1. Introduction
In an overview of problems that the world’s water resources would face in 2050, De Marsily [2007] wrote, “The perceived potential risks were linked to climate change consequences for water resources availability, to the increase of the World’s population, to the impact of society on water ecosystems, to potentially deteriorating drinking water quality,1 and to the increasing number of mega-cities (with more than ten million inhabitants) mostly in the developing world”.
Climate change influences all fluxes of the water cycle: rainfall, evapotranspiration, and discharge regimes via the frequency of extreme events, such as floods and droughts. In addition to these hydrologic impacts, surface water quality also responds to climate change [Delpla et al. 2009; Eekhout and de Vente 2022; Michalak 2016; Ryberg & Chanat 2021; Whitehead et al. 2009]. Indeed, water pollution is related directly to urban, industrial and agricultural activities of human beings, all of which are modified by, and contribute to, climate change. For example, in response to increased water and temperature stress over the centuries, human beings have continuously adapted their agricultural practices, which have subsequently led to profound changes in water quality [Merot et al. 2014]. Water quality degradation is a global and growing issue that influences not only economic and social welfare but also the sustainability of ecosystems and biodiversity [Michalak 2016]. However, there remains large uncertainty linking water quality changes, their causes, and their severity to changes in climate.
Changes in water quality in response to climate change are more challenging to address than changes in water quantity for three main reasons. First, data on water quality, chemical concentrations, and chemical fluxes are usually less common, with shorter time-series and more parameters than typical hydrologic flux and storage measurements. Second, we lack detailed understanding about feedbacks between climate and water quality [Lintern et al. 2021], whereas feedbacks between climate and hydrology are better understood from global and regional hydrologic modelling. Third, it is difficult to parse the confounding effects of climate change on water quality with those of changes in human activities, particularly agricultural activities, which makes it difficult to distinguish true climate effects [Lungarska & Chakir 2018].
These challenges in linking water quality to climate change are particularly difficult in populated temperate zones, where downstream waters are influenced by both point-source wastewater-treatment plants, industrialised agriculture and its associated diffuse inputs of nutrients and pesticides. In these regions, the intensification of agricultural activities that began in the 1960s (e.g., increased production and simplification of agricultural systems, increased energy demands, grassland and hedgerow destruction) has recently abated in response to environmental regulations after the 1980s. Despite these regulatory efforts, researchers observed persistent retention of chemical elements (especially nutrients) in the soil and groundwater and the need for long response times to mitigate these stocks in the environment [Dupas et al. 2020]. These storage processes may lead to unsatisfying lag times in water quality response, especially as climate change effects on hydrology can reduce or exacerbate transport at multiple time scales (e.g. long-term trends, inter-annual, seasonal, event-scale) [Watts et al. 2015].
There are several linked effects among climate change, hydrology, and water quality. For example, reduced water availability induced by climate change is likely to decrease dilution effects during low-flow periods, thereby increasing concentrations of potentially harmful solutes. For example, modifications of soil and water temperature [Ducharne 2008; Seyedhashemi et al. 2022], soil water content, and drying and wetting cycles of catchments directly modify primary production, respiration, and decomposition, and indirectly modify water quality. In the short term, floods, which are increasing in frequency and extent under climate change [Hirabayashi et al. 2013; Arnell et al. 2016], can rapidly bring huge quantities of nutrients, especially in particulate form, which connect areas of the agricultural or urban landscape mosaic to hydrographic networks. On the other hand, drought, which is also increasing in frequency and duration [Prudhomme et al. 2014; Spinoni et al. 2018], can disrupt the biogeochemical processing functions of stream networks and even modify microbial populations and their functional abilities [Mosley 2015]. In the long term, the increase in the atmospheric carbon dioxide concentration increases plant growth, which is an important component of nutrient balances and the water cycle (e.g. interception, transpiration) in agricultural catchments, and thus of potential losses. Indirect effects caused by progressive adaptations of agriculture can contribute to changes in water quantity and quality [Salmon-Monviola et al. 2013].
