Key points
- Mururoa's chalky limestones collapse at 16–18 MPa of effective pressure.
- Brittle faulting of chalky limestone can occur below 6 MPa of effective pressure.
- The resistance of chalky limestones is lowered under water saturation.
- Under deviatoric stress, increasing brittle hardening and shear-enhanced compaction are promoted with increasing effective pressure, either in wet or dry conditions.
1. Introduction
The deformation of chalks can have significant impacts on the mechanical instability of underground mines and reservoirs [Al Heib et al., 2015, De Gennaro et al., 2004, Georgieva et al., 2020, Geremia et al., 2021, Keszthelyi et al., 2016, Renaud et al., 2019, Risneset al., 1994, Sulak, 1991]. This deformation can sometimes lead to rock collapse, as for coastal cliffs [Brossard and Duperret, 2004, Duperret et al., 2002, 2004, El Khattabi et al., 2018, Mortimore et al., 2004, Senfaute et al., 2009] and submarine slopes, that could induce tsunamigenic waves [e.g. Abadie et al., 2012, McGuire, 2006, McMurtry et al., 2004, Ward and Day, 2001, Wright and Rathje, 2003]. Slope instabilities are frequent on volcanic islands [Rowberryet al., 2023] but remain under-documented on atolls.
An atoll is a ring-shaped coral reef island, usually surrounding a central lagoon. Most of the world’s atolls are in the Pacific Ocean. This is the case of Mururoa Atoll (French Polynesia, France; Figure 1a), an atoll famous as the site of the Centre d’Expérimentation du Pacifique (CEP). 147 underground nuclear tests were carried out there between 1975 and 1996. One of these nuclear tests (Tydée) was followed by a submarine flank collapse and a tsunami generated at the Southwest rim of the atoll in 1979 [Poupardin et al., 2017; Figure 1a].
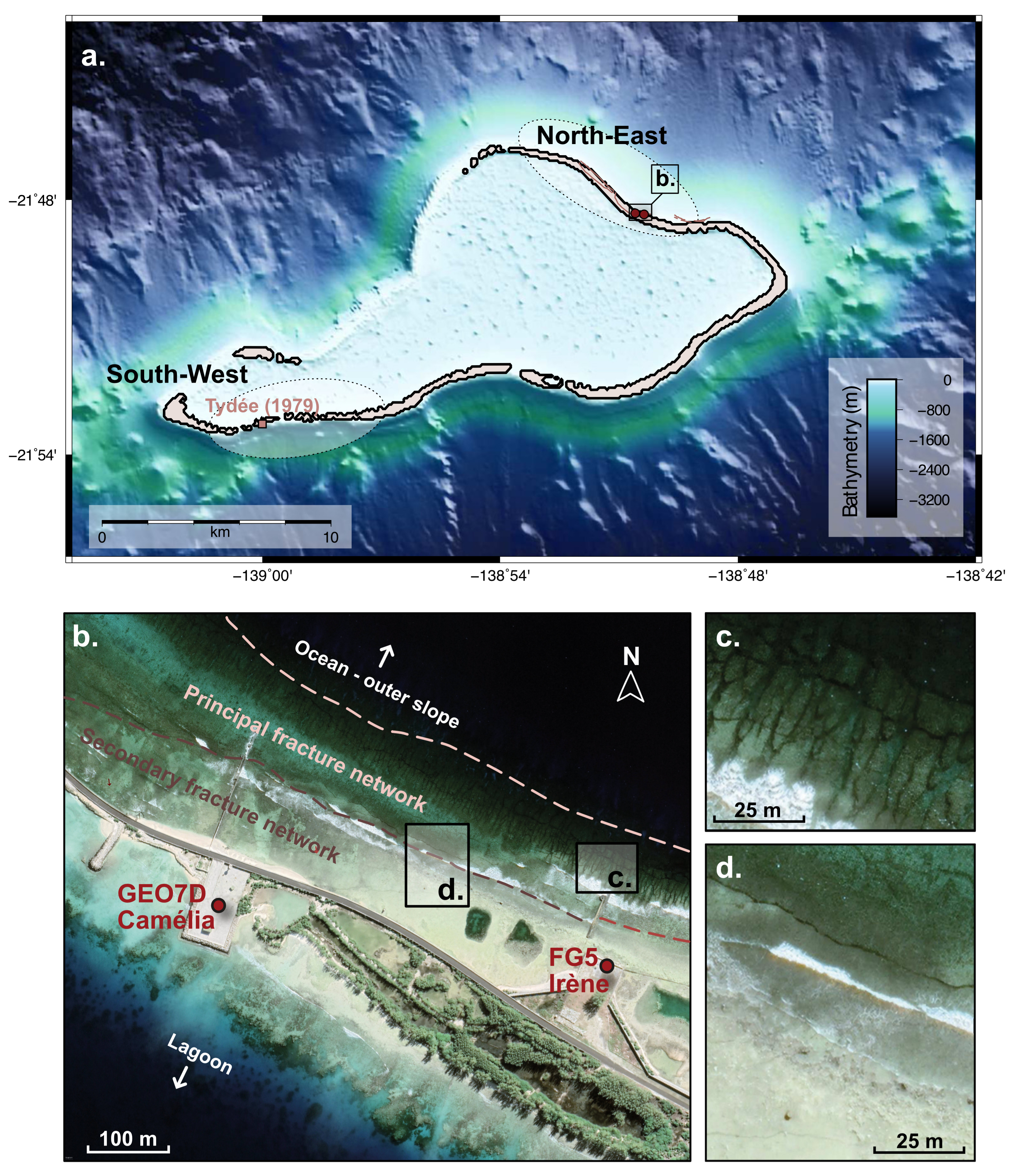
(a) Bathymetry and location of the atoll of Mururoa and (b) close-up view of the north-east area with the main fracture networks (c) and (d).
After Tydée, the Military Applications Division of the French Alternative Energies and Atomic Energy Commission (CEA-DAM, Bruyères-le-Châtel, France) set up several generations of systems to monitor the mechanical stability of Mururoa and the safety of neighboring atolls. The monitoring devices included topographic networks (presently composed of continuous GPS, supplemented by frequently measured reference points), seismic stations, tiltmeters, extensometers and trigger alarms. The atoll coral rim is divided morpho-geologically into several regions: an emerged zone, which is distinguished from the inner platform (towards the lagoon, with knolls on the surface) and the outer coral rim (open to the ocean, built on the 12-million-year-old volcano) which includes the reef platform, the algal ridge and the outer slope of the atoll [Buigues et al., 1992, Perrin, 1990; Figure 1b].
The International Society for Rock Mechanics recommended 20 years of monitoring after the last nuclear tests in 1996 [Fairhurstet al., 1999]. In 2016, CEA-DAM decided to continue to monitor the atoll. Instruments that had become obsolete were replaced. New boreholes were drilled in order to instrument the northeastern rim of the atoll, including lateral inclined boreholes for extensometers, and vertical boreholes to monitor the stress/strain heterogeneities with tiltmeters and seismic stations at depth. A 608 m-long core, GEO7D, was drilled and collected, complementing an older core (FG5) sampled earlier in the vicinity. These cores document the entire sedimentary sequence down to the volcanic rocks sequence, which were reached at a mean depth of 600 m, and complement previous core drilling dating back from the 1980’s. Succinctly, the cores displayed carbonates lithologies that lay on volcanic rocks. In carbonates, some porous rocks, called chalky limestones, were affected by an intense deformation. The behavior of this lithological unit is suspected to be related with the displacement of the northeastern flank of the atoll that has reached 2 m locally. The displacement rate has progressively decreased over time [CEA/DAM, 2021]. In order to gain an understanding of the long-term mechanical behavior of chalky limestones sampled in the atoll’s rim, we conducted experiments in a laboratory setting.
Compaction experiments are generally performed under hydrostatic or triaxial loading. In the hydrostatic case, grain crushing and pore collapse occur at a critical stress P∗ at the onset of inelastic compaction, whether localized or distributed [Dubaet al., 1974, Dunn et al., 1973, Fortin et al., 2007, Schock et al., 1973, Wong et al., 1992, 2001, 2004, Wong and Baud, 2012, Zaman et al., 1994, Zhang et al., 1990]. Under triaxial loading and confining pressure, the onset of compaction and crack closure (C′) is followed by a transition towards strain hardening at a critical stress C* during which shear-enhanced compaction occur. In carbonates and porous limestones, dilatancy, microcracking and shear localization occur at low confining pressure while strain-hardening and inelastic (shear-enhanced) compaction, accommodated by crystal plasticity or dilatancy, generally occur at high confining pressure [Baud et al., 2004, 2009, 2017a,b, Fortin et al., 2005, 2009, Menéndez et al., 1996, Nicolas et al., 2016, 2017, Tembe et al., 2008, Vajdova et al., 2004, Wong and Baud, 1999, Wong et al., 2001, Zhang et al., 1990, Zhu et al., 2010]. In both experimental conditions, bulk volume change can be estimated with strain gages in dry conditions [Dubaet al., 1974, Schock et al., 1973] or estimated from a pore volume change in water-saturated conditions [Zhang et al., 1990].
Bouchez et al. [1997] previously studied and deformed chalky limestones from Mururoa in the laboratory. In their experiments, chalky limestones were compacted in dry conditions at confining pressures of 1.5 and 3 MPa—at higher analogue depth compared to the depth of chalky limestone in nature. Their experiments showed that chalky limestones creeped up to 8% of axial deformation. However, Mururoa is a water-saturated atoll, subdivided into gradual fracture zones between the outer slope and the lagoon, with stagnant water with a residence time greater than 100 days in the central part of the atoll [CEA/DAM, 2021]. Knowing that water-weakening has been observed in porous rocks including chalks [Baud et al., 2009, 2017a,b, Castagna et al., 2018, Lisabeth and Zhu, 2015, Nicolas et al., 2016, 2017], the study of the micromechanics of compaction and deformation of water-saturated chalky limestones seems crucial, although not yet explored.
