1 Introduction
Thermal conductivity of mantle minerals controls the heat balance and the thermal evolution of the Earth. Periclase-wüstite solid solution, (Mg,Fe)O, has been examined as an important binary system to consider the mineralogy in the Earth's lower mantle, which constitutes more than half the Earth's volume. MgO periclase has been traditionally used as a proxy mineral for estimating lower mantle thermal conductivity (Brown, 1986; Manga and Jeanloz, 1997). Rocksalt (B1) type structure stabilizes in periclase up to several hundred gigapascals region (Coppari et al., 2013; McWilliams et al., 2012), and its thermal conductivity monotonically increases with increasing pressure and decreases with increasing temperature increment (e.g., Dalton et al., 2013; Imada et al., 2014; Manthilake et al., 2011). On the other hand, Fe1−xO wüstite shows various structural, magnetic, and insulator-metal transitions in the conditions relevant to the Earth's lower mantle (e.g., Fei and Mao, 1994; Fischer et al., 2011a; Kantor et al., 2004; Ohta et al., 2012; Ozawa et al., 2011; Yagi et al., 1985). Thermal conductivity of wüstite has only been measured at 1 bar (Akiyama et al., 1992), and thus the pressure response and the effect of such pressure-induced transitions on the conductivity of wüstite are unknown.
Ferropericlase, a Mg-rich member of (Mg,Fe)O, is considered to be the second most abundant mineral in the lower mantle, and its thermal conductivity strongly affects bulk lower mantle thermal conductivity despite its abundance being smaller than that of bridgmanite (Hsieh et al., 2018; Ohta et al., 2017). Magnesiowüstite, Fe-rich (Mg,Fe)O, possibly exists at the base of the mantle and could be the origin of abnormally low seismic wave velocity in regions called Ultra Low Velocity Zones (Wicks et al., 2017). Indeed, magnesiowüstite has been found in natural diamonds collected in the Juina province, Brazil, which could have originated from the lowermost mantle (Wirth et al., 2014). Moreover, experiments and thermodynamic modeling indicated that remnants of a crystallizing basal magma ocean could be evolving toward a magnesiowüstite-rich composition, which may still exist at the base of the mantle (Boukaré et al., 2015; Nomura et al., 2011). Strong iron impurity effect on lattice thermal conductivity (i.e. thermal conductivity by phonon propagation) of (Mg1−xFex)O with 0 < x ≤ 0.56 was confirmed by high-pressure experiments both with a large volume press (Manthilake et al., 2011) and a diamond anvil cell (DAC) (Goncharov et al., 2015; Hsieh et al., 2018; Ohta et al., 2017). However, there is no report on the conductivity of iron-rich (Mg1−xFex)O solid solutions, even at ambient conditions.
In this study, we examined the effects of iron content and pressure on lattice thermal conductivity of (Mg1−xFex)O solid solutions in a DAC. We measured the lattice thermal conductivity (κlatt) of wüstite up to 61 GPa and 300 K. The results show that the pressure dependence of κlatt of wüstite drastically changes, likely due to structural distortion from a B1 lattice. The κlatt of magnesiowüstite with five different compositions was also measured at 1 bar and 15 GPa and 300 K, which is lower than those of periclase and wüstite endmembers due to the phonon-impurity scattering effect.
2 Materials and methods
The samples used in this study were polycrystalline (Mg1−xFex)O (x = 0.40, 0.50, 0.80, 0.95, and 1.00) that have been used in the literature (Fujii et al., 2011, 2013; Ohta et al., 2014). Prior to our experiments, we confirmed by means of powder X-ray diffraction (XRD) measurements that all the samples were aggregates of B1-structured (Mg1−xFex)O single phase. The chemical composition of the wüstite sample was obtained as Fe0.98O from its lattice constant at ambient conditions (McCammon and Liu, 1984). DACs with 300-μm culet anvils were used for high-pressure generation. Gold was sputtered on both sides of the samples for thermal conductivity measurement. The sample was loaded into a sample chamber of the DAC together with the pressure medium, NaCl, and a single crystal of sapphire. Pressure was calculated from the wavelength of ruby fluorescence (Mao et al., 1978) or the Raman shift of diamond anvil (Akahama and Kawamura, 2004). Uncertainties of the methods are 5–10%.
