1 Introduction
The rocky planets (Mercury, Venus, Earth, and Mars), their satellites, and the large bodies from the asteroid belt each have distinct mineralogical and geophysical identities. They differ by the presence, or not, and volume of a core, their mineralogical nature, the thicknesses of their crust and mantle, the existence, or not, of a liquid ocean and of an atmosphere, not to mention the existence of life. One of the most striking contrasts is between the Earth, with its liquid ocean and granitic continental crust, on the one hand, and the bone dry Moon, with a lithosphere enriched in plagioclase, on the other hand. With respect to the overwhelming homogeneity of the Solar System, which harbours the elemental and isotopic composition of chondrites (the material dominating the asteroid belt), in particular for the less volatile elements, such a prominent variability of the most visible features of the rocky planets calls for a strong first-order explanation. There is very little geochemical information on Mercury and Venus. The information on the composition of Mars as obtained from Martian meteorites is fragmentary and controversial, notably for the lack of a useful chronological perspective, while the spectrometric data obtained from the orbiters are imprecise.
This paper will focus on the identification of a common thread that can account for the geochemical, mineralogical, and chronological evolution of differentiated rocky planets with emphasis on the Earth, Moon, Mars, and the parent body of eucrites, for all of which samples are available. It will be shown that most of the modern mineralogical and dynamical features of these planets are related to the size of each planet and therefore to its capability of retaining light elements, notably water, and to the distance of each planet from the Sun. We will demonstrate that much of the early history of each planetary body, in particular the existence of a magma ocean stage, and its dynamic regime, such as plate tectonics on Earth, can be explained successfully in this way.
After first reviewing the effect of gravity and water content on the nature of minerals crystallizing from liquid silicates, we will address the relationships between the size of an accreting planet, its gravity, and its thermal evolution. Gravity and water content affect the early thermal stages, especially the presence and evolution of a magma ocean.
2 The effect of gravity and water content on magmatic differentiation
In a medium of density ρ in hydrostatic equilibrium, pressure P and acceleration of gravity g at depth z are related by the simple relationship:
Pressure affects the stability field of compressible mineral phases and, by far, its most important effect is on plagioclase, a Ca–Na feldspar present in many basaltic rocks and which has a very large compressibility. Fig. 1 uses experimental data to outline the stability fields of the major minerals of anhydrous basalt and emphasizes a crucial point of planetary differentiation, which is the cross-over between plagioclase and pyroxene at ∼0.5 GPa [43,48]. A cooling anhydrous basaltic magma will first experience saturation of olivine regardless of pressure. At pressures below ∼0.5 GPa, olivine will be followed by plagioclase, which saturates before pyroxene, whereas at pressures >0.5 GPa, pyroxene will saturate before plagioclase, which appears only much later during magma solidification.