Along with these basic processes, many complex interactions among climate, nutrient cycles, and stream water flows can be highlighted, even at the local scale in agricultural headwater catchments, and antagonistic effects can occur at the same time. For example, biotransformation can be accelerated by higher temperature but slowed by lower soil water content. C, N, and P exports can be accelerated in winter and slowed in summer. Climate change can modify hydrological connectivity (i.e. flow pathways, velocities, and transport capacities) in catchments, whether between fields due to land use and ecological infrastructure, or between hydrological compartments (e.g. groundwater, riparian wetlands) [Ehrhardt et al. 2021], and therefore can also modify concentration dynamics [Aubert et al. 2013a; Strohmenger et al. 2020]. However, modifications of the water cycle can also influence the resident time of water in these compartments, biogeochemical reactivity, and regulation of pollutant export from agricultural land. These changes in water quality can have differing effects on dissolved and particulate forms and on concentrations of a variety of chemical species, nutrients, pesticides, or emerging pollutants.
Literature on climate change effects on water quality is moderately abundant, having increased significantly since 2008. When combining queries for “water quality” and “climate change” in Web of Science, most of the articles we found were focused on aquatic ecosystem health (e.g. habitats; eutrophication; ecological effects, especially on emblematic organisms). Fewer studies focused on physical or chemical water quality. Literature reviews in this subject area [Delpla et al. 2009; Giri et al. 2021; Watts et al. 2015; Whitehead et al. 2009] highlight the inherent complexity of interactions between climate change and water quality and the fact that they are often studied at different spatial scales [Michalak 2016]. While climate change is studied at global and regional scales, water quality is usually studied locally. Effects of climate on water quality are analysed using three main approaches [Delahaye & Gascuel-Odoux 2013]: (i) experiments under controlled climate conditions [Forber et al. 2017; Gu et al. 2018], (ii) simulations of human activities and climate scenarios using integrated agro-hydrological models [Oni et al. 2012], and (iii) retrospective analysis of long-term data time-series from research and operational observatories [Worrall et al. 2015; Zhang et al. 2013].
The experimental approach is well suited for soil processes but not for the catchment scale. Consequently, effects of climate change on the water cycle and river regimes have been analysed mainly by simulation modelling studies that predicted water flows under future climate-change scenarios at multiple time scales. Theoretically, this modelling approach is the most relevant, and some models have been developed for large catchments [Kaushal et al. 2014; Garnier et al. 2018; Rozmeijer et al. 2021; Dallison et al. 2022], but simulations are subject to multiple sources of uncertainty [Whitehead et al. 2009]: human-activity scenarios, emission scenarios used in global climate models (GCM); the GCM models themselves (e.g. due to uncertainty in understanding of processes, parameter values), downscaling uncertainty, and uncertainty in agro-hydrological models, as they are used to simulate climate conditions beyond those for which they were calibrated [Refsgaard et al. 2014]. This suggests that the overall uncertainty of the modelling approach will be so large as to render its predictions questionable. For example, Salmon-Monviola et al. [2013] predicted that climate change would likely have the net effect of decreasing nitrate concentrations, but that the effect depended strongly on the denitrification function used in the model, and that climate change could modify this function by influencing microbial activities. Moreover, models cannot easily analyse the climate variability component of climate change due to effects that cascade from climate scenarios to water quality, water quantity and agriculture that cannot be known or represented in models. Therefore, despite limited availability of long-term data, the retrospective approach ultimately appears to be the most relevant, and the present article focuses on synthesising retrospective analyses.
The objective of this article is to illustrate effects of climate change on water quality in headwater catchments in temperate zones of western Europe, using examples of retrospective analysis of long-term data time-series from long-term research or operational observatories (Figure 1), and then to identify and highlight research perspectives for studying these relations.