Thermal conductivity (κ) is a function of thermal diffusivity (D), density (ρ), and isobaric heat capacity (CP): κ = D ρ CP. The thermal diffusivity of (Mg1−xFex)O was determined by means of the pulsed light heating thermoreflectance method in the DAC (Fig. 1). Details of the technique may be found in the literature (Ohta et al., 2017; Yagi et al., 2011). The reported equations of state of the (Mg1−xFex)O were used to correct sample thickness at high pressure, and to calculate the density and the isobaric heat capacity on the basis of the Debye approximation for each chemical composition (Fei et al., 2007; Fischer et al., 2011b; Jacobsen et al., 2005; Solomatova et al., 2016; Wicks et al., 2015; Zhuravlev et al., 2010).
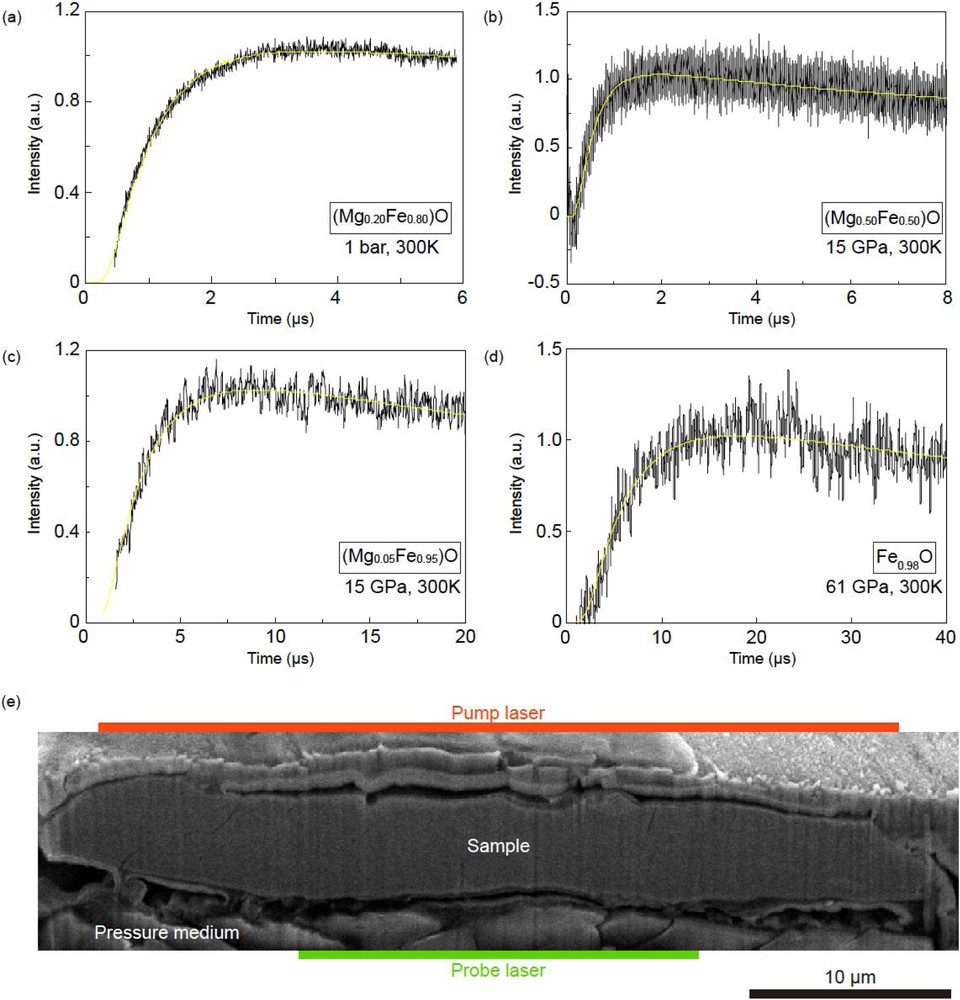
Representative transient temperature curves of (Mg0.20Fe0.80)O obtained at 1 bar (a), (Mg0.50Fe0.50)O at 15 GPa (b), (Mg0.05Fe0.95)O at 15 GPa (c), and Fe0.98O at 61 GPa (d). A scanning electron microprobe image of a cross-section of the Fe0.98O sample recovered after the thermoreflectance measurement (e). Red and green bars indicate the spot diameter of pump and probe lasers.