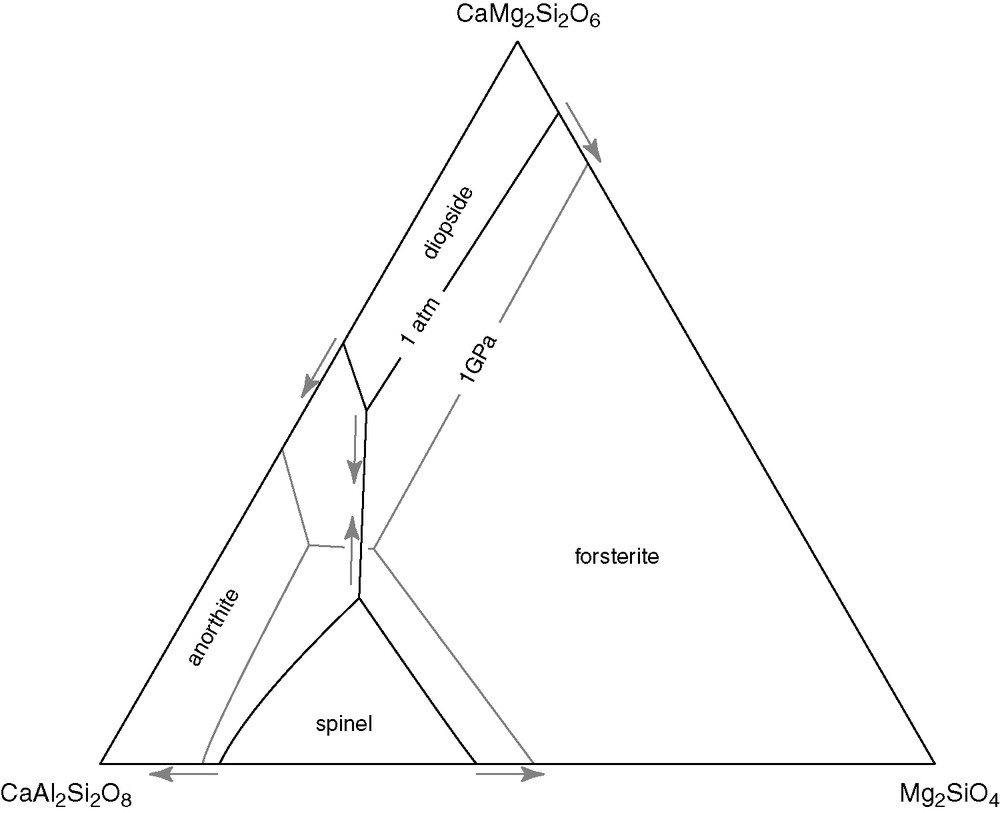
Effect of pressure (arrows) on the stability of minerals at the liquidus of a simplified basaltic melt composition [43]. From ambient pressure to 1 GPa, the stability fields of anorthite (plagioclase) and forsterite (olivine) shrink, whereas the field of diopside (clinopyroxene) expands. Most natural compositions plot next to the triple point between anorthite, forsterite, and diopside.
Fig. 1. Effet de la pression (flèches) sur la stabilité des minéraux au liquidus d’une composition basaltique simplifiée [43]. Les champs de stabilité de l’anorthite (plagioclase) et de la forstérite (olivine) se rétrécissent, quand la pression augmente entre la pression atmosphérique et 1 GPa, alors que le champ de stabilité du diopside (clinopyroxène) se dilate. La plupart des compositions naturelles se placent près du point triple entre anorthite, forstérite et diopside.
The density and bulk modulus of the relevant mineral phases [6] are shown in Table 1. Two key features emerge from these data: plagioclase is the only mineral less dense than the melt and plagioclase is the most compressible mineral, thus making it highly sensitive to pressure: diminishing the pressure will expand the molar volume of the plagioclase component thereby rendering it more insoluble. At low pressure, cooling magmas quickly exsolve buoyant plagioclase, which floats to the surface, whereas at higher pressures, olivine and pyroxene precipitate and sink to the bottom of the liquid mass.
Density (ρ) and bulk modulus (K) of common minerals and melts [6]
Tableau 1 Densité (ρ) et module de compressibilité (K) de minéraux communs et fondus [6]
ρ (kg m−3) | K (GPa) | |
Olivine | 3300 | 130 |
Clinopyroxene | 3350 | 115 |
Garnet | 3700 | 95 |
Plagioclase | 2650 | 65 |
(Ultra)mafic liquid | 2900 | 18 |
Serpentine | 2550 | |
Talc | 2700 | |
Anthophyliite | 2800 |
An additional effect of gravitation on magmatic differentiation is that of mineral segregation from liquids through Stokes’ law:
The role of water on magmatic differentiation is not less crucial than that of gravity. Plagioclase is a framework silicate in which each oxygen atom forms a double bond between either Si and Si or Si and Al. The effect of water on plagioclase solubility is related to its role as a bond-breaking agent with respect to the molten silicate framework. The ‘silanol’ reaction:
3 The effect of planetary size on gravity and the initial thermal state
The acceleration of gravity varies by a very large amount from one planet to another and this is a critical factor in controlling the pressure gradient within any given planet and the mineralogy of its different layers (core, mantle, crust). The acceleration of gravity g is related to the force F on the mass m exerted by a planet of mass M at distance r and is defined by:
On average, gravity increases linearly with the radius of a planet: because of a radius about four times smaller than that of the Earth and a mean density 40% lighter, the Moon has a gravity field only 1/6 that of the Earth and therefore an internal pressure gradient much smaller than its terrestrial equivalent.