In this study, (i) we document the intrinsic and mechanical properties of the main lithologies sampled in the two cores forementioned, (ii) we present laboratory experimental results on chalky limestones obtained in various conditions and (iii) draw their mechanical yield envelope. The results provide information on the mechanical deformation of the Mururoa atoll, 28 years after the last French nuclear test, offering new insights into the micromechanics of chalk-content rocks in dry and saturated conditions.
2. Methods
2.1. Geological context of the borehole’s cores made available
For this study, we used two borehole’s cores. The sedimentary rocks sampled in the GEO7D core are similar to those of an older core (FG5) sampled 480 m to the east. The two cores help to document lateral variations in the sedimentary rocks that are open to the ocean (Figure 2a). These rocks consist of a set of carbonates, altered sedimento-volcanic and volcanic facies. The upper facies consists of non-cohesive limestones, interbedded with sandstone-sandy units. These limestones are locally affected by surface cracks and fractures along the northeastern rim of Mururoa atoll—between the algal ridge and the emergent zone—(Figure 1c,d), as in the Takapoto Atoll [French Polynesia; Montaggioni, 2005]. The lower facies consists of massive limestones, alternating with highly consolidated dolomites, which are in contact with chalky limestones. Downwards, the base of the carbonate facies is composed of indurated and highly heterogeneous dolomites, followed by a dolomitic transition zone that develops in an argilo-volcanic altered geological complex. The transition zone rests on a volcanic base made up of massive basalts, intercalated with more clayey or vacuolated altered volcanic rocks up to 600 m deep on average. Only chalky limestones were sampled in the FG5 core.
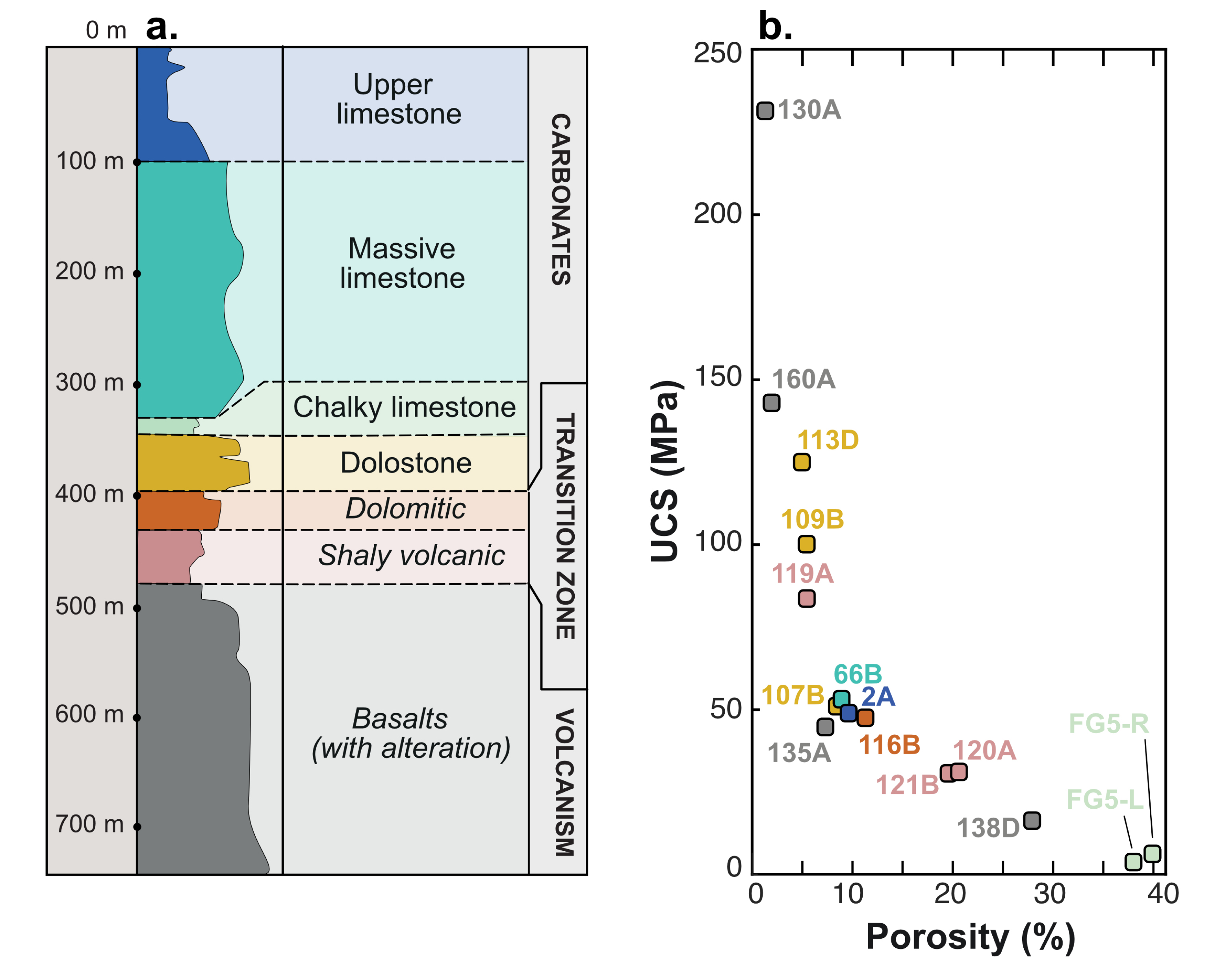
(a) Lithostratigraphic sequence [modified from Buigues et al., 1992] and (b) peak mechanical strength of Mururoa’s rocks revealed by uniaxial compressive strength (UCS) as a function of porosity.
2.2. Intrinsic properties collected prior to the laboratory experiments
A preliminary study on the intrinsic properties of representative lithologies of the borehole’s cores was carried out prior to the experiments. This methodological study helped to locate the chalky limestones within the stratigraphic pile and to understand their differentiated properties compared to other lithologies. Representative samples were selected for uniaxial compressive strength (UCS) tests (Figure 2b). Data on the dry bulk density and the connected porosity 𝜙 (calculated using the triple-weight method) are shown in Table 1 for several lithologies (Figure 3a). Permeability (symbolized by k) was measured with a gas permeameter (hydrostatic core holder with gas as confining fluid, Top Industrie, Laboratoire de Géologie de l’École Normale Supérieure, Paris, France) between 0.1 and 40 MPa (Figure 3b; Table 2). Pore throat diameter and size distribution of chalky limestones (Figure 4; Table 3) were obtained using a mercury porosimeter (Epitopos, Strasbourg, France).
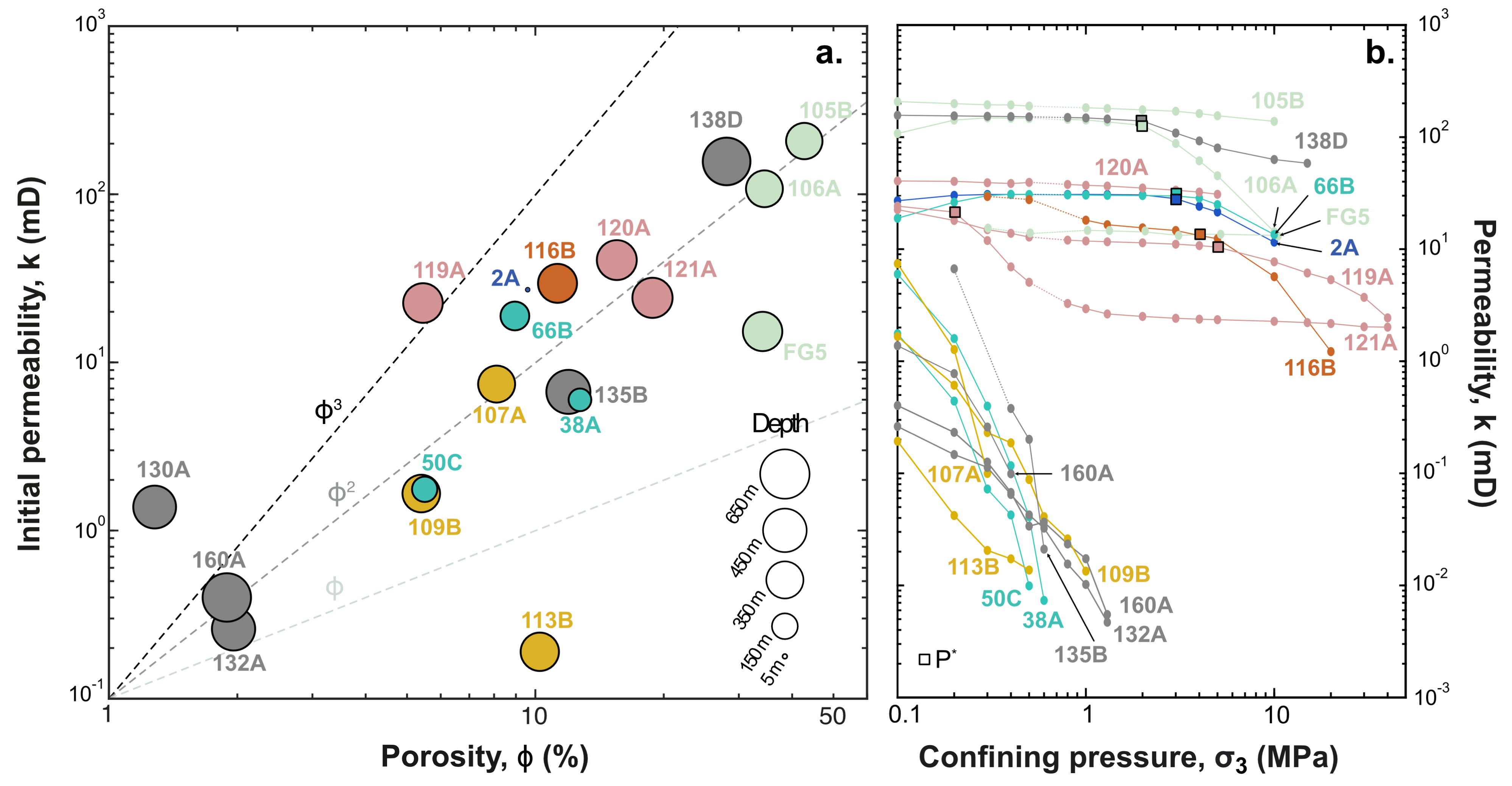
(a) Initial permeability at 0.1 MPa as a function of porosity (color code of Figure 2). (b) Permeability as a function of confining pressure. Permeability is given in mD (1 mD = 10−15 m2).