3 Results and discussion
3.1 Pressure response of lattice thermal conductivity of wüstite
We conducted ten separate sets of experiments to determine the κlatt of Fe0.98O from 6 GPa to 61 GPa at 300 K (Table 1 and Fig. 2). For comparison, the κlatt values of Fe0.95O wüstite at 1 bar (Akiyama et al., 1992) and MgO periclase up to 60 GPa (Dalton et al., 2013) are plotted together in Fig. 2. At ambient conditions, the κlatt of wüstite is about one order of magnitude lower than that of periclase. The κlatt of periclase monotonically increases with pressure. On the other hand, the pressure derivative of the κlatt of wüstite shows to be both positive and negative, which changes the sign at around 33 GPa. The value of the κlatt of Fe0.98O is 4.0 W/m/K at 61 GPa.
Experimental pressures, sample thickness, and thermal diffusivity and conductivity of Fe0.98O.
Pressure (GPa) | Sample thicknessa (μm) | Thermal diffusivity (mm2/s) | Thermal conductivity (W/m/K) |
6.1 (3) | 4.50 (37) | 1.69 (31) | 6.71(122) |
10.2 (5) | 4.46 (37) | 2.06 (41) | 8.30 (164) |
14.9 (7) | 17.02 (88) | 4.12 (43) | 16.82 (174) |
18.9 (9) | 3.08 (30) | 6.31 (53) | 24.90 (21) |
32.8 (33) | 6.96 (25) | 4.52 (40) | 25.65 (228) |
42.1 (42) | 8.25 (20) | 3.00 (18) | 17.05 (102) |
49.2 (49) | 8.65 (70) | 2.11 (37) | 12.40 (216) |
60.6 (61) | 4.77 (26) | 0.68 (7) | 3.97 (43) |
a After correction for pressure effect using P-V-T EOS of FeO (Jacobsen et al., 2005; Fischer et al., 2011a).
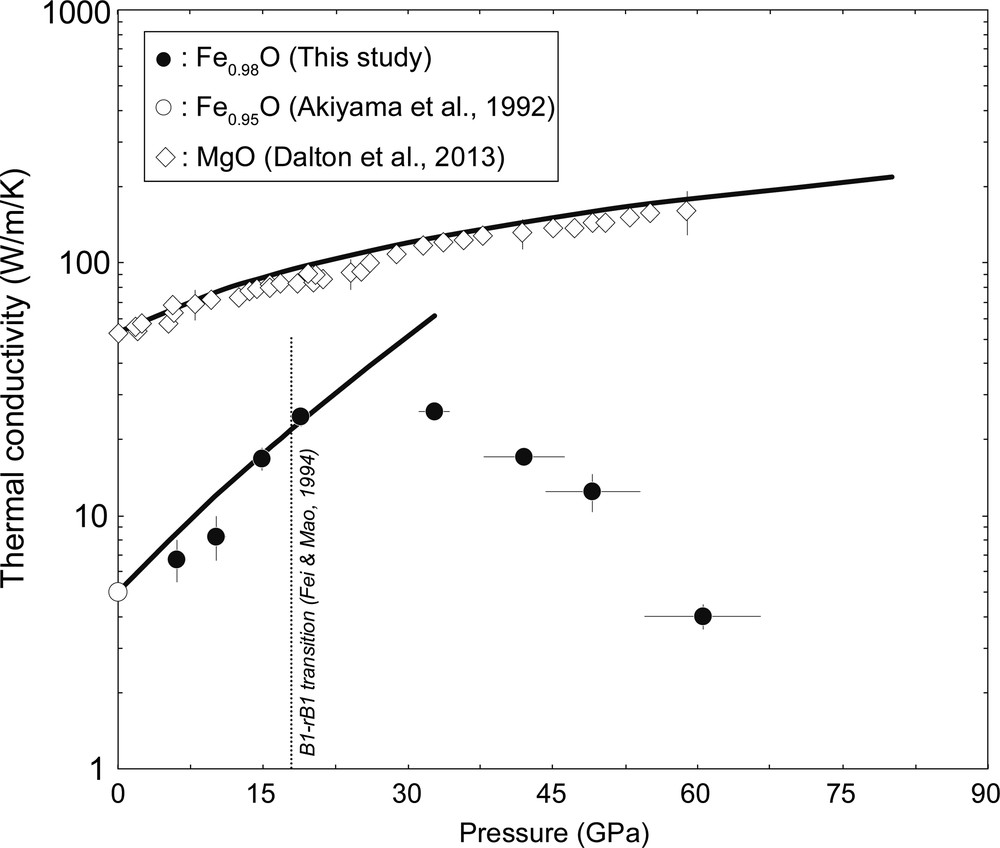
κlatt values of wüstite and periclase as a function of pressure at 300 K. Black circles, Fe0.98O (this study); white circle, Fe0.95O (Akiyama et al., 1992); diamonds, MgO (Dalton et al., 2013). Broken line, the phase transition pressure from B1 to rB1 structure of Fe0.98O under quasi-hydrostatic conditions (Fei and Mao, 1994). Black lines indicate the pressure dependence of κlatt of Fe0.98O and MgO based on the damped harmonic oscillator–phonon gas model (Hofmeister, 1999) with reported thermoelastic properties of these minerals (Tange et al., 2009; Yagi et al., 1985).