The size of a planet also determines the gravitational energy released upon accretion of an infinitesimal shell of material with density ρ. Impacts are just a particular form of accretion. Gravitational forces are the negative derivative of the gravitational potential U, and therefore U = −G M/r. We assume that the planet before this new addition has a mass M. The energy released, assuming a constant density r, is:
This energy is converted into kinetic energy of opposite sign and, upon impact, into thermal energy. The time-independent increment ΔT, such as dEP = dm CP ΔT, which determines the gravitational heating of the accreted material is:
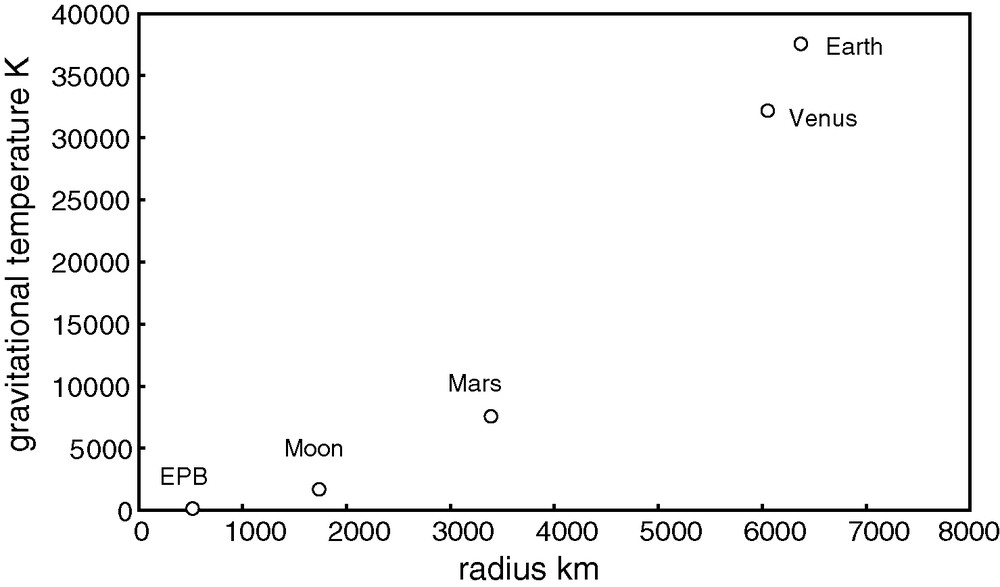
Maximum heating temperature of planetary bodies (EPB = Eucrite Parent Body, Vesta?) by release of gravitational energy (impacts) in the absence of surface radiation.
Fig. 2. Température maximum atteinte par un corps planétaire (EPB désigne le corps parent des eucrites, Vesta ?) grâce à la dissipation de l’énergie gravitationnelle (impacts) en l’absence de pertes de surface par radiation.
In addition to the gravitational energy corresponding to the accretion of undifferentiated material, the heat released by the segregation and differentiation of the core [20,53] also must be taken into consideration. Solomon [53] estimates that formation of the planetary cores, which took place within 30 Myr of planetary accretion, released enough heat to warm up the Earth by 2300 K and Mars by 300 K, but left the Moon's inner temperature nearly unchanged, reflecting the small size, or complete absence, of the Moon's core.