Summary of intrinsic properties (density, porosity 𝜙 and permeability k at 0.1 MPa) and mechanical parameters (critical pore collapse pressure P∗, yield stress at the onset of compaction and of failure) for the samples of the boreholes GEO7D and FG5
Borehole reference | Lithology | Depth (m) | Density | Porosity 𝜙 (%) | Permeability k at 0.1 MPa (mD) | UCS (MPa) | P∗ (MPa) |
---|---|---|---|---|---|---|---|
GEO7D-2A | UPPER LIMESTONE | 5.5 | 2.08 | 9.59 | 27.06 | 48.95 | - |
GEO7D-2B | UPPER LIMESTONE | 5.5 | 2.39 | 15.13 | - | - | |
GEO7D-22A | UPPER LIMESTONE | 79.4 | 2.42 | 7.97 | - | - | |
GEO7D-23A | UPPER LIMESTONE | 82.4 | 2.6 | 28.63 | - | - | |
GEO7D-31A | UPPER LIMESTONE | 113.3 | 2.71 | 7.48 | - | - | |
GEO7D-31B | UPPER LIMESTONE | 113.3 | 2.57 | 6.86 | - | - | |
GEO7D-31D | UPPER LIMESTONE | 113.3 | 2.46 | 10.72 | - | - | |
GEO7D-35A | MASSIVE LIMESTONE | 124.5 | 2.4 | 25.17 | - | - | |
GEO7D-35B | MASSIVE LIMESTONE | 124.5 | 2.3 | 27.25 | - | - | |
GEO7D-38A | MASSIVE LIMESTONE | 133.3 | 2.64 | 12.72 | 5.99 | - | - |
GEO7D-38B | MASSIVE LIMESTONE | 133.3 | 2.58 | 18.95 | - | - | |
GEO7D-50A | MASSIVE LIMESTONE | 166.8 | 2.42 | 7.55 | - | - | |
GEO7D-50B | MASSIVE LIMESTONE | 166.8 | 2.51 | 4.6 | - | - | |
GEO7D-50C | MASSIVE LIMESTONE | 166.8 | 2.5 | 5.5 | 1.76 | - | - |
GEO7D-66A | MASSIVE LIMESTONE | 214.4 | 2.48 | 12.46 | - | - | |
GEO7D-66B | MASSIVE LIMESTONE | 214.4 | 2.48 | 8.95 | 18.89 | 52.35 | - |
GEO7D-66C | MASSIVE LIMESTONE | 214.4 | 2.46 | 14.27 | - | - | |
GEO7D-104A | CHALKY LIMESTONE | 338.5 | 2.22 | 48.24 | - | - | |
GEO7D-105A | CHALKY LIMESTONE | 343.8 | 2.39 | 31.59 | - | - | |
GEO7D-105B | CHALKY LIMESTONE | 343.8 | 2.34 | 42.71 | 207.21 | - | 16 |
GEO7D-105C | CHALKY LIMESTONE | 343.8 | 2.32 | 37.84 | - | 18 | |
GEO7D-105D | CHALKY LIMESTONE | 343.8 | 2.39 | 32.51 | - | - | |
GEO7D-105E | CHALKY LIMESTONE | 343.8 | 2.4 | 26.4 | - | 18 | |
GEO7D-105F | CHALKY LIMESTONE | 343.8 | 2.23 | 40.85 | - | - | |
GEO7D-106A | CHALKY LIMESTONE | 360 | 2.3 | 36.5 | 107.8 | - | - |
FG5-B | CHALKY LIMESTONE | 482.5 | 2.3 | 40 | - | 14 | |
FG5-C | CHALKY LIMESTONE | 482.5 | - | - | - | - | - |
FG5-D | CHALKY LIMESTONE | 482.5 | - | - | - | - | - |
FG5-E | CHALKY LIMESTONE | 482.5 | 2.3 | 35 | 18.92 | - | - |
FG5-F | CHALKY LIMESTONE | 482.5 | - | - | - | - | - |
FG5-G | CHALKY LIMESTONE | 482.5 | - | - | - | - | - |
FG5-H | CHALKY LIMESTONE | 482.5 | - | - | - | - | - |
FG5-I | CHALKY LIMESTONE | 448.5 | - | - | - | - | - |
FG5-J | CHALKY LIMESTONE | 448.5 | - | - | - | - | - |
FG5-K | CHALKY LIMESTONE | 448.5 | - | - | - | - | 11 |
FG5-N | CHALKY LIMESTONE | 462 | - | - | - | - | - |
FG5-P | CHALKY LIMESTONE | 462 | - | - | - | - | - |
FG5-L | CHALKY LIMESTONE | 462 | 2.3 | 40 | - | 3.04 | - |
FG5-R | CHALKY LIMESTONE | 481 | 2.3 | 37 | - | 6.13 | - |
FG5-X | CHALKY LIMESTONE | 481 | - | - | - | - | - |
GEO7D-113B | DOLOSTONE | 381.2 | 2.58 | 6.96 | - | - | |
GEO7D-113C | DOLOSTONE | 381.2 | 2.57 | 4.61 | - | - | |
GEO7D-113D | DOLOSTONE | 381.2 | 2.59 | 4.96 | 128 | - | |
GEO7D-113E | DOLOSTONE | 381.2 | 2.54 | 4.21 | - | - | |
GEO7D-113F | DOLOSTONE | 381.2 | 2.6 | 4.53 | - | - | |
GEO7D-115A | DOLOMITIC TRANSITION ZONE | 388.7 | 2.56 | 6.15 | - | - | |
GEO7D-116A | DOLOMITIC TRANSITION ZONE | 392.4 | 2.65 | 7.29 | - | - | |
GEO7D-116B | DOLOMITIC TRANSITION ZONE | 392.4 | 2.6 | 11.27 | 46.78 | - | |
GEO7D-116C | DOLOMITIC TRANSITION ZONE | 392.4 | 2.62 | 10.84 | - | - | |
GEO7D-119A | SHALY VOLCANIC TRANSITION ZONE | 406.3 | 2.62 | 5.45 | 22.55 | 83.82 | - |
GEO7D-120A | SHALY VOLCANIC TRANSITION ZONE | 412.9 | 2.45 | 15.52 | 40.59 | 31.35 | - |
GEO7D-120C | SHALY VOLCANIC TRANSITION ZONE | 412.9 | 2.47 | 13.39 | - | - | |
GEO7D-120D | SHALY VOLCANIC TRANSITION ZONE | 412.9 | 2.41 | 20.7 | - | - | |
GEO7D-121A | SHALY VOLCANIC TRANSITION ZONE | 416.9 | 2.35 | 18.82 | 24.21 | - | - |
GEO7D-121B | SHALY VOLCANIC TRANSITION ZONE | 416.9 | 2.37 | 19.77 | 31.28 | - | |
GEO7D-121C | SHALY VOLCANIC TRANSITION ZONE | 417 | 2.51 | 13.18 | - | - | |
GEO7D-130A | VOLCANISM | 475 | 2.91 | 1.28 | 1.37 | 231.72 | - |
GEO7D-132A | VOLCANISM | 485.7 | 2.75 | 1.96 | 0.26 | - | - |
GEO7D-132B | VOLCANISM | 491.1 | 2.74 | 2.22 | - | - | |
GEO7D-133A | VOLCANISM | 501.6 | 2.5 | 10.62 | - | - | |
GEO7D-135A | VOLCANISM | 501.6 | 2.4 | 7.38 | 44.25 | - | |
GEO7D-135B | VOLCANISM | 513.9 | 2.4 | 11.95 | - | - | |
GEO7D-138A | VOLCANISM | 513.9 | 2.48 | 28.7 | - | - | |
GEO7D-138B | VOLCANISM | 513.9 | 2.46 | 33.55 | - | - | |
GEO7D-138C | VOLCANISM | 513.9 | 2.48 | 28.69 | - | - | |
GEO7D-138D | VOLCANISM | 513.9 | 2.45 | 28 | 156.54 | 16.78 | - |
GEO7D-160A | VOLCANISM | 608 | 2.76 | 1.89 | 0.40 | 142.81 | - |
GEO7D-160B | VOLCANISM | 608 | 2.78 | 1.8 | - | - | - |
GEO7D-160C | VOLCANISM | 608 | 2.8 | 2.12 | - | - | - |
GEO7D-160D | VOLCANISM | 608 | 2.78 | 1.95 | - | - | - |
Permeability is given in mD; 1 mD = 10−15 m2. A strong disparity in density and porosity is observed within the basalts, which occur in three forms: a highly consolidated, dense and tightly porous form (porosity between 1 and 2%, sample 160B), a moderately porous form (around 10–12%, sample 135B) and a very altered form, which may be as porous as certain chalky limestones (porosity around 30%, sample 138A). Heterogeneity is also observed amongst the massive limestones (between 5 and 30% porosity, samples 35B vs. 50C) and in rocks of the clay-volcanic transition zone (between 5 and 20%, samples 119A, 120A and 121A). The upper limestones are not very competent and are associated with a disparity of densities ranging from 2.1 to a little more than 2.7 (Table 1). The porosity of these upper limestones is less than or equal to 10% for samples 2A and 31A, although certain levels of bio-constructed limestones reach total porosities between 20 and 28% (sample 23A). Contrary to other lithologies, indurated dolomites and rocks of the dolomitic transition zone show homogeneity in terms of porosity and density (between 4 and 10%, between 2.4 and 2.7, respectively—samples 107A to 116C).
