4,23]. Les deux coupes montrent de manière identique l’exitence d’une zone migmatitique de haut grade située au-dessus d’un domaine de métamorphisme barrovian, caractéristique d’un sous-charriage continental. La faille de Joyeuse-Grimaud, qui sépare ces deux panneaux crustaux dans le MTM, correspond à la faille de Posada-Anisara en Sardaigne.
This crustal structure is fundamentally similar to that currently reported from Sardinia (Fig. 4), where a similar prograde metamorphic upper-crustal section is observed in the south, separated from intensely migmatized northern Sardinia by the Posada-Anisara strike-slip fault ([4,23] and references therein). If the Oligocene rotation of Sardinia (∼30°) is restored, the foliation strike and the orientation of the main faults in Sardinia and MTM are similar and can be correlated to each other. In Sardinia, geochronological dating indicates MP–MT metamorphism reflecting the end of thickening stage at 344 ± 7 Ma (whole rock Rb-Sr on migmatite [18] followed by retrograde metamorphism at 320–300 Ma [40Ar/39Ar on muscovite [12]]) and the emplacement of anatectic granitoids at ca. 290 to 310 Ma (whole rock Rb-Sr [10]). Tectonic exhumation was accommodated by dome-like km-scale antiforms and local normal faulting [5,15].
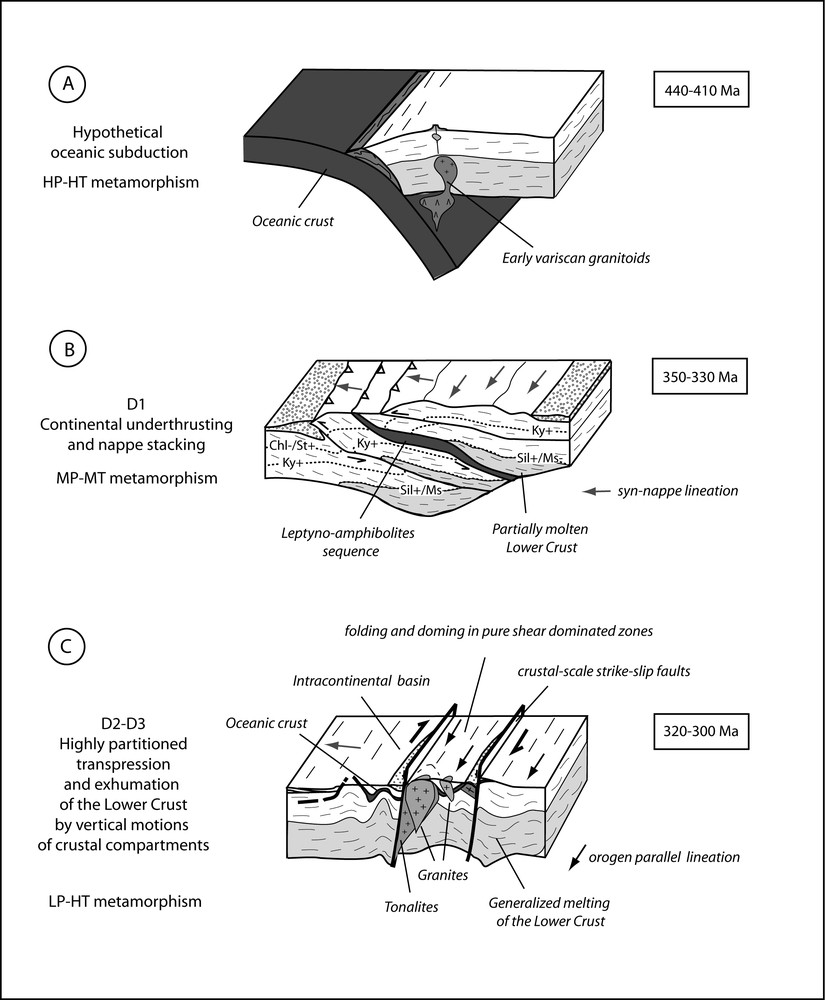
Geodynamic conceptual model of evolution of the Maures-Tanneron massif (MTM). The first stage shows hypothetical oceanic subduction, responsible for the formation of HP rocks and granodiorite calc-alkaline magmas. The second stage shows westward underthrusting of the continental crust beneath the partially molten upper plate indicating early continental convergence. The third stage shows the highly portioned transpressive stage with the development of pure shear- and simple shear-dominated zones.