Gravity also changes the adiabatic gradient in the planetary interiors according to:
4 The rocky planets accreted dry
The standard theory of the Earth holds that the ocean and the atmosphere formed at an early stage by degassing of the mantle. Let us show, however, why this assertion is most certainly incorrect by using a simple comparison between two elements with well-understood properties, K and U. Potassium is an alkali element and, like all alkali elements (Li, Na, K, Rb, Cs), is highly volatile. In particular, it is found in measurable quantities in the vapour emitted by lava lakes. Lodders [34] assigns K a 50% condensation temperature in the Solar System of 1001 K. In a milestone paper, Gast [24] demonstrated that the loss of most of potassium's close relative Rb during accretion of the Earth could be seen in the very low terrestrial abundance of the 87Sr isotope, which is the decay product of the radioactive 87Rb nuclide. In contrast, U is very refractory and Lodders [34] assigns this element a condensation temperature of 1610 K. These contrasting properties between K and U can be seen in the K/U ratio of each planet: with respect to a K/U ratio of 60,000 in unprocessed chondrites, whether carbonaceous or ordinary [40], and the solar photosphere, this ratio is 10,000 in the Earth, 6000 in Mars, and 3000 in the Moon [54]. In other words, assuming that U was left untouched by accretion, the Earth kept only 10,000/60,000 = 17% of its K, Mars 10%, and the Moon 5%, which indicates that the concentration of volatile elements was not constant in planetary materials across the inner Solar System. This begs the question of how much gas and water can be expected to have been preserved in a body that lost such a huge proportion of an element volatile at 1000 K? The answer is: essentially none!
This strong assertion can be tested even further. Condensation of an element from a vapour phase in general follows Henry's law such as:
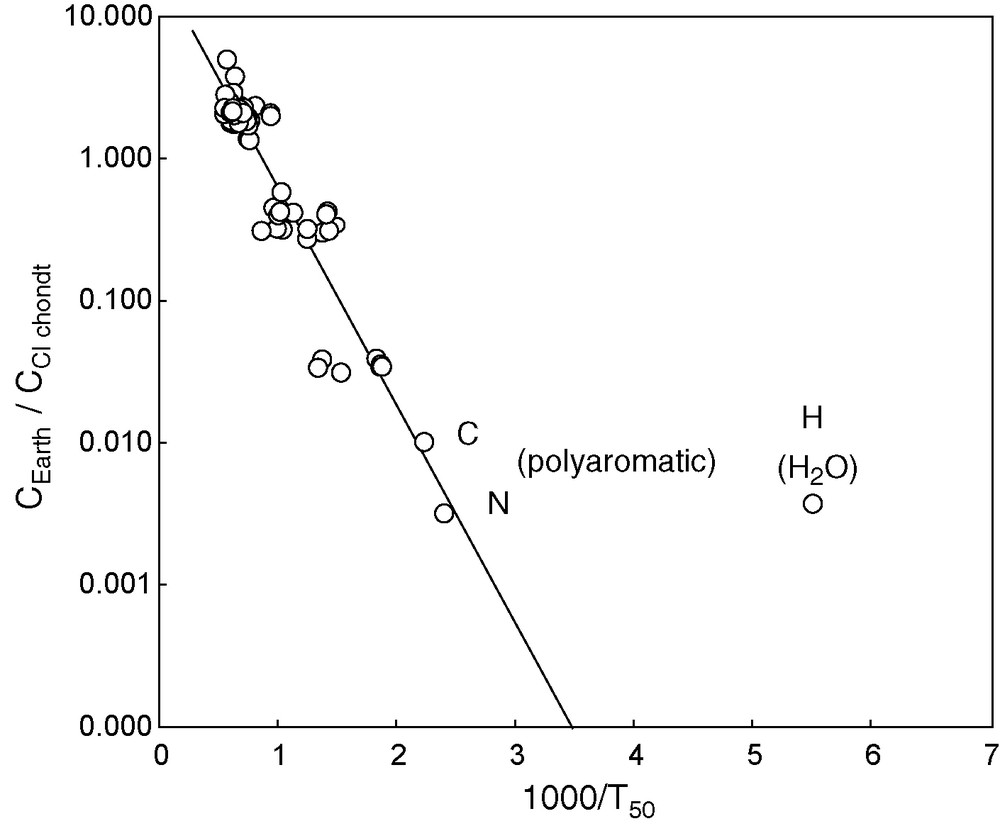
Depletion factor of the Earth's composition [23] with respect to CI carbonaceous chondrites [3]. The values of T50, the 50 percent condensation temperatures, were taken from Lodders [34] except for those of C and N (∼200 °C), which correspond to the condensation of polyaromatic compounds, the most common form of these elements in carbonaceous chondrites [19,49].