Permeability of the samples as a function of confining pressure (related to Figure 3b)
𝜎3 (MPa) | Permeability of the samples (in mD) | ||||||||||||||||||
---|---|---|---|---|---|---|---|---|---|---|---|---|---|---|---|---|---|---|---|
2A | 38A | 50C | 66B | 105B | 106A | 107A | 109B | 113A | 116B | 119A | 120A | 121A | 130A | 132A | 135B | 138D | 160A | FG5 | |
0.1 | 27.06 | 5.99 | 1.76 | 18.89 | 207.21 | 107.78 | 7.42 | 1.66 | 0.19 | - | 22.55 | 40.59 | 24.21 | 1.37 | 0.26 | - | 156.54 | 0.40 | |
0.2 | 30.20 | 1.59 | 0.44 | 26.27 | 198.81 | 141.95 | 1.27 | 0.61 | 0.04 | - | 18.06 | 40.25 | 21.42 | 0.78 | 0.15 | 6.68 | 154.67 | 0.23 | |
0.3 | 30.74 | 0.39 | 0.07 | 30.05 | 194.06 | 148.51 | 0.09 | 0.23 | 0.02 | 29.52 | 14.95 | 39.14 | 11.94 | 0.26 | 0.11 | - | 153.46 | 0.13 | |
0.4 | 30.77 | 0.11 | 0.04 | 30.87 | 193.60 | 147.37 | - | 0.18 | 0.01 | - | 13.86 | 38.53 | 6.95 | 0.09 | 0.06 | 0.38 | 152.53 | 0.067 | |
0.5 | 30.83 | 0.04 | 0.01 | 30.75 | 189.12 | 146.53 | - | 0.08 | 0.01 | 27.68 | 12.83 | 39.42 | 5.07 | - | 0.04 | 0.20 | 151.63 | 0.03 | |
0.6 | - | 0.01 | - | - | - | - | - | 0.041 | - | - | - | - | - | - | 0.032 | 0.02 | - | 0.03 | |
0.8 | 30.76 | - | - | 30.48 | - | 144.15 | - | 0.02 | - | - | 12.04 | 37.79 | 3.28 | - | 0.01 | - | 149.70 | 0.02 | |
1 | 30.8 | - | - | 30.37 | 182.75 | 142.15 | - | 0.01 | - | 18.15 | 11.83 | 37.14 | 2.94 | - | 0.01 | - | 148.39 | 0.01 | |
1.3 | 30.71 | - | - | 30.21 | 180.11 | 136.73 | - | - | - | 16.54 | 11.63 | 36.57 | 2.64 | - | 0.01 | - | 145.11 | 0.01 | |
2 | 30.51 | - | - | 30.10 | 175.15 | 127.27 | - | - | - | 15.51 | 11.39 | 35.11 | 2.51 | - | - | - | 139.20 | - | |
3 | 28.21 | - | - | 29.86 | 170.14 | 87.71 | - | - | - | 14.68 | 11.07 | 33.59 | 2.42 | - | - | - | 108.85 | - | |
4 | 24.15 | - | - | 28.56 | 162.29 | 61.38 | - | - | - | 13.26 | 10.75 | 32.32 | 2.37 | - | - | - | 92.47 | - | |
5 | 21.47 | - | - | 24.98 | 155.13 | 45.21 | - | - | - | 12.41 | 10.42 | 30.87 | 2.35 | - | - | - | 79.98 | - | |
10 | 11.54 | - | - | 13.51 | 138.19 | 14.29 | - | - | - | 5.68 | 7.71 | 2.27 | - | - | - | 63.03 | - | ||
15 | - | - | - | - | - | - | - | - | - | 2.31 | 6.13 | - | 2.21 | - | - | - | 58.40 | - | |
20 | - | - | - | - | - | - | - | - | - | 1.22 | 5.34 | - | 2.17 | - | - | - | - | - | |
30 | - | - | - | - | - | - | - | - | - | - | 3.71 | - | 2.03 | - | - | - | - | - | |
40 | - | - | - | - | - | - | - | - | - | - | 2.44 | - | 2.02 | - | - | - | - | - |
Permeability is given in mD; 1 mD = 10−15 m2.
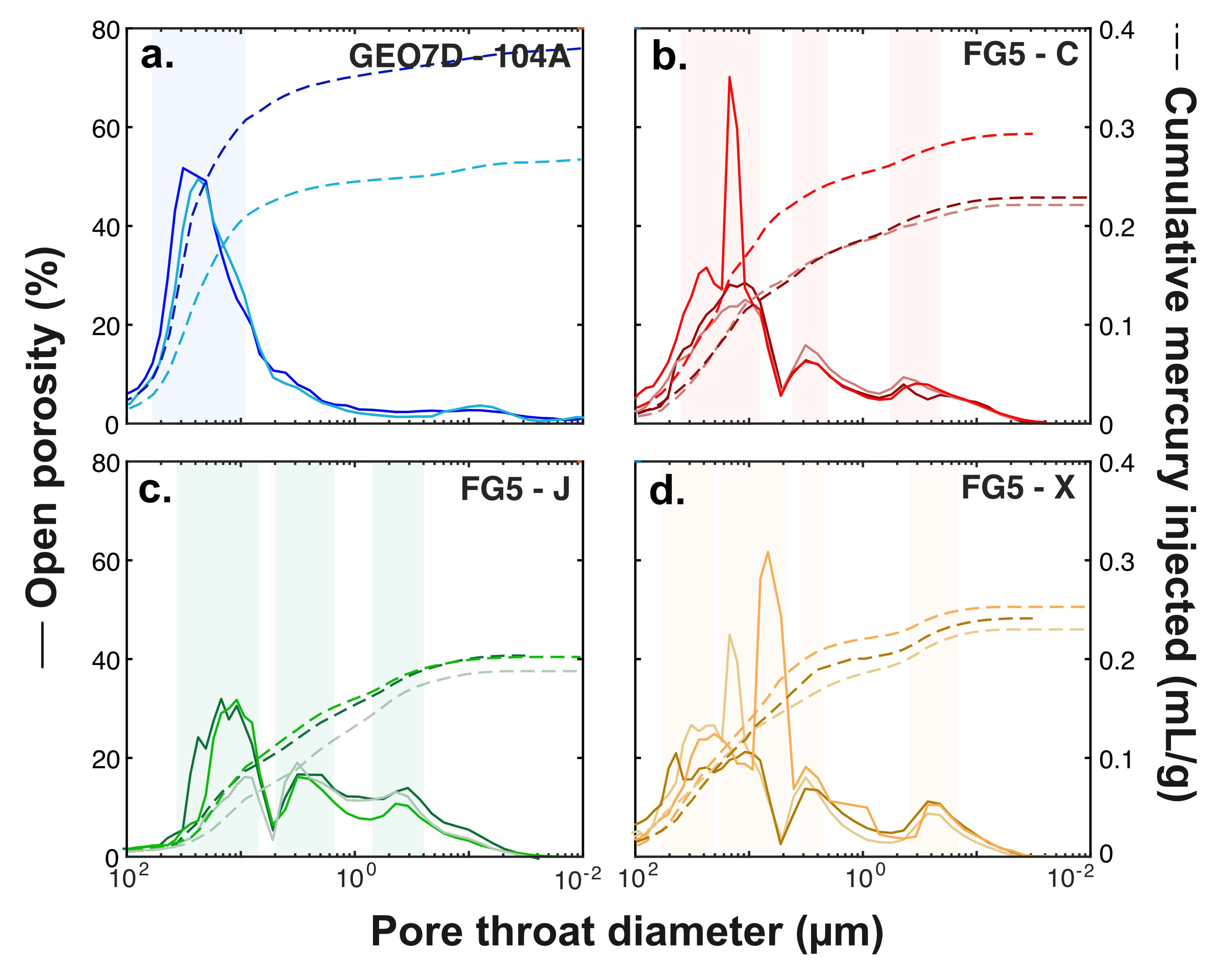
Pore throat diameter as a function of open porosity and cumulative mercury injected (in mL/g) for samples GEO7D-104 (a), and samples of the core FG5 (b–d). Each sample was divided in three sections (each curve—top, middle and bottom for the measurements).
Mercury porosimetry data related to the open porosities of the chalky limestones (related to Figure 4)
Borehole reference | Open porosity (%) | Pore throat diameter (μm) | Bulk density (g/mL) | ||||
---|---|---|---|---|---|---|---|
GEO7D-104A | Top | 54 | 40 | 1.41 | |||
Middle | - | - | - | ||||
Bottom | 43 | 30 | 1.57 | ||||
FG5-C | Top | 35 | 14 | 4 | 0.55 | 1.59 | |
Middle | 36 | 14 | 4 | 0.55 | 1.58 | ||
Bottom | 47 | 19 | 4 | 0.43 | 1.61 | ||
FG5-J | Top | 41 | 19 | 3 | 0.43 | 2.02 | |
Middle | 34 | 14 | 4 | 0.55 | 1.69 | ||
Bottom | 28 | 12 | 4 | 0.55 | 1.51 | ||
FG5-X | Top | 40 | 40 | 19 | 4 | 0.34 | 1.57 |
Middle | 37 | 55 | 14 | 4 | 0.34 | 1.62 | |
Bottom | 39 | 25 | 9 | 4 | 0.34 | 1.61 |
2.3. Why chalky limestones have been chosen for compaction experiments compared to other lithologies: a singularity revealed by …
2.3.1. A (critical) weakness revealed by uniaxial compressive strength tests
UCS experiments were conducted on dry samples of 40 mm in diameter and 80 mm in length to constrain the intrinsic strength of Mururoa’s rocks, in the absence of confining pressure (Figure 2b, Table 1). The volcano-basaltic bedrock and the dolostones are amongst the most resistant rocks in the sequence, in contrast to the chalky limestones (FG5-R and FG5-L), which appear to be the weakest rocks by far. The UCS experiments provide strong support to target this lithology for the experimental investigation described below.