Fig. 4. Modèle géodynamique conceptuel de l’évolution du massif des Maures-Tanneron (MTM). Le premier stade montre une subduction océanique hypothétique responsable de la formation des roches de HP et l’intrusion de magmas granodioritiques calcoalcalins. La deuxième étape montre le sous-charriage vers l’Est de la croûte continentale partiellement fondu sous la plaque supérieure, indiquant un début de convergence continentale. La troisième étape montre le développement de cisaillement pur et simple dominé par des plis et des zones de cisaillement, qui correspond à un contexte transpressif.
In Corsica, four postcollisional magmatic events were recognized using U-Pb zircon geochronology, at 345 Ma, 338 Ma, 305 Ma and 280 Ma, with a contribution of mantle-derived magmas triggering partial melting in the lower crust [33]. A transpressive dextral tectonic regime, under amphibolite-facies conditions, was accompanied with the emplacement of synkinematic high-K melts at 320 Ma and then overprinted by a final HT–LP metamorphism constrained at 312 to 308 Ma by monazite U-Th-Pb isotope dating [21].
In the External Massif of Argentera, two main domains are separated by the Bersézio ductile strike-slip shear zone, which bounds a Late Carboniferous basin and the Argentera granite. Geochronological studies have provided evidence for an HP/HT event at ca. 424 ± 4 Ma (U-Pb dating [32]), an amphibolite facies metamorphic event and related anatexis at 323 ± 12 Ma (U-Pb dating [35]), LP/HT metamorphism at ∼310 to 315 Ma [6] and emplacement of the Argentera granite during regional crustal melting at ca. 296 to 299 Ma [6,29]. However, the orientations of structures in the Argentera massif (N140°E) are clearly oblique to the north–south strike observed in the Maures massifs. This shift in strike is interpreted as anticlockwise tilting of the Argentera massif of ∼ 40° during the Alpine collisional phase. This is clearly indicated by a strong ductile reactivation of the main Bersézio fault during the Alpine phase (mainly at ca. 22 Ma) and by a greenschist facies foliation suggesting intense N140°E ductile to brittle deformation [6].
Towards the north, a similar segmentation has been proposed by Fernandez et al. [17] in the Pelvoux External Massif, which preserves a migmatitic eastern compartment and a low grade western segment comprising the Belledonne obducted sequence, with a similar strike of structures to the MTM. In the Belledonne Massif, eclogitic relics are dated at 390 ± 8 Ma (U-Pb on zircon [32]). Nappe stacking was contemporaneous with Mg–K granite emplacement along N30°E sinistral faults [28] and a transpressive tectonic setting was dated at 324 ± 12 Ma (K/Ar method on hornblende [22]). Widespread Stephanian extension associated with LP granulite and granite emplacement occurred between 305 and 295 Ma [22,28].
In the Mont-Blanc/Aiguilles-Rouges massifs, partial melting took place at 320 Ma (U-Pb monazite dating) and several peraluminous magmatic bodies, including the Mont-Blanc granite, were emplaced between 307 and 304 Ma [9]. Carboniferous magmatism is characterized by the association of crustal and mantle-derived melts emplaced in relation to major dextral shear zones [3,40].
All the foregoing indicates that the southeastern Variscan margin is characterized by a lithosphere-scale dextral and transpressive shear zone called here the East Variscan Shear Zone (EVSZ), which delineates the high-grade domain to the east and medium to low-grade units to the west. This shear zone is highly partitioned and associated with an important thermal event responsible for continental margin-scale melting at about 300 Ma.
