1 Introduction
Karstic aquifers are of particular interest and importance to Mediterranean countries, because they contain most of their groundwater resources. Their structure and dynamics are complex [5], because they modify various hydrodynamic features and flow conditions. Usually, the description and understanding of the functioning of karstic systems requires the use of several indirect methods, based on the interpretation of hydrodynamics and hydrochemical time series, which are measured at the system's outlets. These methods enable the degree of karstification and the volume of groundwater resources to be assessed in the saturated zone of the aquifer. However, they cannot be used to determine the structure of the system or to locate underground drains. In order to gain further knowledge of the geometry and structure of various parts of a karstic aquifer (epikarst, vadose zone, saturated zone, drains, caves and fissured matrix), direct methods such as geophysical studies must be used. The aim of such studies is to locate areas which are:
- (i) more favourable to the implementation of drilling and/or;
- (ii) which present a significant pollution risk for the groundwater.
For several decades, numerous geophysical studies have been carried out in order to investigate karstic structures. Geophysical methods (electrical resistivity imaging [ERI], georadar, magnetic resonance soundings [MRS], electromagnetic very low frequency [VLF], seismic) have been used with success to localise cavities [2,18,23], and to estimate the mean azimuth of the fracturing [6,12,22]. In every case, the results produced by ground-based geophysics have been useful in localising structural features (faults, fractures, altered areas, etc.) related to karstic systems, rather than to determine the position of saturated or unsaturated karstic conduits.
Initial results obtained with MRS [23] indicate that this method can allow groundwater resources to be detected and vertically located, at different karstic levels, down to a depth of investigation of the order of 20–30 m.
In the framework of the Waterscan ECosphère COntinentale, Programme National de Recherche en Hydrologie (ECCO/PNRH) project, a multi-method geophysical survey was carried out at the Poumeyssen test site in France. This site was chosen because it is located far from any potential electromagnetic interference (high-voltage lines, industry, human activities, etc.). The scope of the study was to test the MRS method, and to combine the results with other geophysical measurements in order to establish a methodology suitable for the study of karstic systems.
2 Hydrogeological context
The Poumeyssen test site is located on the plateau of Causse de Gramat (Lot administrative region, South-West France). This plateau has been built up by Callovian limestone, and has well-developed karstic features (caves, sinkholes and resurgences [4]). The Poumeyssen shaft provides direct contact with one of the main drains of the Ouysse karstic system, which corresponds to an area of approximately 540 km2, of which 360 km2 are covered by Causse de Gramat carbonate rocks. The remaining 180 km2 are covered by more impermeable rocks with surface drainage relying on infiltration through several sinkholes, whenever the river flows over the carbonate rocks. During the course of its underground drainage, covering a distance of more than 25 km, this water is mixed with karstic water, and is then discharged by several springs, one of which is that of Cabouy (Fig. 1a). The Poumeyssen shaft intercepts the main karstic conduit, 600 m upstream from this spring. It is a wide, shallow, probably unique, water-filled conduit located in compact limestone, which has been previously mapped by cave divers (Fig. 1b). The conduit has a diameter of 5 to 9 m (i.e., its section varies between 20 and 65 m2), and is located 10 to 15 m below the surface at the test site. The water's resistivity varies between 15 and 24 Ω m (i.e., its conductivity lies in the range between 670 and 420 μS/cm). The hydraulic head is far above the main conduit. The water flows from east to west. The Poumeyssen shaft allows a direct vertical connection to this main conduit. The water table in the shaft is linked to the discharge of the Cabouy spring. As the karstic structure is very heterogeneous, this water table is not representative of the entire structure.