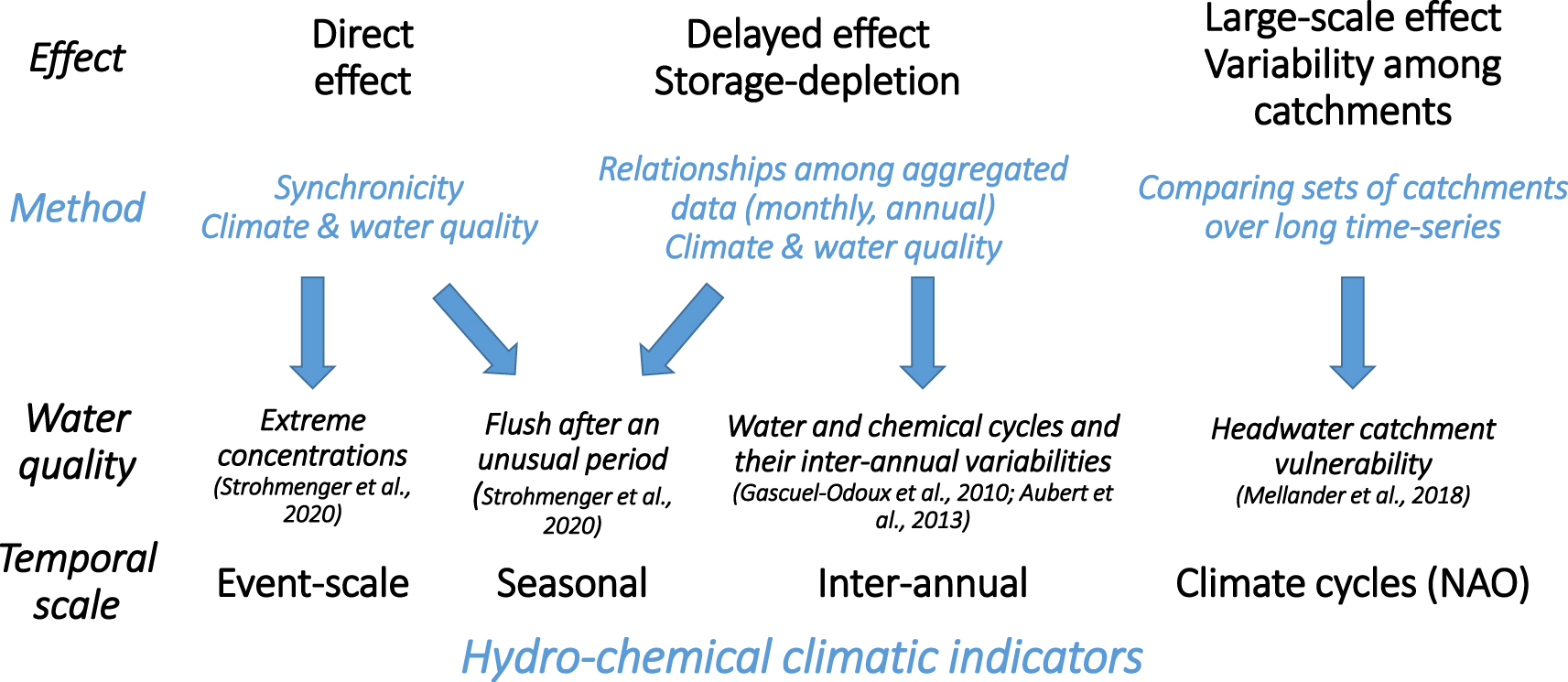
Conceptual framework, where the examples used to illustrate effects of climate change on water quality are classified by the method, specific objective, and temporal scale, using retrospective analysis of long-term data time-series of headwater catchments in temperate zones of western Europe.
2. Retrospective data analysis of effects of the climate on water quality
2.1. Searching for synchronous climatic and chemical anomalies at multiple temporal scales
The two following examples were based on a daily dataset from 2000–2020 from the AgrHyS French Critical Zone Observatory [Fovet et al. 2018; Gascuel-Odoux et al. 2018], in the Kervidy-Naizin headwater catchment (Brittany, France), a 4.9-km2 agricultural area that drains a second-Strahler-order intermittent stream with mean annual precipitation and discharge of 810 ± 180 and 296 ± 150 mm for 2002–2017, respectively. The catchment contains intensive mixed crop-livestock farming, with 91% of its area under agriculture, mainly crops used for animal feed, such as maize (silage or grain) and grasslands.
2.1.1. Research
- At the event scale, Strohmenger et al. [2020] analysed effects of climate on the frequency of solute-concentration classes (C, N, and P) to capture changes in low and high concentrations over time. They hypothesised that effects of climate, particularly its highest variability, could be more apparent on extreme values. Focusing on extreme values is an original way to assess relations between climate and chemical signals in water, which is particularly relevant for storm events.