4 Discussion and conclusion
The style and timing of thermal and tectonic events is quite similar in the different segments of the South European Variscan Belt (MTM, Corsica, Sardinia and Alpine External Crystalline Massifs) during Late Carboniferous times (Table 1). Progressive exhumation of the orogenic lower crust developed during dextral and highly partitioned transpressive shearing, with prominent orogen-parallel motion associated with crustal-scale strike-slip displacement and regional folding. Final exhumation of partially molten crust at ∼ 300 Ma was accompanied by numerous magmatic intrusions. Several processes are invoked for the formation and exhumation of partially molten deep-crustal levels along the South European transpressive shear zone that rims the eastern border of the Variscan Belt. The first event recorded in MTM is a hypothetical oceanic subduction, related to the development of Devonian HP rocks and intrusion of the Siluro-Devonian granodiorites (Fig. 4A). Even if the kinematics of the subduction process remain unclear, we favour the possibility of eastward movements as a precursor to continental underthrusting (Fig. 4B). This event was followed by eastward continental underthrusting (in recent coordinates) beneath an active continental margin, related to progressive thickening and melting of the upper-plate crust. The east–west stretching is not recognized to be preserved in the eastern high-grade unit and therefore we suppose the fabric here is associated with orogen-parallel flow in the partially molten upper-plate rocks (Fig. 4C). This indicates that if not crustal burial, then certainly early thrusting and exhumation of high-grade core occurred at a highly oblique setting. The second important process includes the relative vertical motions of crustal-scale blocks surrounded by transcurrent shear zones. This mechanism indicates an important degree of deformation partitioning into simple-shear and pure-shear domains in an oblique transpressive system [34]. In pure shear-dominated areas, the high-grade rocks were uplifted within crustal-scale folds showing passive amplification enhanced by gravity potential, as is typical for diapiric growth (Fig. 3). The absence of active amplification – that is, a crustal buckling mechanism is exemplified by the shape of crustal antiforms, sharp metamorphic gradients observed in the field, existence of ductile-to-brittle normal faulting along antiform limbs and the growth of intracontinental basins along marginal synforms. However, the presence of upright folds in sedimentary beds in adjacent Carboniferous basins, with the same strike of hinge zones as for the crustal-scale folds in the high-grade core of the orogen, indicates an important component of lateral shortening. Thus, the growth of folds occurred in a partitioned transpressive context during the Late Carboniferous in both infra- and supra-crustal levels.
Synthesis of geochronological data from different domains of the European eastern Variscan margin.
Tableau 1 Données géochronologiques synthétiques des différents domaines de la marge orientale varisque d’Europe.
Orogenic evolution | Age (Ma) | Major event | Sardinia | Corsica | Maures/Tanneron | Argentera | Pelvoux/Belledonne | Mont-Blanc/Aiguilles-Rouges | Bohemia |
Exhumation during transpression | 280 | Dome-like antiforms strike-slip faults and local normal faults | [5,15] | [21] | [7,34] | [39] | |||
Magmatism | [10] | [21,33] | [7,11,26] | [6,29] | [9,22] | [3,9,40] | [39] | ||
320 | HT-BP metamorphism | [12] | [21] | [2,7,30] | [35] | [9,22,28] | [39] | ||
Collision | 330 | MP–MT metamorphism | [18] | [33] | [31] | ||||
350 | |||||||||
Subduction | 410 | Magmatism | [34] | ||||||
440 | HP metamorphism | [31] | [32] | [9] |
Consequently, the structural evolution of the South European Variscan Belt is quite different from that of the French Central Massif, which is characterized by a main tectonic transport direction orthogonal to the supposed suture strike and principal nappe contacts of the belt. The South European Variscan Belt presents more similarities with the eastern border of the Bohemian massif. This is characterized by LP–HT regional metamorphism, combined with voluminous granitic intrusions in the Moldanubian domain and dextral strike-slip shearing parallel to the margin of the Brunia continental block during Late Carboniferous times [38]. However, in the Bohemian Massif, the high-grade Moldanubian zone (an equivalent of the eastern MTM) occurs to the West of the Barrovian metamorphic series of the Moravian zone to the east. Also, the kinematics of both high-grade domain and medium-grade continental shear zones are identical [20,39]. This indicates that even if the kinematics of the Moravian zone are similar, there is a fundamental difference in the position of the high-grade core compared with that of the EVSZ (Fig. 5). In addition, a partitioned-age sequence similar to that of EVSZ was proposed by Finger et al. [19] for dextral NW–SE shearing and associated magmatism along the Bavarian shear zone and partly also along the Elbe zone by Schulmann et al. [39]. Again the ages of magmatism and metamorphic evolution are in considerable agreement with the studied MTM segment of the EVSZ. Finally, sinistral transpressive (NE–SW) shearing of the identical age as reported in the MTM is also documented by Edel et al. [14] in the area of the Upper Rhine Transcurrent Shear Zone (Fig. 5). Here, the metamorphic ages, the character of magmatism and the north east transfer of the high-grade core parallel to the low-grade continental margins show close agreement with our studied area [36,37].