An anomalous pressure dependence of κlatt was observed in (Mg0.81Fe0.19)O ferropericlase and (Mg0.44Fe0.56)O magnesiowüstite, which should be due to the spin crossover of iron in the mineral (Hsieh et al., 2018; Ohta et al., 2017). However, the decrease of κlatt of Fe0.98O observed in this study is separate from the spin crossover because it has been confirmed that high-spin FeO was preserved up to 140 GPa and 300 K on the basis of X-ray emission spectroscopy measurements (Badro et al., 1999; Mattila et al., 2007). Alternatively, such an abnormal pressure response from the κlatt of Fe0.98O is likely induced by the rhombohedral distortion of B1 structured wüstite at high pressure. Previous powder XRD studies on wüstite reported that the B1 to rhombohedral B1 (rB1) phase transition occurred roughly at 16 GPa and 300 K under quasi-hydrostatic conditions similar to those of the present study (Fei and Mao, 1994; Yagi et al., 1985). The rhombohedral distortion in wüstite has been interpreted as the consequence of an increase of the exchange interaction of iron ions in the antiferromagnetic ordering, although the observed magnetic transition pressure for Fe1−xO was not completely in accord with the B1–rB1 transition pressure (Kantor et al., 2004). The κlatt value tends to be lower for substances with poor crystal symmetry, and thus an increase in the lattice distortion would contribute to a decrease of the κlatt. Indeed, the obtained κlatt of Fe0.98O at 33 GPa where it is in the rB1 stability field is lower than the predicted κlatt of B1 Fe0.98O by the damped harmonic oscillator phonon-gas model with the elastic parameters of B1-type wüstite (Hofmeister, 1999; Jacobsen et al., 2005) (Fig. 2). Such change in the phonon behavior across the B1–rB1 transition of wüstite was also observed in the decrease of Debye sound velocity via Nuclear Resonance Inelastic X-ray Scattering spectroscopy (Struzhkin et al., 2001; Wicks et al., 2017). Further decrease of the κlatt of Fe0.98O above 33 GPa was unexpected because no anomaly has been reported in the high-pressure sound velocities of rB1-type wüstite so far (Badro et al., 2007; Struzhkin et al., 2001; Wicks et al., 2017). The negative pressure dependence of the κlatt of Fe0.98O between 33 and 61 GPa may be attributed to magnetoelastic coupling in wüstite (Struzhkin et al., 2001) or further distortional transition of the rhombohedral unit cell as suggested in Yagi et al. (1985) and Fjellvåg et al. (2002).
3.2 Lattice thermal conductivity of (Mg,Fe)O solid solutions
We also measured the κlatt of (Mg1−xFex)O at 1 bar and 15 GPa at 300 K to examine the compositional dependence of κlatt in a MgO-FeO binary system (Table 2). We found that the obtained κlatt of magnesiowüstite is much lower than that of MgO, FeO and ferropericlase at both 1 and 15 GPa, and the compositional dependence in the κlatt of the (Mg,Fe)O solid solution does not follow the averaging model of the κlatt of MgO and FeO endmembers (broken lines in Fig. 3):
(1) |
Experimental composition, pressures, sample thickness, and thermal diffusivity and conductivity of (Mg1−xFex)O.