Fig. 3. Facteurs d’appauvrissement de la composition terrestre [23] par rapport aux chondrites carbonées CI [3] Les valeurs de T50, la température à laquelle 50 % de l’élément considéré est condensé, proviennent de Lodders [34] à l’exception de celles pour C et N (≈200 °C) qui correspondent aux températures de condensation des composés aromatiques polycycliques, la forme la plus commune sous laquelle sont présents ces deux éléments dans les chondrites carbonées [19,49].
The elements plotted in Fig. 3 form a reasonably linear alignment leaving only H off the trend and by several orders of magnitude. This is a strong indication that the bulk of H2O was added to the Earth by a mechanism different from the normal accretion sequence. It is now commonly admitted [2,35,38,44] that a substantial part of the water present in the inner Solar System may have been imported from the outer Solar System when icy asteroids from behind the snow line were perturbed by the gravitational effect of Jupiter. As argued by Robert [44] for D/H, and by Drake and Righter [18] for Ar/H2O, the terrestrial ratios are inconsistent with a cometary origin. The D/H ratios are, however, consistent with those measured for the hydrous minerals of carbonaceous chondrites. Drake and Righter [18] correctly pointed out that the Earth and carbonaceous chondrites have different Kr/Xe ratios, but the modern perception of material fluxes [15,55] indicates that more than one class of meteorites, whether carbonaceous or enstatite chondrites [32], must have contributed to the late veneer. This leaves copious room to accommodate the constraints of terrestrial rare gas abundances and distributions, especially if proper consideration is given to the aqueous alteration and metamorphism on small planetary objects. It is unfortunate that the same argument cannot be made for other planets: a few tens of plutonic rocks, heavily shocked, represent the meagre and non-compelling sampling of Mars’ interior, while Venus is still beyond reach.
5 The magma ocean and its fate
Whether or not a planet started out with a magma ocean depends on a number of parameters: the temperature of the accreting material, the size of the planet, the existence of an atmosphere, and the existence of a conductive boundary layer.
It is now well understood [15,55] that large planets form essentially by coalescence of planetary bodies with radii of hundreds to thousands of kilometres (runaway growth). The temperature of the accreting material is related to the rate of accretion of the small planetary bodies: slowly accreting bodies, which within a few million years did not reach the size of a thermal boundary layer (∼10 km) lost the radioactive heat from 26Al (T1/2 = 0.75 Ma) and 60Fe (T1/2 = 1.5 Ma) decay and ended up as relatively cold objects. Bodies that accreted faster must have been largely molten and contributed their internal heat to the target planet.
The effect of the size of a planet is visible in Fig. 2. Planets the size of asteroids barely heat up upon accretion, while bodies the size of the Moon or Mercury heat up moderately. In contrast, gravity releases enough energy at the surface of planets the size of the Earth, Venus, and Mars to melt and even vaporize most of their outermost layers. Heating is limited by radiation into space. Equating radiation rate with the rate of release of gravitational energy gives:
When the gravity of a planet is weak, the depth interval of plagioclase saturation is important: large amounts of this mineral collect and float to the surface. This is the case of the Moon and probably also of the parent body of eucrites. Plagioclase crystallization from anhydrous basaltic melts produces ‘anorthositic’ foam (the lunar highlands) down to depths of about 0.5 GPa, which corresponds to ∼ 30 km on Earth but to nearly 200 km on the Moon. On Earth, plagioclase is only a significant magmatic mineral for magmas erupted at mid-ocean ridges or in large igneous provinces, whereas it controls important characteristics of the Moon's internal structure. Fig. 4 compares rare-earth element concentrations in lunar basalts from Apollo 15 [39], 3.4 Ga old komatiites from Barberton, South Africa [33], and 2.7 Ga old basalts from Belingwe, Zimbabwe [51]: the strongly negative Eu anomaly (×0.5) of mare basalts indicates that the source of these liquids had at one point during its evolution been depleted in a plagioclase component by plagioclase flotation in the lunar magma ocean [57]. On a large planet with no hydrosphere and no atmosphere, the magma ocean crystallizes from the bottom up. Since it is not protected by a buoyant plagioclase lithosphere, it quickly looses all its heat by radiation into space. This explains why, until the demonstration of the presence of 142Nd anomalies in terrestrial rocks [12–14], the concept of a lasting (30 Myr or more) terrestrial magma ocean, with few noticeable exceptions [1], had been essentially abandoned.