2.3.2. Very specific intrinsic properties (dry bulk density, permeability, connected and open porosities)
Chalky limestones are found as the most porous rocks in Mururoa: these properties are unique with regard to the other rocks of the lithostratigraphic sequence (except for basaltic sample 138D which is found as porous as chalky limestones; see Figure 3a). The dry bulk densities of chalky limestones are in the range of 1.4–1.6. Their connected porosities are measured between 28 and 54%. In details, chalky limestones are found in a very porous form (connected porosity greater than 35–45%, sample 104A) or a slightly more consolidated form (connected porosity less than 30%, sample 105E). At 0.1 MPa, their initial permeabilities are in the range 101–102 mD (1 mD = 10−15 m2). The permeability of chalky limestones appears to exhibit a strong correlation with a power law in 𝜙2.
Open porosity data have been collected using mercury porosimetry for several samples of chalky limestones (Figure 4). Each sample was divided in three sections for the measurements (except for the sample GEO7D-104A). The pore distribution is heterogeneous depending on the cores, can be founded as unimodal for the only core sampled in borehole GEO7D (104A), trimodal (FG5-C and J) and quadrimodal (FG5-X) for samples sampled in the borehole FG5. This disparity can be explained by (i) the localization of the boreholes and (ii) the drilling period (2016 for GEO7D vs. 1980 for FG5 borehole, with the possibility of alteration over the 40 years of storage at CEA-DAM). The mean throat pore diameter of chalky limestones can be found at one of four mean ranges, depending on the geometry of the pore distribution. These ranges are 0.3–0.5 μm, 3–4 μm, 9–19 μmor 25–55 μm. This heterogeneous pore distribution is explained by the particular and heterogeneous microstructure of chalky limestones.
The relationship between the evolution of permeability as a function of confining pressure is shown in Figure 3b. One can clearly distinguish two families:
- a first family that exhibits little pressure dependence (at least at pressures below the pore collapse pressure P∗), including chalky limestones, samples from the transition zone (samples 116B, 119A, 120A, 121A) as well as some massive and upper limestones (samples 2A and 66B). The permeability of these rocks is constant from 0 to 2 MPa, then abruptly decreasing above a critical pressure (P∗) which may be as low as 4 MPa (except for transition rocks for which a gradual decrease in permeability is observed at a lower value of 0.3 MPa—sample 121A).
- a second family for which permeability is strongly pressure dependent, including massive limestones, basalts excepting clayey and very altered basalts (as sample 138D) and indurated dolomites. For these rocks, the minimum permeability measurement threshold (approx. 1 μd) attainable with our system was reached for confining pressures ranging between 0.4 and 1.3 MPa.
2.3.3. A fine microstructure formed by equilibrium between mineralogy and marine bio-skeletons
Chalky limestones are composed of calcite (CaCO3), dolomite (CaMg(CO3)2) with some local traces of halite (NaCl). The grain size ranges from 10 to 100 μm. As shown by scanning electron micrographs prior to experiments (Figure 5a,b), chalky limestone is composed of planktonic skeletons (such as foraminifera and coccolithophores) and larger marine organisms such as mollusks, echinoderms, or bryozoans. The presence of dolomite has been related to a dolomitization reaction during the history of atoll formation [Aissaoui et al., 1986].
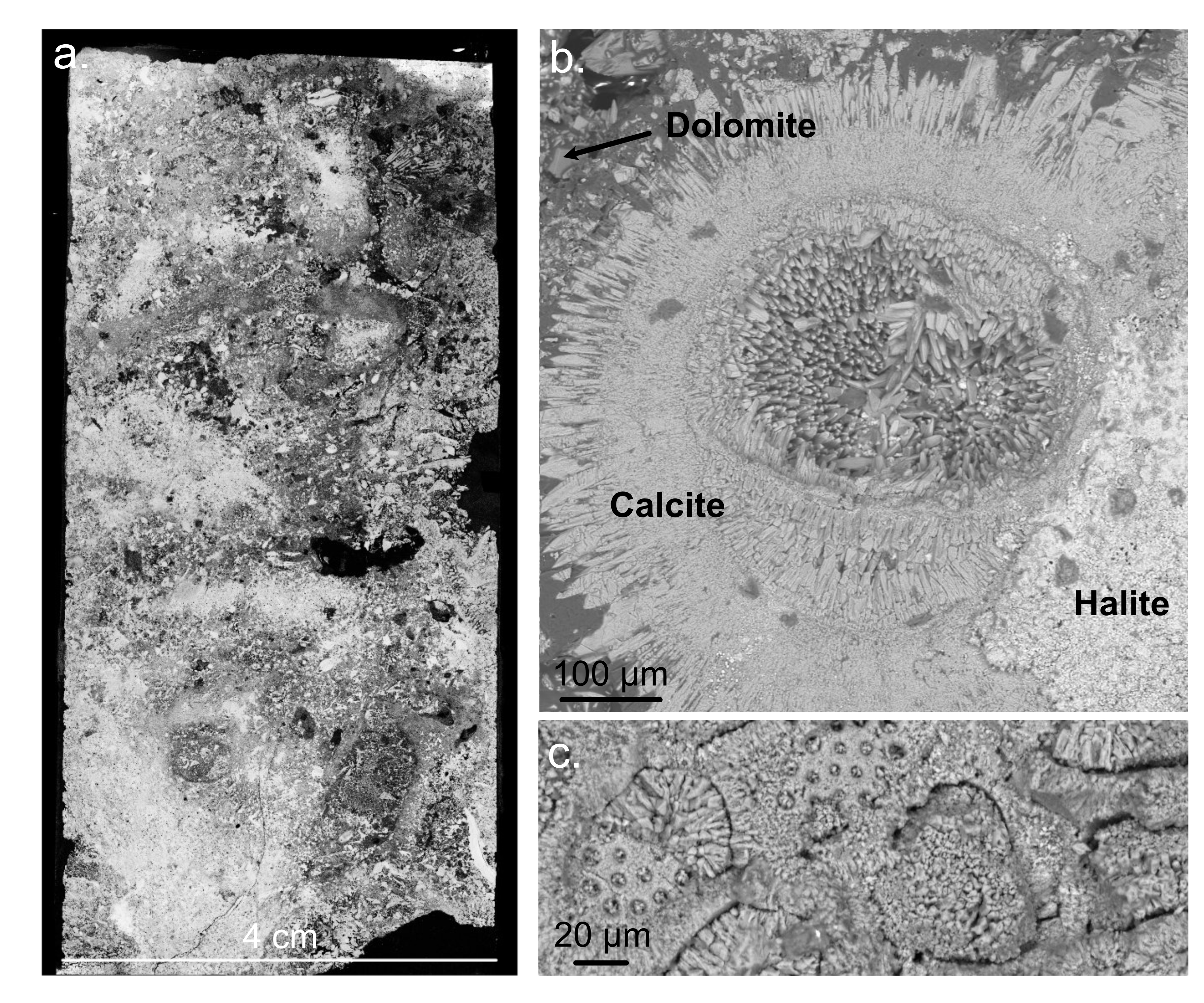
SEM micrographs and composition of chalky limestone. (a) SEM micrographs of a sample before the experiment. (b) Mineralogical composition of chalky limestones. (c) Bio-construction and marine fossil skeletons of the chalky limestone matrix.
2.4. Sample preparation and experimental procedure for mechanical deformation
Cylindrical samples 40 mm in diameter and 80 mm in length were cored from selected samples from two boreholes (GEO7D and FG5) drilled at Mururoa (Figure 1). The samples were cut and drilled and then placed in a heat chamber at a temperature of 50 ± 2 °C for 72 h. Due to the high rate of breakage during drilling, only 23 out of more than 80 chalky limestone samples from the original cores were used for the experiments. These samples were taken from 7 in GEO7D core and 16 in FG5 core.
The samples were deformed at room temperature using a triaxial oil loading press [Laboratoire de Géologie de l’École Normale Supérieure, Paris, France; Aubry et al., 2018, 2020, Fortin et al., 2005, Schubnel et al., 2007]. Ready for testing, the sample was placed in a 8 mm thick jacket (Viton Rubber FKM B, EFB75CS, Elastomer Engineering) to isolate it from the oil confining fluid. Strain gages were not used in the experiments. All initial tests resulted in strain gage breakages even when the pores in surface were filled with epoxy and polished to improve adhesion. Pore volume change was monitored using a micro-volumetric syringe pump (Quizix 10K, Laboratoire de Géologie de l’École Normale Supérieure, Paris, France), in order to observe the compaction of the rock on the water-saturated specimen only. Post-mortem specimens were impregnated with mounting resin and finally cut in half and polished for microstructural analysis.
Hydrostatic and deformation experiments were carried out according to a three-stage experimental plan:
- Hydrostatic compression tests were performed on chalky limestones only (GEO7D and FG5 samples), under drained water saturated conditions, to determine the critical pore collapse pressure P∗. These experiments consisted of first saturating the chalk sample for 24 h and then gradually increasing the confining pressure up to 90 MPa (±0.1 MPa) at a loading rate of 0.01 MPa/s. The pore fluid pressure was constant at 9 MPa ± 0.05 MPa—the maximum pore fluid pressure that can be applied to the Quizix pump—in all experiments except one which was carried out at 2 MPa ± 0.05 MPa. The compaction ΔV/V is measured during the experiments with the compressibility of grains being disregarded. Consequently, it is simply the change in porosity that is observed.
- Triaxial deformation tests have been carried out on water-saturated chalky limestones (FG5 samples) at effective pressures between 2 and 10 MPa. These experiments consist of saturating the sample with water for 24 h at constant pore pressure, then deforming the sample at a constant axial deformation rate, at a constant pore pressure of 2 MPa (chosen for allowing us to perform long-time experiments without stopping or re-filling the Quizix pump in water before the end of the experiment). The axial shortening rate was fixed at 10 μm/s (i.e. a strain rate of 10−5/s).