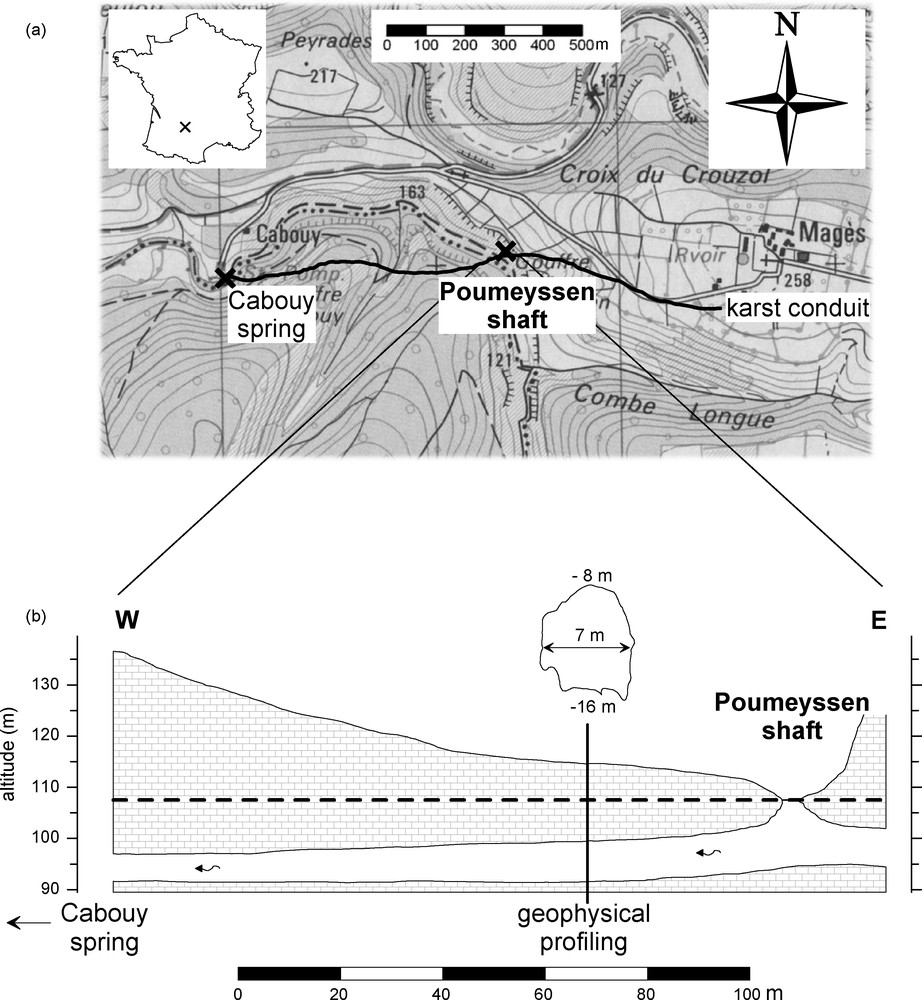
(a) Location map of the studied site extracted from 1:25 000 IGN no. 2137 O topographic map, (b) conduit cross-section given by cave divers.
(a) Plan de localisation du site d’étude extrait de la carte topographique 1:25 000 IGN no 2137 O, (b) coupe du conduit donnée par les spéléologues.
3 Geophysical methodology
The “mise-à-la-masse” technique, initially designed for the mining industry [20], allows a conductive linear structure to be followed. At the Poumeyssen test site, the conductive target was the karstic conduit. A pole-pole array was used: an injection electrode was placed directly inside the conductive target (i.e., in the shaft), and a potential electrode was moved over the ground, in such a manner as to generate an approximately 5 m × 5 m mesh; two other electrodes (one injection and one potential) were located at infinity. Appropriate representation of the measured potential enabled the following characteristics of the conductive medium to be determined:
- (i) extension;
- (ii) dip;
- (iii) direction, and;
- (iv) continuity.
The injected current was in the range of 0.1 A. The “mise-à-la-masse” data presented in this study were collected using a Syscal R2 resistivity meter (IRIS instruments) with a DC/DC convertor supplied with a 12 V battery.
MRS is based on the measurement of the electromagnetic signal that is generated by the processing of the nuclei of the hydrogen atoms in groundwater molecules after an electromagnetic excitation at a specific frequency (i.e., resonance frequency). This method is directly sensitive to groundwater and allows estimates of the vertical distribution of water content [16]. Although MRS was initially developed for 1D targets, in the work described here, a profile was determined using eleven soundings, and was then interpreted with a 2D algorithm. For each sounding, square coincident transmitter/receiver loops (25 m in width) and the Numisplus device (IRIS instruments) were used.
An ERI can be useful for the measurement of electrical resistivity contrasts, and is thus able to locate fractured zones. In 2D, the technique [13] consists in using a multi-electrode system along a profile and carrying out a combination of electrical quadrupole measurements following a predefined geometry to obtain a 2D geoelectrical section. The exciting current (between 1 and 50 mA) is injected between two electrodes, and two other electrodes measure the electrical potential difference (between 0.01 and 1 V). Different arrays and spacings with 72 electrodes were used: a Wenner–Schlumberger array with an electrode distance of 1, 2 and 5 m; a pole-dipole array and a dipole-dipole array, both with electrode distances of 1 and 2 m. The ERI was carried out using a Syscal Pro-resistivity meter (IRIS instruments). The different arrays were merged (to take advantage of each array [10] and to better constrain the results) and the resulting data were inverted with the Res2dinv software package [17].