- At the seasonal scale, Strohmenger et al. [2020] analysed effects of climate on chemical flushing, when flow starts in autumn, as a function of the length of the dry phase in an intermittent stream. Relations between climate conditions and water quality can be revealed at the seasonal scale, particularly when climate conditions influence the hydrological regime.
2.1.2. Conclusions
These two examples analysed specific temporal patterns of water quality, extreme concentrations, and hydro-climatic drivers, as well as concentrations during flushing after the summer dry phase, their timing during the water cycle, and their duration and intensity. Because the water quality signal was weak and dominated by moderate values, focusing on extreme concentrations or on atypical/extreme periods in catchment was a way to simplify it by focusing on the concentrations or periods that were influenced the most.
2.2. Annual and inter-annual variabilities: cycles controlled by climate, internal storage, and depletion of this storage in a catchment
The first example is again in Kervidy-Naizin catchment, while the other two focus on a set of coastal rivers of an agricultural region (Brittany, France) with intensive mixed crop-livestock farming and crops used mainly for animal feed. Nutrient and pesticide pressure in this region has been high for decades.
2.2.1. Research
- At the annual scale, Aubert et al. [2013b] analysed effects of climate conditions on seasonal patterns of stream-water chemistry by plotting monthly mean in-stream concentrations of five solutes (i.e. nitrate, chloride, sulphate, and dissolved organic and inorganic C) vs. monthly mean air temperature or discharge. All solutes displayed hysteresis loops due to the time needed to rewet catchment soils, and the direction of the loop depended on the spatial control of each element. Comparison of chemical concentrations during wet vs. dry years and cold vs. hot years showed the influence of catchment drying–wetting cycles on chemical export. This method, used here to study inter-annual climate variability, could also be used to capture effects of climate change over a longer time-series.
- At the inter-annual scale, Gascuel-Odoux et al. [2010] showed that annual cyclic patterns of concentrations depended on those of previous years, by plotting annual mean nitrate concentrations vs. annual mean nitrate fluxes for several rivers in Brittany (western France). Dupas et al. [2018] performed a similar analysis for five rivers whose data time-series were longer but had a lower temporal resolution, by plotting N loads vs. N surplus over time. In the medium term (ca. 10 years), climate variability was the main driver of concentration dynamics due to the strong legacy effect in these rivers’ catchments.
2.2.2. Conclusions
Due to noisy daily or weekly signals in concentrations, annual and inter-annual patterns and their relations to hydro-climatic variables cannot be identified without aggregating the data (here, monthly and annual, respectively). In these two examples, the hysteresis relations observed indicate that climate influences chemical export by mobilising and depleting chemical storage in the catchment, which indicates an indirect climate effect via water flow.
2.3. Aggregation over time and space: effects of the North Atlantic Oscillation in north-western Europe
The previous examples were at the scales of a small catchment (Kervidy-Naizin) or region (i.e. a set of neighbouring catchments in Brittany). Analysis of climate change at the scale of a larger region of Europe requires integrated approaches. Here, we present the development of “climate-chemical” indicators of diffuse pollution based on a large set of highly monitored agricultural catchments in western Europe, with a high diversity of agricultural intensification and environmental contexts, considered as representative of the response of water quality dynamics to hydro-climatic dynamics [Mellander et al. 2018].
2.3.1. Research
Continental-scale climate patterns, characterized by the intensity of the North Atlantic Oscillation (NAOi), were found to influence nutrient export to water differently among rivers in agricultural catchments in north-western Europe (i.e. Ireland, Norway and north-western France). A sharply increasing trend in NAOi, which has amplified annual weather patterns in recent years, may override positive benefits of local management depending on the catchment and year. Because these effects depend on a catchment’s characteristics, agricultural history (inputs), and flow pathways, the catchment’s response is specific and cannot be predicted easily.
2.3.2. Conclusions
The use of the ocean-scale NAOi, whose prediction still includes many uncertainties at the local scale, and local-scale water quality data is an example of using a climate-chemical indicator in the framework of the science of integrated systems. Developing climate-chemical indicators of diffuse pollution in highly monitored catchments in western Europe is useful for increasing the predictability of their vulnerability.
3. Perspectives for identifying effects of climate change on water quality
Some conclusions and research perspectives can be drawn from these examples.