- In addition, triaxial deformation tests were carried out on dry chalky limestones (FG5 samples), at confining pressures between 2 and 10 MPa to investigate possible water-related weakening. C* was determined with stress–strain data.
3. Results: compaction and pore-collapse experiments
In the following, compressive stresses, shortening and volumetric compaction are considered positive. Compaction is denoted ΔV/V . Axial and radial stresses are denoted 𝜎1 and 𝜎3, respectively. The difference 𝜎1–𝜎3 is defined as the differential stress. The effective pressure Peff, is defined as the difference between the confining pressure Pc and the pore fluid pressure Pp: Peff = Pc − 𝛼Pp where 𝛼 is the effective stress coefficient. The effective mean stress Pm is calculated as (𝜎1 + 2𝜎3)/3 − Pp.
3.1. Experiments under hydrostatic condition
The pore collapse of chalky limestone is studied according to three initial porosities (Figure 6a) and two different pore pressures (Figure 6b). Whatever their initial porosity (26, 38 and 42%), the critical pressure of pore collapse P∗ was attained at effective pressure ranging between 16 and 18 MPa, i.e. pressures equivalent to an approximate depth of >1 km (assuming hydrostatic conditions and an average solid density of 2500 kg/m3). Their initial porosity has an influence on the mechanical behavior for pressure higher than P∗. Figure 6b report two hydrostatic experiments performed on chalky limestones of the same porosity (40%), but at different pore pressure (at 2 or 9 MPa). For FG5 samples, P∗ was reached at 11 and 14 MPa, for the experiments at pore pressures of 2 and 9 MPa, respectively—at lower P∗ values than for GEO7D samples. Figure 6b shows that the evolution of ΔV/V during Peff increase and the position of P∗ were not affected to a great extent by the change in pore fluid pressure (meaning that 𝛼 is close to 1).
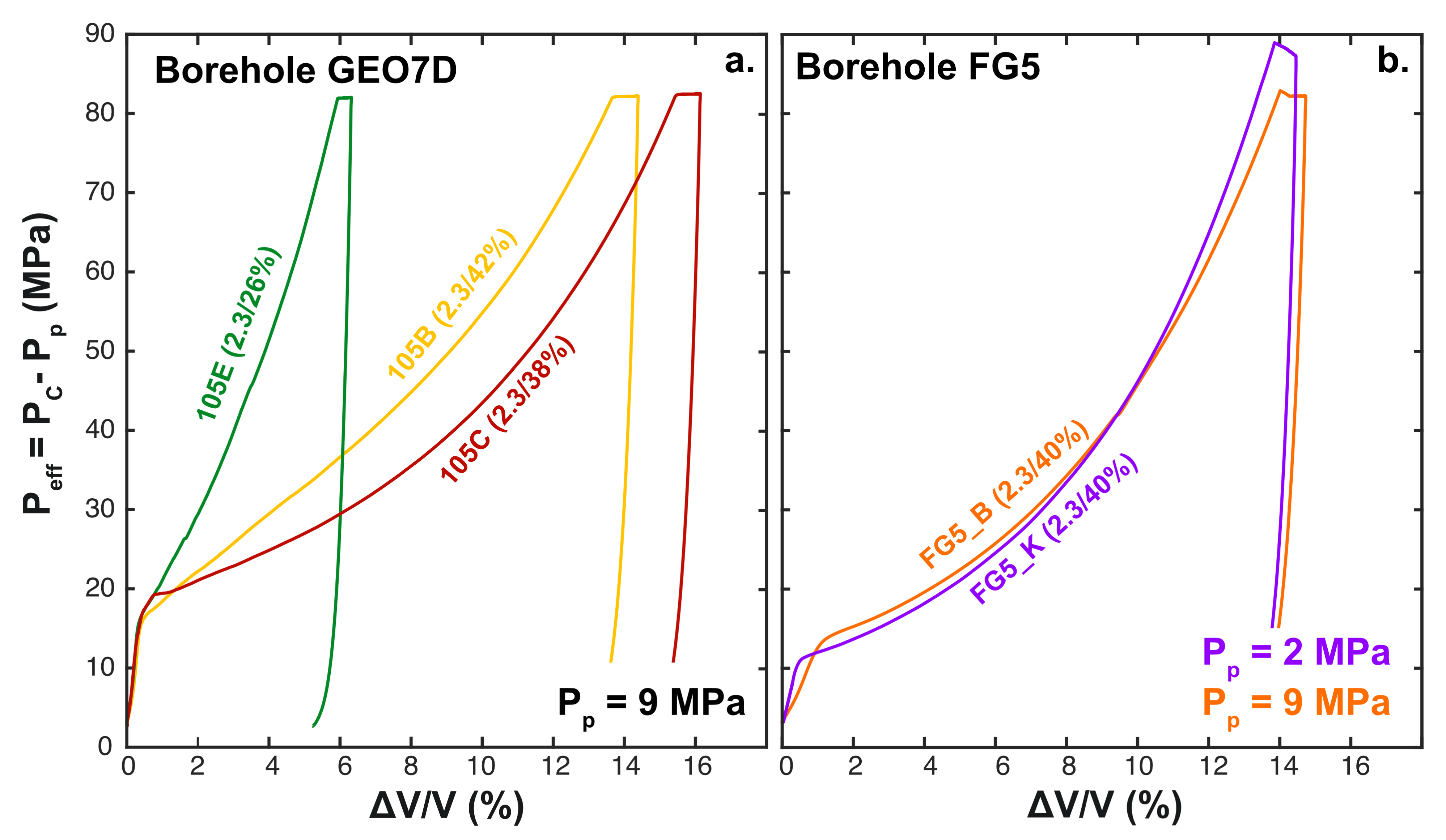
Effective pressure as a function of compaction. (a) For 3 chalky limestones from the GEO7D borehole with different porosities at a pore pressure of 9 MPa. (b) For two chalky limestones from the FG5 borehole at pore pressures of 2 and 9 MPa.
3.2. Triaxial deformation experiments in water-saturated and dry conditions
During triaxial deformation, samples exhibit an elastic loading followed by shear-enhanced compaction at all the effective pressures tested (Figure 7). Compaction ranged from 3% at 2 MPa of effective pressure to almost 10% at 10 MPa of effective pressure. Above 6 MPa of effective pressure, compaction was associated with strain hardening (Figure 7—top), for axial deformation up to a maximum of 30%. Strain weakening was only observed at the effective pressure of 2 and 4 MPa. At 2 MPa of effective pressure, a few stress drops were associated with an acceleration of the volumetric strain or compaction—not at 4 MPa. In consequence, our mechanical investigation suggests that strain-weakening (i.e. stress release) may occur in chalky limestone, but below an effective pressure of 4 MPa (i.e. an approximate depth of 250 m, using the same assumptions as above).
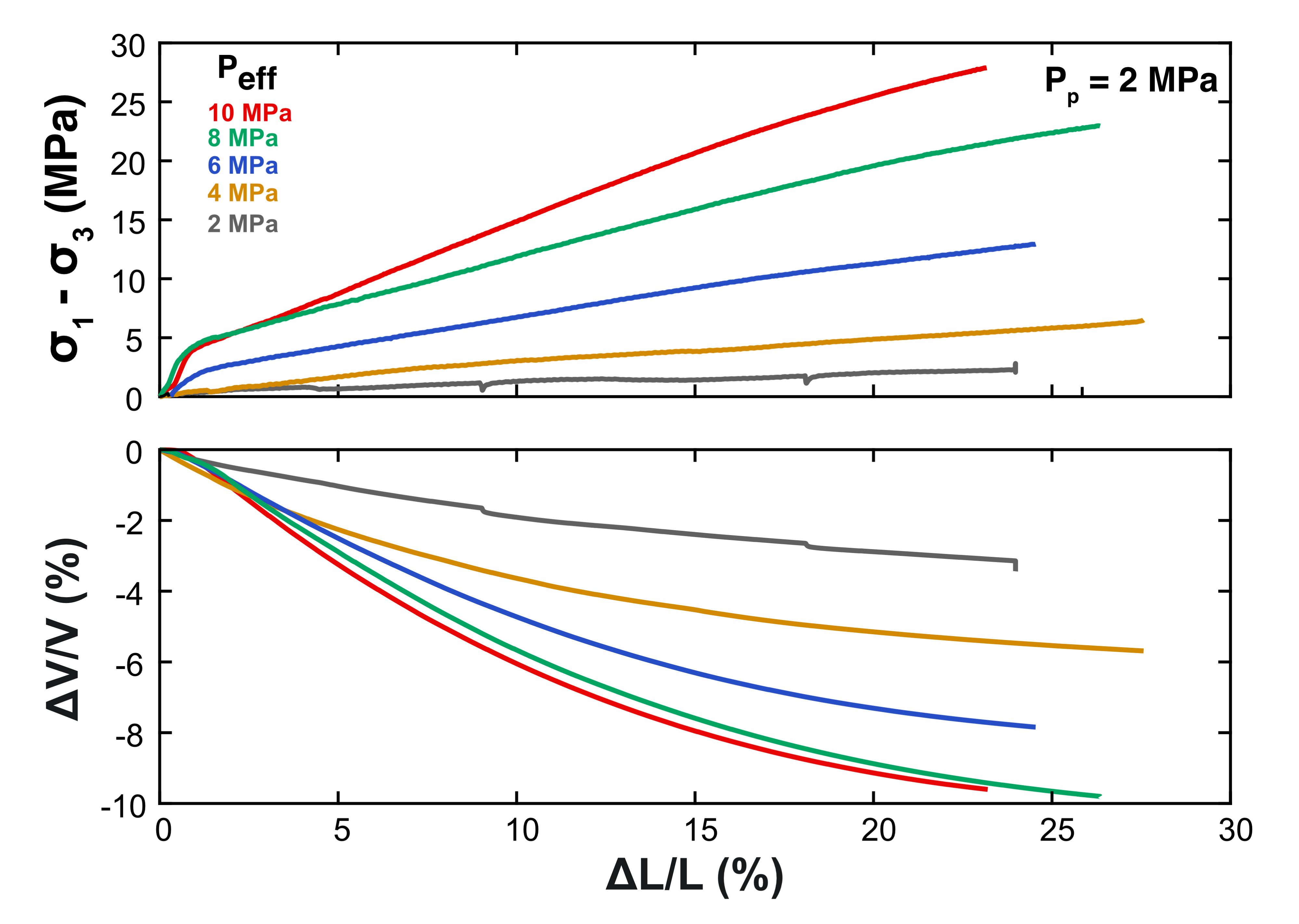
Mechanical data for water-saturated deformation tests. Differential stress and compaction as a function of axial deformation for water-saturated chalky limestones between 2 and 10 MPa (depending on color) at a constant pore pressure of 2 MPa.