High-resolution seismic methods were used for the study of the karstic area, because of their advantageous penetration depth and spatial resolution (see a review in [15]). The seismic signature of a void in a karstified medium, measured by compression (P) waves, is highly variable, due to the influence of diffraction patterns, local attenuation, perturbations caused by reflecting material deeper than the target, and the P-wave low velocity anomaly. A conduit can thus be more difficult to detect when it is water-filled, due to a lower acoustic impedance contrast. Diving-wave tomography and reflection are better suited to a karstic setting than standard refraction techniques, because of the presence of strong lateral heterogeneities in the medium. If the spacing of shots is sufficiently close throughout the recording, the same data can be used for reflection processing and tomographic inversion. In the present study, a seismic line was recorded by a vendor in parallel with the ERI profile. The seismic source was a hammer striking a metallic plate; stacking was used to enhance signal to noise ratio. The profile was acquired by using the Summit C device (DMT) and moving the source along a fixed 186 receivers spread centred above the conduit. Shot and receiver spacings were 3 and 1 m, respectively. The first breaks inversion was carried out with the Rayfract software package implementing wavepath eikonal traveltime inversion [21].
4 Results and discussions
The results of the “mise-à-la-masse” map shown in Fig. 2 reveal a generally strong dependence on:
- (i) distance from the Poumeyssen shaft electrode injection point: the potential is inversely proportional to the distance of the two closed pole-pole electrodes and;
- (ii) the complex topography of the site (location with vertical slope, quick orientation change, etc.).
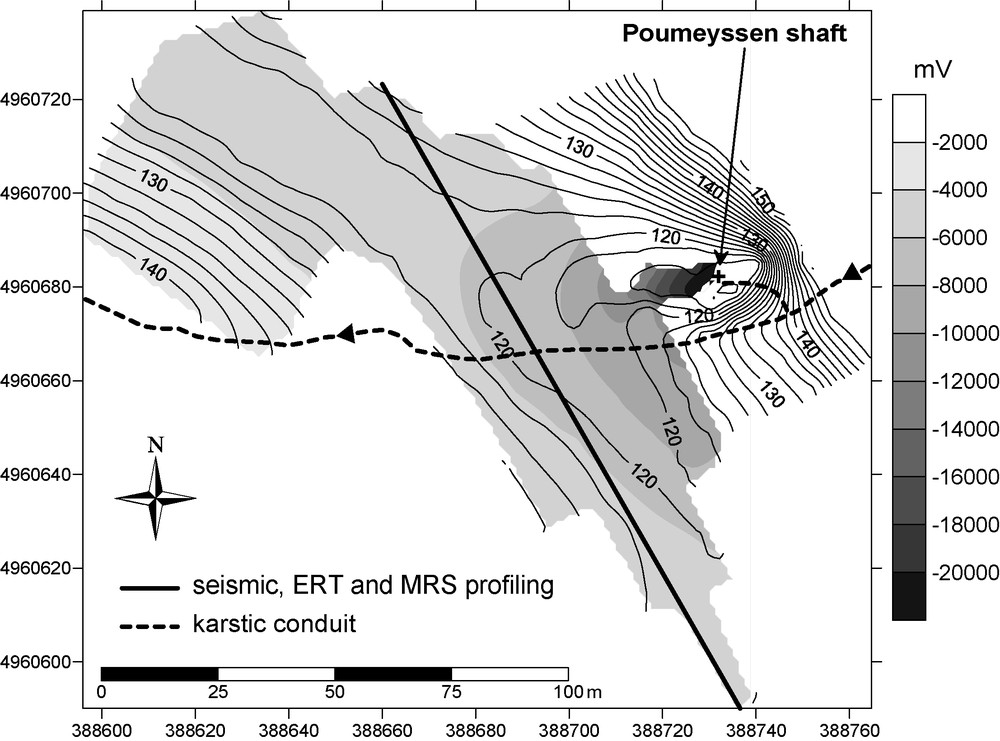
“Mise-à-la-masse” map (grey shading), contours represent altitude (in meters). The dotted line corresponds to conduit location. The water flow in the conduit goes from east to west. The solid line corresponds to geophysical profile.