3.1. Functional typology of chemical elements
Observation, modelling, and retrospective analysis cannot be performed for all chemical parameters related to water quality. A typology needs to be created that considers (i) the operational feasibility (e.g. cost, technical analysis) of long-term monitoring, if possible at a high temporal resolution, which is necessary to detect changes at all temporal scales; (ii) characteristics of chemical elements, particularly their affinity for soil and their sources (e.g. human inputs), and thus their flow pathways in a catchment; and (iii) research sites, to monitor specific key compartments related to hydro-climatic processes (e.g. soil, wetlands, groundwater). Because nutrients (C, N, and P) generally meet these three considerations, they could be considered as model elements for assessing effects of climate change on water quality. Nevertheless, it remains difficult to include all three of these elements (and their chemical species) simultaneously in long time-series; thus, doing so could be made a priority. We recommend monitoring suspended matter as well because it carries many elements adsorbed on soil particles and reacts strongly to storm events [Piazza et al. 2018]. Pesticides, and more generally organic compounds, would be important to consider, but their data time-series likely do not allow for retrospective analysis of effects of climate variability.
3.2. Multi-temporal and multi-spatial analysis: aggregating data in space and time to capture trends
Interactions between climate and water quality must be considered across a cascading range of multiple temporal and spatial scales [Aubert et al. 2015; Michalak 2016]. The examples presented have adequately illustrated key temporal scales (e.g. storm-event, seasonal, annual, and inter-annual variabilities; long-term trends). We stress the utility of considering their timing within time-series as well. For example, water quality during storm events depends on when storms occur during the annual cycle [Aubert et al. 2013c; Dupas et al. 2015; Piazza et al. 2018]. Water quality during a given year depends on previous inter-annual cycles. We also emphasize the utility of considering time not only on an absolute scale but on a contextual scale, by considering past dynamics from the viewpoints of climate (e.g. inter-annual phenomena such as NAO), hydrology (e.g. connectivity of flow pathways, catchment wetness), hydrochemistry (e.g. internal storage related to the history of human activity; changes in microbial biotransformation functions in soils, particularly in wetlands) and agricultural activities. For example, the dry year of 1976, which is often considered the beginning of increasing nitrate concentrations in north-western Europe, combined a hot and dry summer with low plant growth, which caused soil N surpluses that leached during the following rainy winter and contributed greatly to internal storage of N in the groundwater and N export [Gascuel-Odoux et al. 2010; Hong et al. 2005; Jones & Smart 2005].
Effects of climate change as a function of spatial scale have been illustrated less often. Mellander et al. [2018] clearly observed large differences in the responses of headwater catchments to climate conditions and the many drivers involved. This scale of approach highlights the utility of developing simple indicators that can be applied easily to many rivers, such as the mean nitrate concentration per month or per water year [Ebeling et al. 2021]. Effects of the climate on river continuums are rarely analysed [Abbott et al. 2018; Minaudo et al. 2021]. From upstream to downstream, a river’s internal functioning, deposits, remobilisation of deposits, consumption, and biological production can smooth the variability upstream, and climate can have a dominant influence on water quality. Moreover, the footprint and complexity of types of feedback of climate change depend on the spatial scale. Transit times in hillslopes and rivers, as well as flow pathways between soil, groundwater, and rivers, are a function of catchment size and stream order [Fovet et al. 2015]. It is therefore essential to assess effects of climate change on water-quantity/quality interactions, acquire observations at multiple scales, and develop equations to describe scale effects [Pinay et al. 2015].
3.3. Decoupling effects of the climate and human activities: from analysis of multiple data time-series to testing of climatic scenarios with new models
The future of water quality, especially for elements emitted by human activities depends on changes in these activities. Regardless of the changes, whether abrupt (i.e. over a few years) or slow (i.e. over a few decades), responses of water quality to them are always smoothed over time, as identified by combining data bases on these changes, usually in response to regulations (e.g. European Union’s Water Framework Directive, Common Agricultural Policy, or Strategy on Green and blue Infrastructure). When effects of human activities cannot be identified, effects of the climate are often hypothesised, whether they have been demonstrated or not. Analysis of multiple chemical elements endogenous to a catchment, such as dissolved organic C, whose dynamics depend mainly on climate conditions [Evans et al. 2005], can also help identify climate effects on other chemical elements, as can analysis of chemical dynamics in key hydrological compartments. Supporting these hypotheses, however, often requires combining approaches, including modelling [e.g., Birkel et al. 2014].