Pressure (GPa) | FeO content, x | Sample thicknessa (μm) | Thermal diffusivity (mm2/s) | Thermal conductivity (W/m/K) |
20 (1) | 0.40 | 7.54 (9) | 0.81 (3) | 2.99 (12) |
1.5 (1) | 0.50 | 2.32 (2) | 0.55 (10) | 2.03 (39) |
0 | 0.80 | 1.50 (10) | 0.47 (7) | 1.76 (26) |
0 | 0.95 | 6.56 (4) | 0.36 (1) | 1.37 (21) |
15.3 (8) | 0.40 | 5.53 (49) | 1.10 (20) | 4.15 (76) |
15.9 (8) | 0.50 | 2.27 (2) | 1.47 (29) | 5.60 (203) |
15.3 (8) | 0.80 | 8.13 (31) | 0.89 (7) | 3.42 (26) |
15.0 (8) | 0.95 | 3.93 (5) | 0.96 (4) | 3.85 (16) |
14.9 (8) | 1.00 | 17.02 (88) | 4.12 (43) | 16.82 (174) |
a After correction for pressure effect using P-V-T EOS of (Mg1−xFex)O (Fei et al., 2007; Zhuravlev et al., 2010; Fischer et al., 2011b; Wicks et al., 2015; Solomatova et al., 2016).
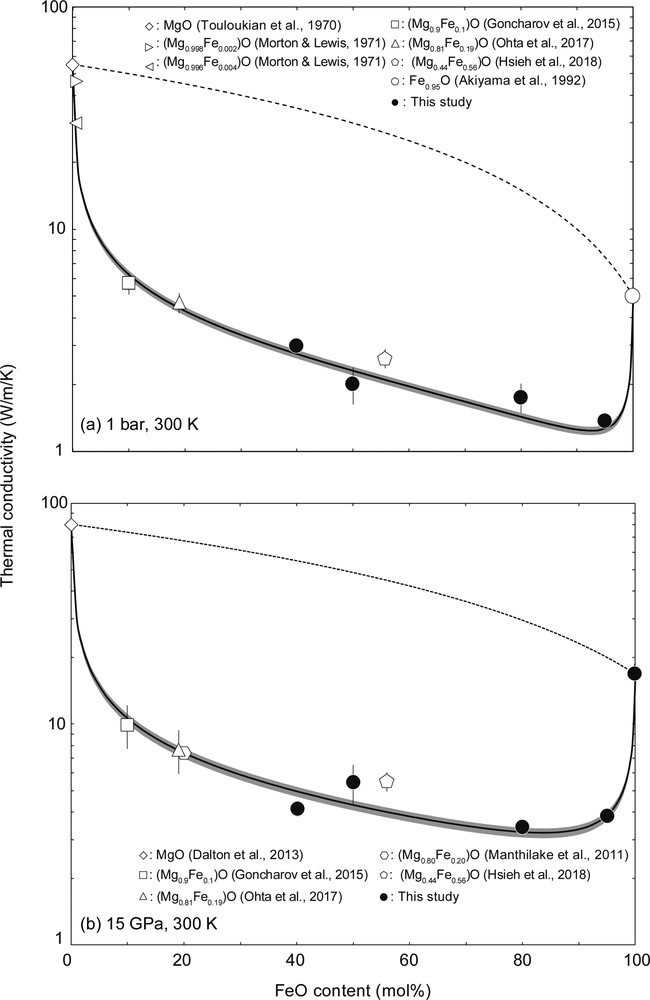
Room temperature κlatt of (Mg1−xFex)O as a function of iron content at (a) 1 bar and (b) 15 GPa. Filled circles, this study; diamonds, MgO (Dalton et al., 2013; Touloukian et al., 1970); right and left-pointing triangles, MgO with 3000 ppm Fe and 7500 ppm Fe, respectively (Morton and Lewis, 1971); square, (Mg0.9Fe0.1)O (Goncharov et al., 2015); triangle, (Mg0.81Fe0.19)O (Ohta et al., 2017); open hexagonal, (Mg0.80Fe0.20)O (Manthilake et al., 2011); open pentagon, (Mg0.44Fe0.56)O (Hsieh et al., 2018); open circle, Fe0.95O (Akiyama et al., 1992). Broken lines indicate the averaging model (Eq. 1) and solid black curves are the fitting results by Eq. (2).