Carte de potentiel (en dégradé de gris) obtenue avec la mise-à-la-masse, les courbes d’isovaleurs représentent les altitudes (en mètres). Le trait en pointillé correspond au tracé du conduit. L’écoulement se fait dans le conduit de l’est vers l’ouest. Le trait plein correspond au profil géophysique.
The complex topography does not allow quantitative modelling of the data [19]. However, a simple structure (conductive conduit in a homogeneous resistive medium) was modelled [9]. This modelling showed that, in a flat area, it should be straightforward to locate the conduit rather than the one described in the study area.
2D inversion of the MRS (Fig. 3) has demonstrated its efficiency in locating and estimating the geometry of a karstic conduit [7,11]. For this inversion the electrical resistivity obtained by ERI was used. Although the MRS signature is not sensitive to the conduit shape, it varies as a function of its volume (or the conduit section). The uncertainty of the depth given by MRS is ±3 m [11], while for the true depth measured by cave divers, it is ±1 m. The uncertainty of the lateral location given by MRS is ±15 m [11], while the true lateral location is known at ±0.5 m. The conduit location was determined with the following errors:
- (i) the depth of the top of the conduit is less than 2 m (thus coherent with depth uncertainty);
- (ii) the lateral position of the centre is less than 1 m;
- (iii) the conduit section has the same level of precision as that given by cave divers.
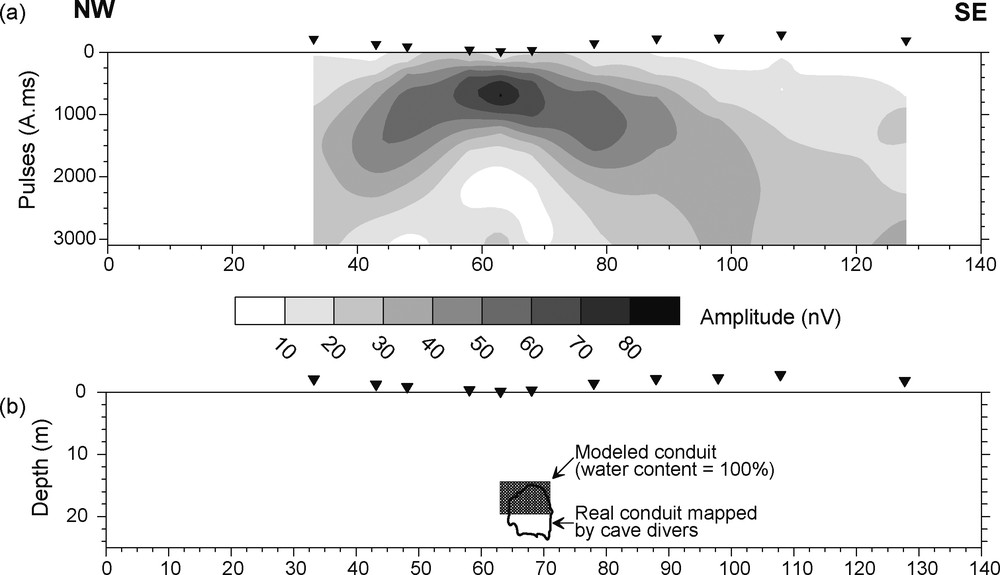
Magnetic resonance sounding (MRS) profiling: (a) amplitude cross-section, (b) comparison between MRS inversion section and the karstic conduit section drawn by cave divers. The conduit is considered here as a 2D parallelepiped structure with 100% of water content.
Profil résonance magnétique des protons (RMP) : (a) coupe en amplitude, (b) comparaison entre l’inversion RMP et la section du conduit karstique donnée par les spéléologues. Le conduit est représenté comme un parallélépipède d’extension latérale « infinie » avec 100 % de teneur en eau.
It has also been shown that it is important to take the relative orientation, between the MRS profile and the conduit, into account.
The ERI (Fig. 4) reveals the presence of a conductive superficial layer inside the limestone, whose resistivity varies between 400 and 1000 Ω m. This conductive area corresponds to altered limestone and/or soil. At the centre of the profile, the thickness of this conductor increases, and it appears to be connected to a deeper structure. This suggests that the environment in which the conduit has developed is not as homogeneous as one might think. The conductive structure is found to be well correlated with the location of the conduit (in the centre of the connective area lying between the conductive superficial layer and the deeper conductive structure).