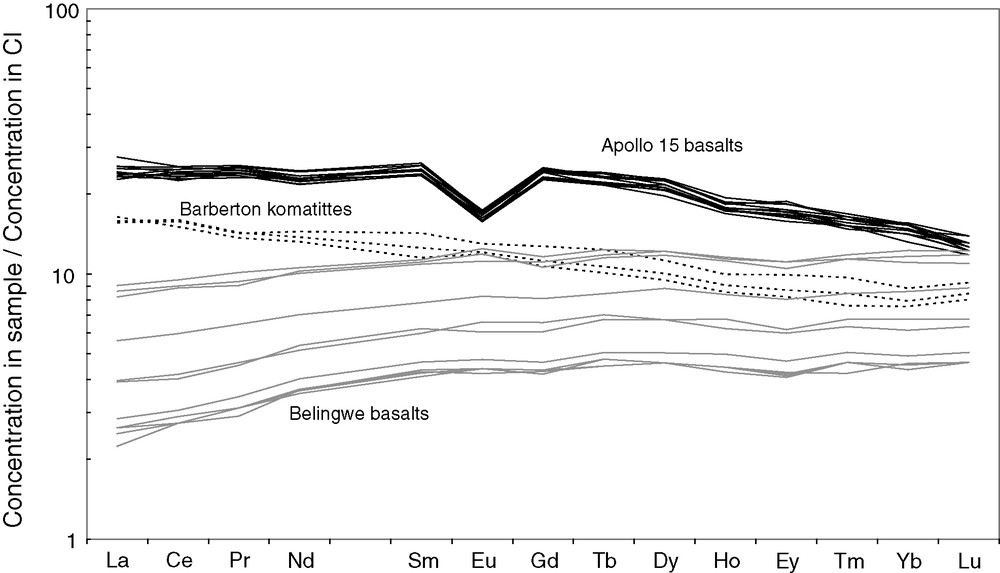
Comparison of rare-earth element concentrations normalized to CI chondrites in lunar basalts from Apollo 15 [39], 3.4 Ga old komatiites from Barberton [33], and 2.7 Ga old basalts from Belingwe [51]. A Eu anomaly is indicative of plagioclase/basaltic liquid fractionation. The deep anomaly visible in lunar basalts (×0.5) cannot result from their fractional crystallization and indicates that the mantle source itself was depleted in Eu by plagioclase flotation in the magma ocean. This feature is missing in terrestrial mafic and ultramafic magmas.
Fig. 4. Comparaison des concentrations des terres rares normalisées aux chondrites CI des basaltes lunaires d’Apollo 15 [39], de komatiites de Barberton [33] et de basaltes de Belingwe [51]. L’anomalie d’europium est indicative d’un fractionnement plagioclase/liquide basaltique. La profondeur de l’anomalie d’europium dans les basaltes lunaires ne peut être produite par cristallisation fractionnée des liquides magmatiques. Elle indique que la source mantellique elle-même a été appauvrie en europium par flottaison de plagioclase dans l’océan magmatique lunaire.