Volumetric strain data are not available for the dry samples, as strain gages were not used in these experiments. Overall, the mechanical data for the dry and wet experiments are qualitatively the same. Yet, for effective pressure below 8 MPa the yield stresses appear largely reduced in the water-saturated experiments (Figure 8). Above 8 MPa of effective pressure, yield stresses are found to be in the same range than for dry experiments. In dry condition, a stress release appears to occur after the yield stress is reached during the experiment at 2 MPa, like in the water-saturated case.
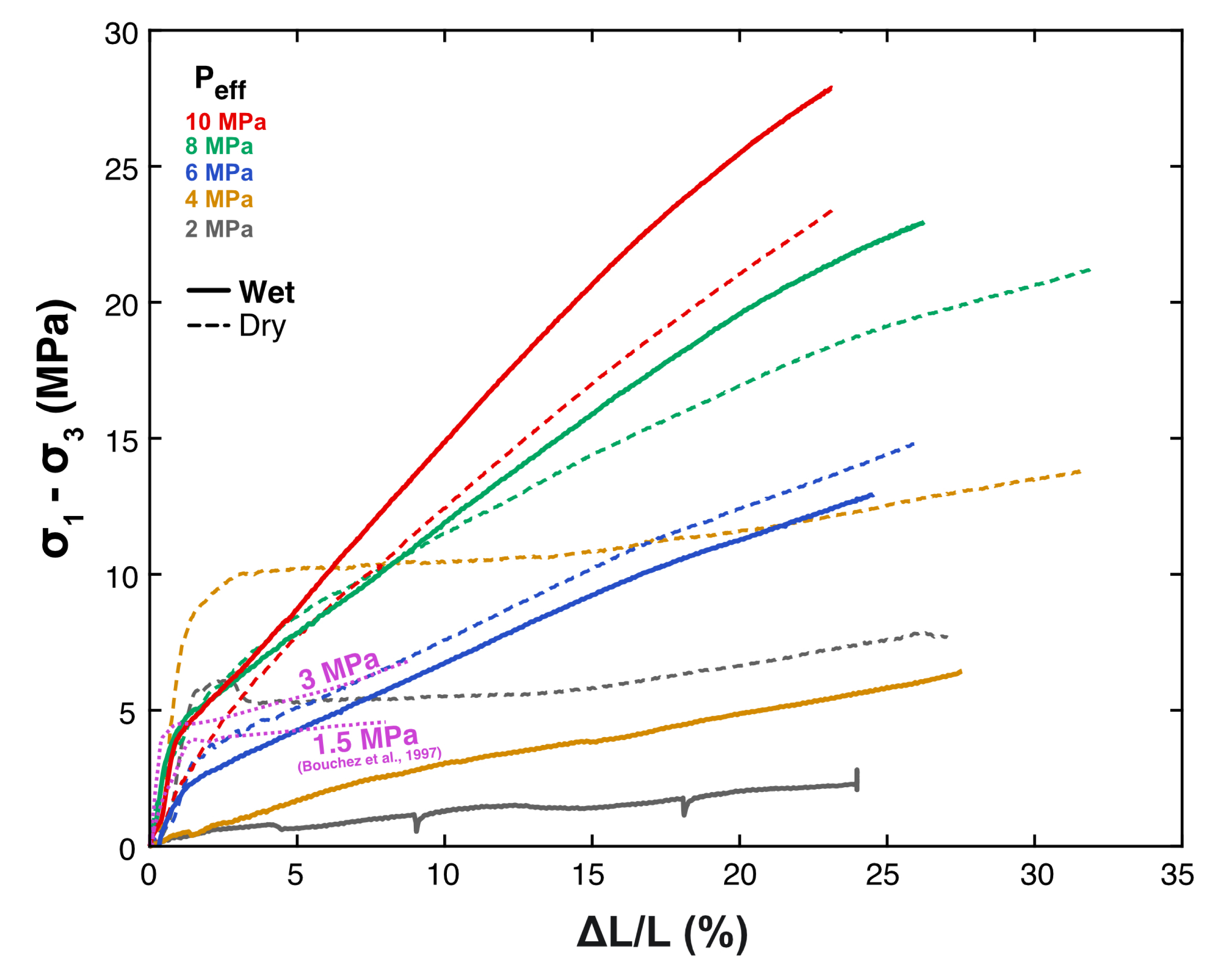
Compilation of water saturated and dry experiments. Differential stress as a function of axial deformation for water-saturated and dry chalky limestones between 2 and 10 MPa of effective pressure, in water-saturated and dry conditions (color and line thickness codes). The wet experiments were carried out at a pore pressure of 2 MPa. The mechanical data are compared with the only laboratory geomechanical data published to date on the Mururoa chalky limestones [in pink; Bouchez et al., 1997]—at low confining pressures (1.5 and 3 MPa) and at strain rates from 10−11 to 10−9 s−1. Masquer
Compilation of water saturated and dry experiments. Differential stress as a function of axial deformation for water-saturated and dry chalky limestones between 2 and 10 MPa of effective pressure, in water-saturated and dry conditions (color and line thickness codes). The wet ... Lire la suite
3.3. Microstructures on post-mortem samples
Samples deformed under wet conditions were difficult to impregnate with epoxy resin, and are therefore not shown in the study. SEM micrographs of samples deformed at 2 and 10 MPa of confining pressure in dry conditions are shown in Figure 9. Scanning-electron micrographs of the sample deformed at 2 MPa effective pressure shows several areas of disaggregated and crushed material (Figure 9a). At 10 MPa of effective pressure, deformation is enhanced by shear-enhanced compaction. The compaction is not homogeneous and seems to depend on the initial microstructure (Figure 9b).
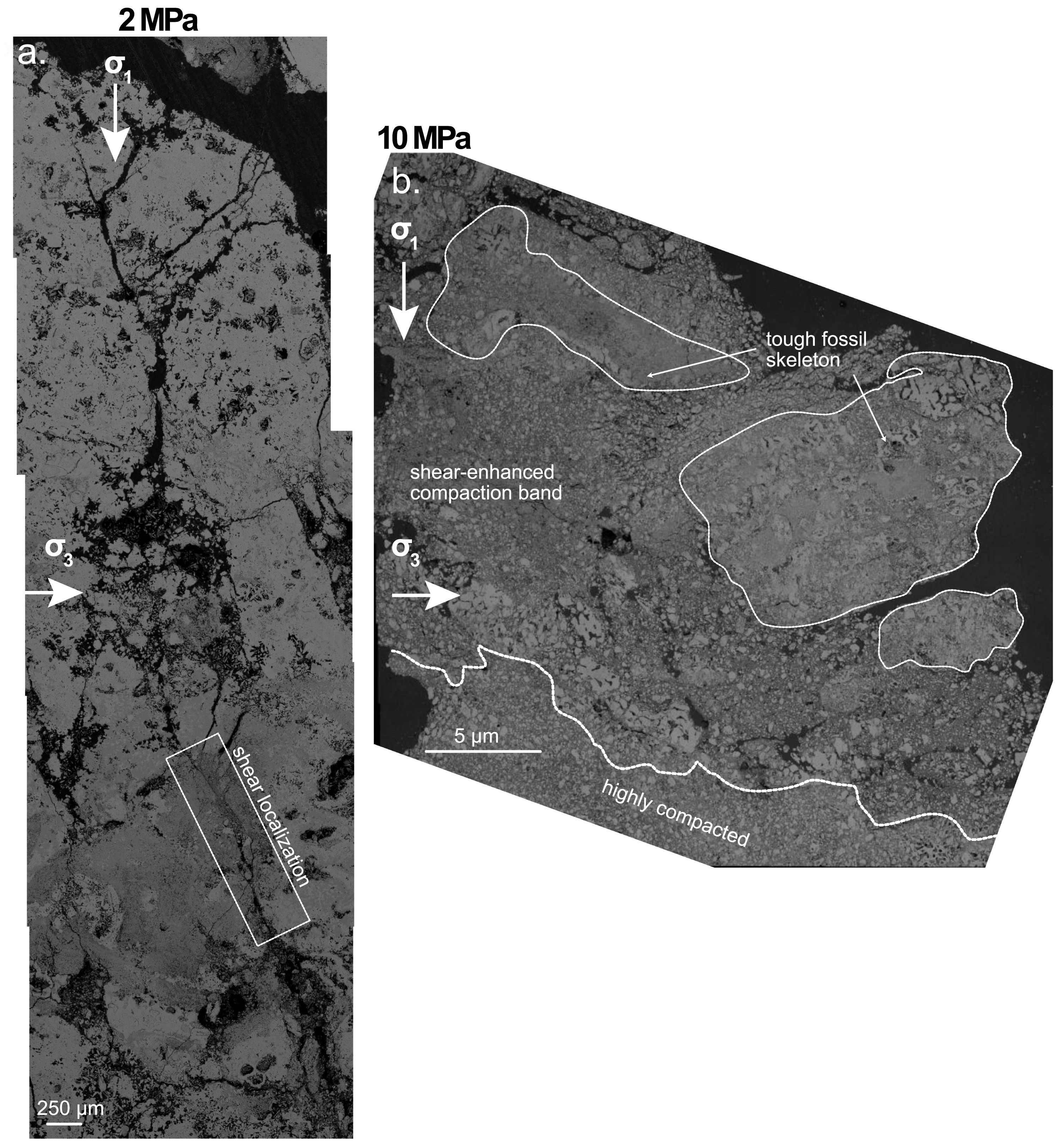
SEM on a sample deformed at 2 MPa (a) and 10 MPa (b) of confining pressure in dry condition.