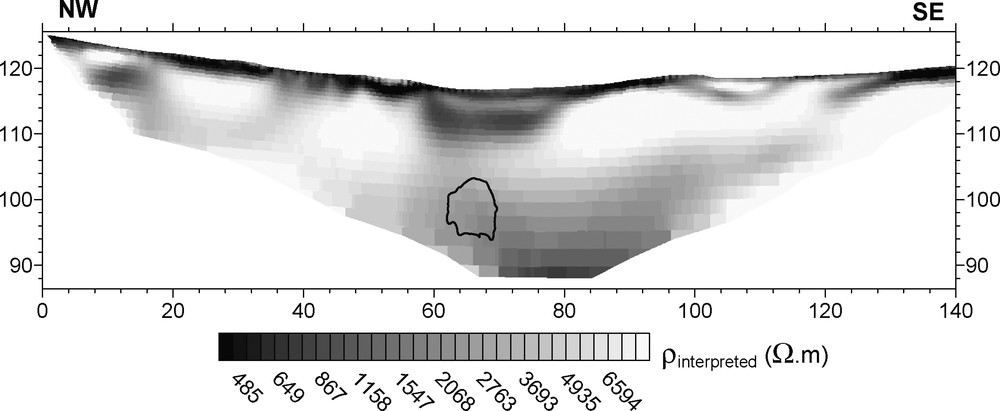
Electrical resistivity imaging (iteration no. 4, RMS error = 6.08%) with the karstic conduit section superimposed. During the inversion topography was used and resistivity contrast was limited.
Imagerie de résistivité électrique (4e itération, erreur RMS = 6,08 %) avec le contour du conduit positionné. La topographie a été prise en compte et le contraste de résistivité limité durant l’inversion.
As mentioned in the introduction, the seismic signature of the conduit can be of several types. In the Poumeyssen case, the conduit is at shallow depth and cannot be identified by a reflexion (we have interference with the source wavelet) on field records. However, when the shot is located away from the conduit, visual examination of records indicates that a wave field perturbation (amplification and ringing phenomena) occurs for the receivers above the exact location of the conduit (Fig. 5a). It is therefore expected that at least nearly horizontal travel paths (refracted or surface waves) can sense the conduit. The P-wave velocity model (Fig. 5b) resulting from tomographic inversion of the wave equation reveals a strong velocity gradient near the surface. The thickness of this gradient layer is highly variable and always less than 15 m, except in the zone where the conduit is vertically aligned. In fact, the 1500 m/s velocity contour (close to the velocity of waves in water) is characterised by a single sharp anomaly at this location. The width of this anomaly is in agreement with the width of the conduit, and it is interesting to note that it is vertically linked to the surface. This observation may suggest that the medium above the conduit is weakened and/or fractured.
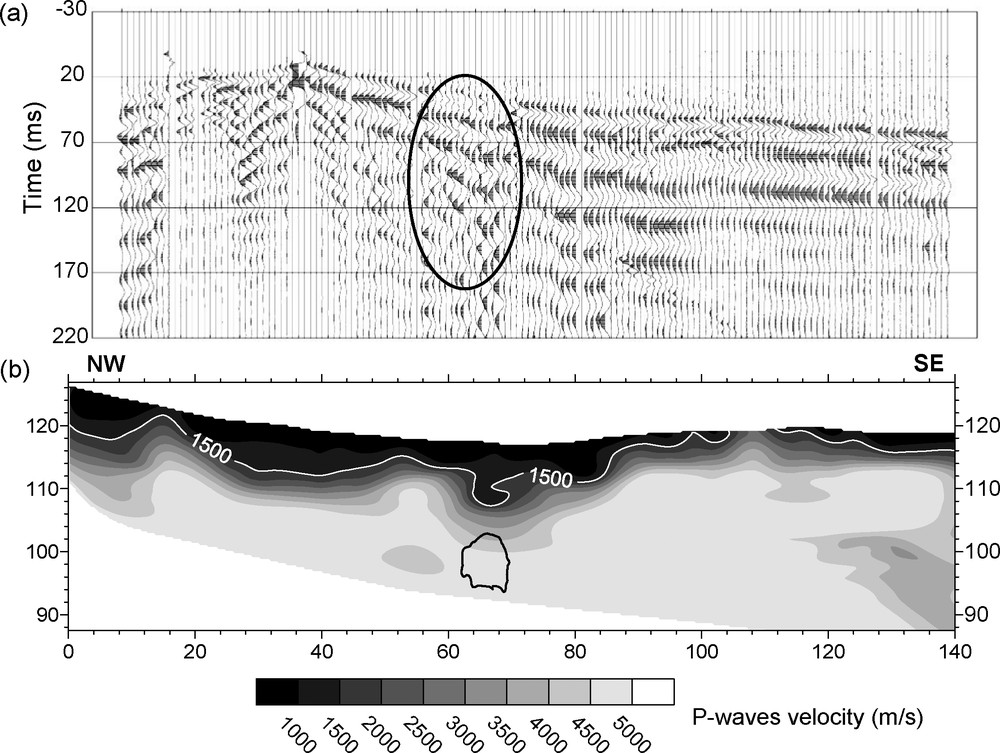
Seismic results: (a) interference of seismic waves with the conduit (amplification and ringing phenomena above the conduit), (b) P-wave velocity model with the karstic conduit section superimposed. The 1500 m/s velocity contour (close to the velocity of waves in water) is characterised by a single sharp anomaly; it suggests that the medium above the conduit is weakened and/or fractured.