A model of κlatt of a substitution type solid solution proposed by Padture and Klemens (1997) may be applied to the κlatt in the MgO-FeO system:
(2) |
where T is the temperature, and χ is a constant. We fitted the present and previous data of κlatt of (Mg1−xFex)O at 1 bar using this model, and obtained χ = 2.11(19) × 10−6 (K−1) (Akiyama et al., 1992; Goncharov et al., 2015; Hsieh et al., 2018; Morton and Lewis, 1971; Ohta et al., 2017; Touloukian et al., 1970) (black line with gray band in Fig. 3a). We applied the same analysis to the present data and the κlatt of MgO, (Mg0.9Fe0.1)O, (Mg0.81Fe0.19)O, (Mg0.80Fe0.20)O, and (Mg0.44Fe0.56)O at 15 GPa (Dalton et al., 2013; Goncharov et al., 2015; Hsieh et al., 2018; Manthilake et al., 2011; Ohta et al., 2017), which yielded χ = 2.89(30) × 10−6 (K−1) (black line with gray band in Fig. 3b). The κlatt of the MgO–FeO system is well reproduced with the Padture and Klemens model (Eq. (2)). This indicates that substitutional solute atoms scatter phonons as a result of differences in atomic masses, differences in atomic binding forces, and lattice distortion introduced by the presence of the solute atoms as remarked in Padture and Klemens (1997). Our results indicate that the Padture and Klemens model (Eq. (2)) can be used as a guide to estimate the κlatt of such variable compositions in the MgO–FeO system present in the B1 structure if the κlatt values of the MgO and FeO endmembers are known.
4 Geophysical implications
One can consider that the iron content of (Mg1−xFex)O would not be uniform in the lower mantle because of the findings of natural (Mg1−xFex)O inclusions in diamond with diverse composition (Palot et al., 2016; Wirth et al., 2014). High-pressure experiments also show the variable iron partitioning coefficient between ferropericlase and bridgmanite (e.g., Irifune et al., 2010; Piet et al., 2016). We confirmed that a combination of the Padture and Klemens model and κlatt of (Mg,Fe)O endmembers is an effective way to estimate the κlatt of (Mg,Fe)O at the Earth's lower mantle conditions. Note that both MgO periclase and FeO wüstite have a B1-type structure across all conditions that exist in the Earth's lower mantle (McWilliams et al., 2012; Ozawa et al., 2011).
Lattice thermal conduction is not the sole heat transport mechanism in solid (Mg,Fe)O, and radiative and electronic conduction may be important in the Earth. The high-pressure optical absorption measurements on (Mg0.85Fe0.15)O ferropericlase indicated that the obtained radiative thermal conductivity (κrad) was 0.1∼0.2 W/m/K at the lowermost mantle conditions (Lobanov et al., 2017). Iron enrichment lowers the κrad of (Mg1−xFex)O, and thus the κrad of magnesiowüstite would be much smaller than that of ferropericlase. Experimental and theoretical evidence for the metallization of (Mg,Fe)O at lower mantle conditions has been reported (Fischer et al., 2011a, 2011b; Holmström and Stixrude, 2015; Holmström et al., 2018; Ohta et al., 2012, 2014). The reported values of the electrical conductivity of metallic (Mg,Fe)O are ∼0.4–1.1 × 105 S/m depending on iron content, pressure, and temperature, which corresponds to an electronic thermal conductivity of ∼3.6–10 W/m/K according to the Wiedemann–Franz law (i.e. Holmström et al., 2018). Such an electronic contribution will be added to κlatt when metallization of (Mg,Fe)O occurs in the lower mantle.
Finally, in this study we found that the present experimental technique and model permits us to estimate the κlatt of (Mg1−xFex)O on a wide variety of iron contents at high pressure from those of MgO and FeO endmembers. The pulsed light heating thermoreflectance system combined with a laser-heated DAC is currently feasible and ongoing. Direct measurement of thermal conductivity of iron-rich (Mg,Fe)O in-situ at high pressure and high temperature is the next step to constrain thermal conductivity in the deep mantle.
Acknowledgments
This work was supported by JSPS KAKENHI Grant numbers 15H05827 and 17H04861.