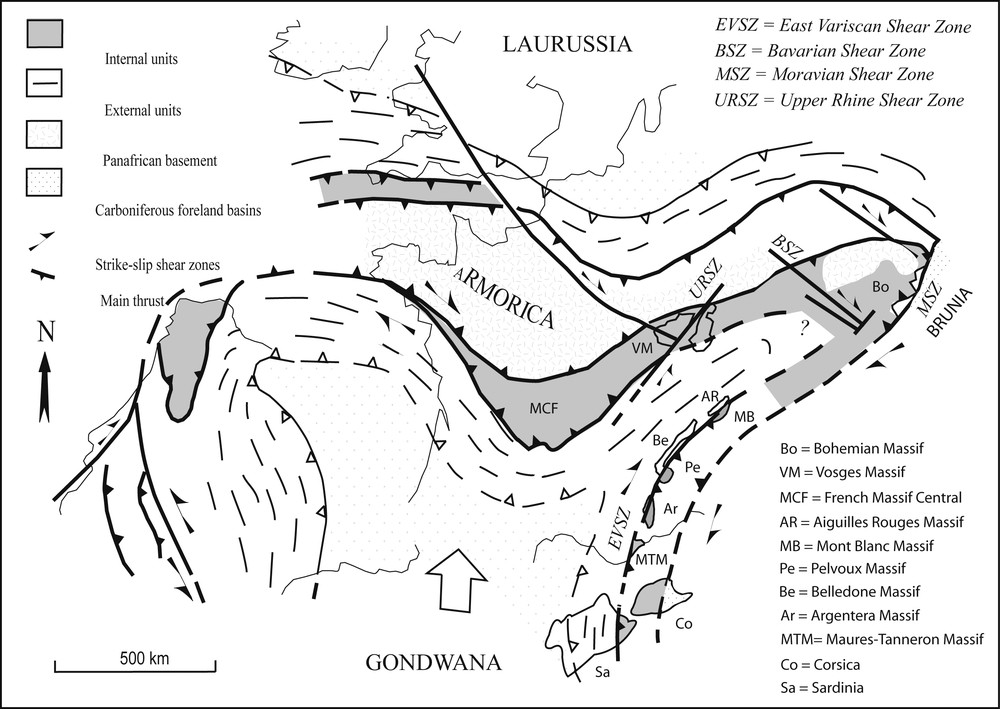
Structural sketch map of the Southern European Varican belt, modified from [27]. This figure indicates transcurrent 310 to 290 Ma shear zones in the Eastern Variscides. Note that the Moravian shear zone and East Variscan Shear Zone (EVSZ) show similar kinematics, but opposite polarity of high-grade to medium-grade domains. Also, note that the kinematics of the Moravian shear zone and the kinematics of the MTM barrovian domain are orthogonal.
Fig. 5. Carte structurale schématique de la Chaîne varisque du Sud de l’Europe, modifiée d’après [27]. Cette figure montre l’existence de grandes zones de cisaillement transcurrentes entre 310 et 290 Ma dans la partie orientale de la Chaîne varisque. Notez que la zone de cisaillement de Moravie et la zone de cisaillement de l’Est Varisque présentent la même cinématique, mais une polarité inverse des domaines de haut grade à moyen grade. Notez également que la cinématique de la zone cisaillée de Moravie et celle du Domaine barrovien MTM sont orthogonales.
In conclusion, we propose that, during the late evolution of the South European Variscan Belt, exhumation of the lower crust is ascribed to a still-surviving synconvergent transpressive context, coeval with regional folding, crustal-scale ductile strike-slip shearing and exhumation of deep orogenic-lower crust in the core of antiformal structures enhanced by gravity potential energy. This kind of tectonic template is reported along several dextral shear zones across the Variscan belt, indicating that the Late Carboniferous period was a time of important heterogeneous deformation of continental margins. In the case of the EVSZ and using the model of Matte [27], the narrow wrench belt was formed by transpressional movements at the border of the colliding Gondwanian continental block (Fig. 5). In contrast, the Moravian, Bavarian, Elbe and Upper Rhine Shear Zones may represent intra-orogenic shear zones induced by lateral displacement of the main continental mass to the north. More research is needed to further tune and develop this Philippe Matte seminal concept of evolution of the Variscan orogenic system and connect the South European Variscan evolution to that of the eastern Variscan continuation in the Bohemian Massif.
Acknowledgments
This publication is a contribution of UMR 6526 Géosciences Azur. We are grateful to K. Schulmann, J.-M. Lardeaux and M. Faure, who have significantly helped in improving this manuscript. The ANR project funding LFO in orogens is acknowledged.