Tomographie sismique : (a) interférences des ondes sismiques dues au conduit (amplification et phénomène de résonance au-dessus du conduit), (b) modèle de vitesse des ondes P, avec le contour du conduit positionné. L’isovaleur 1500 m/s (correspondant à la vitesse de propagation dans l’eau) présente un approfondissement, qui suggère que le milieu est altéré et/ou fracturée au-dessus du conduit.
It is tempting to consider some kind of joint inversion or interpretation of the measurements, taking into account the nature of the different physical measurements and their complementarities. In reality it is impossible to design a reliable workflow for several reasons. Some are related to the different resolutions (both horizontal and vertical) of the methods, which would necessitate complex down- or up-scalings, but the main difficulties are in the physics of the problem. Several cross-property relations have been developed between seismic velocities and electrical conductivities [8], using porosity prediction empirical laws or theoretical models as a bridge between elastic and electrical properties. Unfortunately this framework has been developed for clastics rocks, and when applied to carbonates it breaks down. One of the main reasons is the complexity of the porosity (double porosity) in limestone; it is now widely proven [1] that for a given porosity the different pore shapes and scales (intra-granular, vugs, micro cracks, etc.) have a strong influence on the velocities at the seismic scale. It is therefore impossible to use a reliable model between velocities and conductivities to attempt some kind of joint inversion. Concerning MRS and ERT, joint inversion should give better results, but in fact there are no tools developed as yet: the joint inversion needs to use Archie's law [3] not valid here [14].
5 Conclusions
An electrical survey carried out at the Poumeyssen site reveals that the medium is not homogeneous. MRS gives an estimation of the volume of groundwater retained in a karstic aquifer, whereas seismic measurements show that the limestone host rock is not homogenous. The highly variable, low velocity layer near the surface represents the upper, most weathered part of the karst (epikarst?). Below this layer, the medium is more homogenous. In the present case, the conduit appears to be locally connected to this shallow layer by some kind of weakened structure.
Our survey confirms that it is difficulty to detect a conduit directly, using ground-based geophysical methods under “real” conditions. Several types of difficulties affected the electrical methods used:
- (i) the topography of the site is complex (the conduit appears to be located in a slight structural hollow);
- (ii) the conduit is not always located at the same depth (in the case of the present study, although it remains at the same altitude, the topographical surface is not flat);
- (iii) the resistivity contrast between the conduit and the host rock is not very clearly defined and;
- (iv) the presence of a superficially altered, conductive layer produces a significant signal.
In the case of seismic measurements, apart from the difficulties arising from near surface heterogeneities, as mentioned above, the main drawbacks are:
- (i) the lower acoustic impedance contrast when the conduit is filled (no air-water interface) and;
- (ii) the strong near-surface velocity gradient, which may reduce the depth of penetration by bending the seismic waves upwards.
Despite these difficulties, it was possible to accurately locate the Poumeyssen conduit using ground-based geophysical methods. The fact that the medium above the conduit exhibits anomalous electrical and elastic properties enhances the signature of the conduit itself. Although the nature of the weakened structure above the conduit could not be clearly established, the observed anomalies may indicate that the conduit is developing upwards, by dissolution. If it can be confirmed that this superficial anomaly is directly linked to the conduit, the most effective, combined methods for locating similar structures, over the same range of depths, would be seismic profiling, and subsequent MRS soundings on any low velocity anomalies.
Acknowledgements
The authors wish to thank the ECCO/PNRH programme for providing its support to this study.