4. Discussion–conclusion
The permeability measurements under confining pressure show two families of rocks (Figure 3), with the ease with which water can pass through depending, or not, at the depth of burial of the rock. This dichotomy can be explained by the structure and the initial micro-fracturing state of the different rocks: rocks with low permeability, or whose permeability is highly dependent on the confining pressure (Figure 3), appear as rocks with micro-fractures in a dense matrix with small grain size and low porosity. Permeable rocks, for which the permeability is not dependent on pressure, are the more porous rocks with numerous millimetric or micrometric voids and interstices, visible even on the surface of the sample. The fact that this second family of rocks does not show a permeability-pressure dependency means that the voids are connected. In the first family the connectivity is controlled by the microcracks which explain the pressure sensitivity [Vinciguerra et al., 2005].
Initial porosity plays a role in the peak stress at failure in the brittle regime and for the onset of shear-enhanced compaction (Figure 10). Chalky limestones are porous, permeable and weak rocks with a high compaction capacity compared to results reported in the literature on other porous rocks (Figure 10), including chalks and limestones deformed in the laboratory under similar conditions [Baud et al., 2009, 2017b, Geremia et al., 2021, Xie et al., 2011]. They appear to be compactant and mechanically unstable (Figures 2–5), with mechanical properties close to those of Obourg chalk [Geremia et al., 2021; Figure 9] and P∗ close to 16–18 MPa under water saturation (Figure 5). Under hydrostatic conditions, the influence of pore pressure seems slightly or just as important as the initial porosity (Figure 6a,b). The microstructural heterogeneity of chalky limestones can be explained by their deposition conditions, the variations in sea and phreatic levels [Fabricius, 2007] and the mechanical forcing and relative compaction induced by the past nuclear tests.
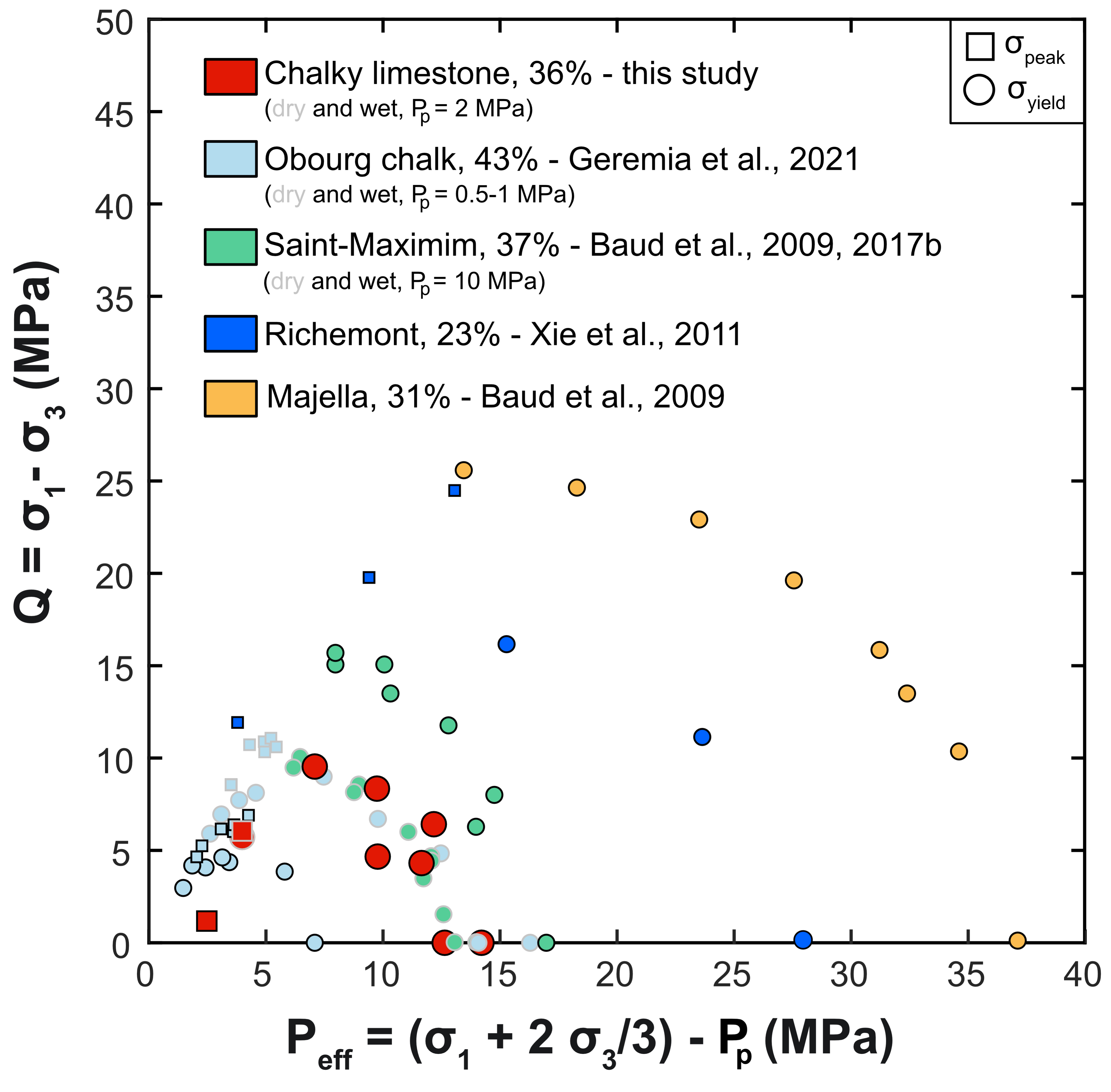
Comparison of the experimental results with a compilation of mechanical yield envelopes for chalky limestones and porous rocks with low critical pore collapse values.
Within the atoll, water transfers between the lagoon and the ocean, and through the internal structure of the atoll, are influenced by a geothermal flux [Rougerie and Wauthy, 1986], are conducive to fluid overpressures that affect the strength of less consolidated rocks, and facilitate localized gravity collapses or landslides [Keating and McGuire, 2000, Viesca and Rice, 2012]. In the context of a crown open to the ocean, karstified or poorly consolidated rocks are also affected by erosion, dissolution or hydrolytic alteration processes that contribute to their progressive weakening [Parise and Lollino, 2011]. The transient displacement measured in the north-east zone of the atoll could be explained by the localization of the deformation under the effect of a progressive compaction and a local hydrological forcing to overpressure in the most porous and permeable levels of the stratigraphic sequence, including in chalky limestones.
Although not directly dedicated to the study of the geomechanical deformation of atolls, several experimental studies discuss the influence of water on the mechanical behavior for a better understanding of the petroleum reservoirs mechanics. These studies show that the weakening effect of water is associated to a decrease in Young’s and bulk moduli, pore collapse yield stress and cohesion with increasing water saturation [Amour et al., 2021, Foged et al., 1996, Papamichos et al., 1997, Risnes et al., 1996, Schroeder and Shao, 1996]. Foged et al. [1996] also showed an increase in the rate of deformation with increasing initial water saturation.
In the brittle regime and in the presence of water (Figure 7), chalky limestones failed at low effective pressures (2 MPa). Failure occurs through microcracking and shear localization as in others lithologies referenced in the literature (Figure 10). The water-weakening effect can be explained by a reduction in surface energy and fracture toughness [Geremia et al., 2021, Noël et al., 2024], dissolution and pressure solution [Newman, 1983], bond rupture at crack tips [Parks, 1984] and a reduction in capillary forces [i.e. between solid grain surface and water; Brignoli et al., 1994, Papamichos et al., 1997, Risnes and Flaageng, 1999].
Above an effective pressure of 4 MPa, microcracking and pore collapse disappear in favor of hardening accommodated by shear-enhanced compaction and grain-crushing (Figure 8), except in some areas where the stiffer microfossil shells remain unaffected (Figure 9). Our experiments also show that water has no effect on compaction above an effective pressure of 6 MPa, indicating that the yield strength is almost the same for dry or wet experiments at higher effective pressures. This is consistent with the results reported by Nicolas et al. [2017] which showed a water weakening effect in the brittle regime of Tavel limestone (a rock with a porosity of 14%, less porous compared to chalky limestones), but no effect on the yield strength at the onset of compaction. Compared to the Obourg chalk [Geremia et al., 2021], no localization markers are found in the post-mortem water-saturated samples. This phenomenon can be attributed to the fact that water-saturated samples deformed by more than 20% of axial deformation are completely disaggregated, whatever the important structural heterogeneity of chalky limestones. In order to gain a more comprehensive understanding of this process, it would have been beneficial to conduct systematic microstructural analysis before and after the experiments, particularly with X-ray computed tomography.
In summary, chalky limestones exhibit a transition from microcracking at extremely low effective pressures to shear-enhanced compaction, associated with pore collapse and grain-crushing at higher effective pressures. This result, obtained after a substantial axial deformation, has significant implications for the long-term stability of the Mururoa atoll rim, as well as for the geomechanics of several other chalk environments.
Declaration of interest
The authors do not work for, advise, own, or receive funding from any organization that would benefit from this article, and have declared no affiliations other than their research organizations.
Funding
This work was supported by the LRC Yves Rocard (Laboratoire de Recherche Conventionné CEA-ENS-CNRS).
Acknowledgements
We thank J.-P. Avouac, P. Pras and A. Loevenbruck for fruitful discussions about the project. C. Lallemand and A. Loevenbruck help us in providing the samples drilled in Mururoa.