The models that can be used are diverse, such as simplified physical-based one-dimensional models of hillslope storage [Guillaumot et al. 2021; Marcais et al. 2017], multiple-box models possibly supplemented with [Strohmenger et al. 2021], and semi-distributed models (e.g. INCA, HYPE, SWAT). However, using these models beyond their calibration domains (due to climate change) often raises questions [Nicolle et al. 2021; Refsgaard et al. 2014]. Few models have considered changes in the atmospheric carbon dioxide concentration, which has a strong influence on agroecosystems and thus chemical export (by vegetation). Thus, to test hypotheses, attention must be paid to the processes simulated, calibration/evaluation strategies in changing contexts [Wagener et al. 2022], and the flexibility of the model itself, which influences the ability to simplify it or make it more complex. To improve consideration of scales in changing contexts, physical realism of models is important, without being over-parameterized; the most important characteristic is to remain consistent with agronomic, climatic, and hydrological hypotheses [Kirchner 2006].
3.4. Incorporating water quality and aquatic ecosystem health: analysing changes in stoichiometry, habitat, and abiotic and biotic conditions
Changes in water quality are often analysed based on one or a few chemical elements but not as a whole, which could lead to negative consequences on habitats, aquatic organisms, and ecosystems. The French scientific expertise on eutrophication [Pinay et al. 2017], after reviewing the literature, highlighted an increasing risk of eutrophication as one effect of climate change, due to cascading effects. Changes in stoichiometric C:N:P ratios have been rarely studied [Pinay et al. 2017; Le Moal et al. 2019]. New methods are needed to analyse element concentrations and patterns, compare them, and analyse loads and element ratios, which could have effects on organisms. Many studies have focused on climate effects on organisms without explicitly relating them to abiotic parameters (i.e. physical and chemical water quality) or their impacts on habitats.
4. Conclusion
Despite difficulties in identifying effects of climate change on water quality at the multiple temporal and spatial scales at which they operate, retrospective studies have highlighted that these effects can be numerous, as demonstrated by observation of long-term time-series. These effects on water quality vary greatly among storm-event patterns (e.g. extreme values, especially for elements associated with particles; concentration distributions), seasonal patterns (e.g. change in seasonal timing, range of variation in summer and winter, flushing after intermittent flow), annual cycles (C/Q or C/T annual hysteresis), and inter-annual cycles (C/Q or C/T inter-annual hysteresis due to hydro-climatic phenomena). These effects can be characterised by hydro-chemical indicators.
These effects need to be investigated in more detail to characterize them and their potential interactions with mitigation measures to restore water quality. Time must be considered explicitly due to chemical legacies in catchments. Long-term observation at higher temporal resolution to capture as many extreme events as possible is one recommendation for research observatories and for river monitoring for the Water Framework Directive. Due to the noise and diversity of water quality data, temporal aggregation over functional periods and spatial aggregation from multiple headwater catchments to river continuums is necessary to demonstrate certain effects of climate on water quality. Modelling is facing new challenges, in which the objective is not to represent current activities but to represent and test the chemical legacies in catchments and their mobilization due to climate conditions, variabilities, and trends.
Society increasingly focuses on the quantity of water (e.g. groundwater, lake and river water, reservoirs) with regard to demographics (e.g. urbanization, migration, drinking water), agriculture, and food security. It is essential, however, to combine the analysis of water quantity and quality. Doing so would require strengthening relations among scientific communities in integrated ecosystem approaches and investigating wider topics (e.g. the water-energy-agriculture nexus, food), particularly when addressing water quality in the context of the ecological transition of societies and adaptation to climate change. Transforming food systems, water and energy consumption in agriculture, and agricultural systems according to agroecological principles, particularly by decreasing agricultural inputs, can help mitigate degradation of water quality, now and under the future climate.
Conflicts of interest
Authors have no conflict of interest to declare.
Acknowledgments
We thank all those who help monitor water quality in the headwater catchments mentioned. We also thank Michelle and Michael Corson for English copyediting and proofreading of the article.
1 Underlined in the original text.