As suggested by Boyet et al. [13], the presence of surface water creates an entirely different situation. The gravity field of the Moon and probably that of Mercury as well is too small to retain water, but not so at the surface of the Earth, Mars, and Venus. At the surface of a magma ocean radiating into space, a solid crust will form, dominated by olivine- and pyroxene-rich rocks. When this crust, which resembles the ‘skin’ observed at the surface of modern lava lakes, thickens under vacuum, its negative buoyancy makes it founder into the magma, which thereby anew becomes exposed to radiation into space. In contrast, if the crust is in contact with water rich in silica (highly soluble at high temperatures), olivine and pyroxene react to form a number of hydrous minerals, notably serpentine, talc, and anthophyllite (Fig. 5). The most relevant of these reactions to the present scenario are those describing the formation of magnetite and quartz from fayalite with the release of hydrogen and the combination of forsterite with the newly produced quartz and water to produce serpentine [5]:
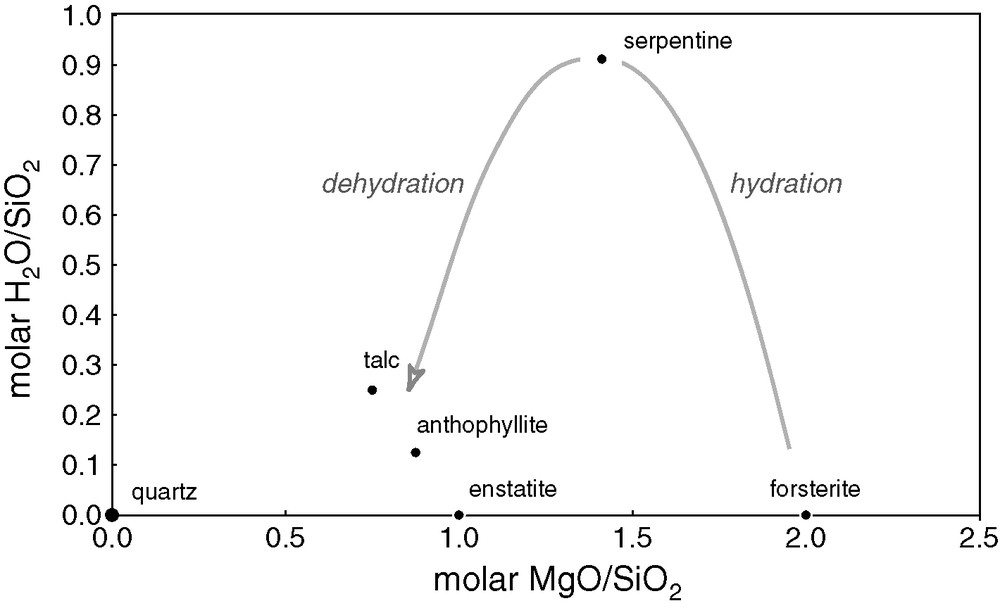
Hydration reactions between Mg-rich minerals. At increasing temperatures, silica-rich solutions react with forsterite (olivine) to produce low-density hydrous minerals, serpentine, talc, and finally anthophyllite (amphibole).
Fig. 5. Réactions d’hydratation des minéraux riches en magnésium. Lorsque la température augmente, les solutions riches en silicium réagissent avec la forstérite (olivine) pour former des minéraux hydratés de faible densité, tels que la serpentine, le talc et l’anthophyllite (amphibole).
The temperature-pressure stability fields of the essential minerals of the MgO–SiO2 system were calculated using standard thermodynamic tables [45] and are represented in Fig. 6. The density of these hydrous minerals, which are stable between 400 and 700 °C (Table 1 and [6]) is particularly low (2.6–2.8 g cm−3). Consequently, such a near-surface hydrous layer may provide buoyancy to a thick pile of underlying anhydrous rocks (notably dunite). For a temperature gradient of 200 K km−1 (about 6 km to the magma temperature of about 1200 °C), the thickness of the hydrous layer is of about 2 km, which is enough to ensure buoyancy of the entire crust. Such an early hydrous crust should have formed at the surface of all planets and be large enough to have been affected by a substantial release of gravitational energy (i.e., Earth, Mars, and Venus) under a strong bombardment of icy asteroids. Thermal absorption by atmospheric CO2 and H2O of the heat radiating from the ground, whether the atmosphere was outgassed by impact or contributed by the outer Solar System, substantially reduced the loss of internal heat [1,36,58]. Together with the creation of a boundary layer of hydrous rocks, the presence of an atmosphere therefore helped stabilize the magma ocean in large rocky planets. The existence of a high gas pressure is, however, questionable: Jeans escape of the huge quantities of hydrogen evolving from serpentinization was certainly instrumental in ‘lifting’ a substantial fraction of all the atmospheric gases outside of the terrestrial gravity field, a process known as dynamic loss [29]. The importance of these processes for the origin of life is nevertheless extreme because they create precisely the reducing environments required for the Miller–Urey experiments [52].
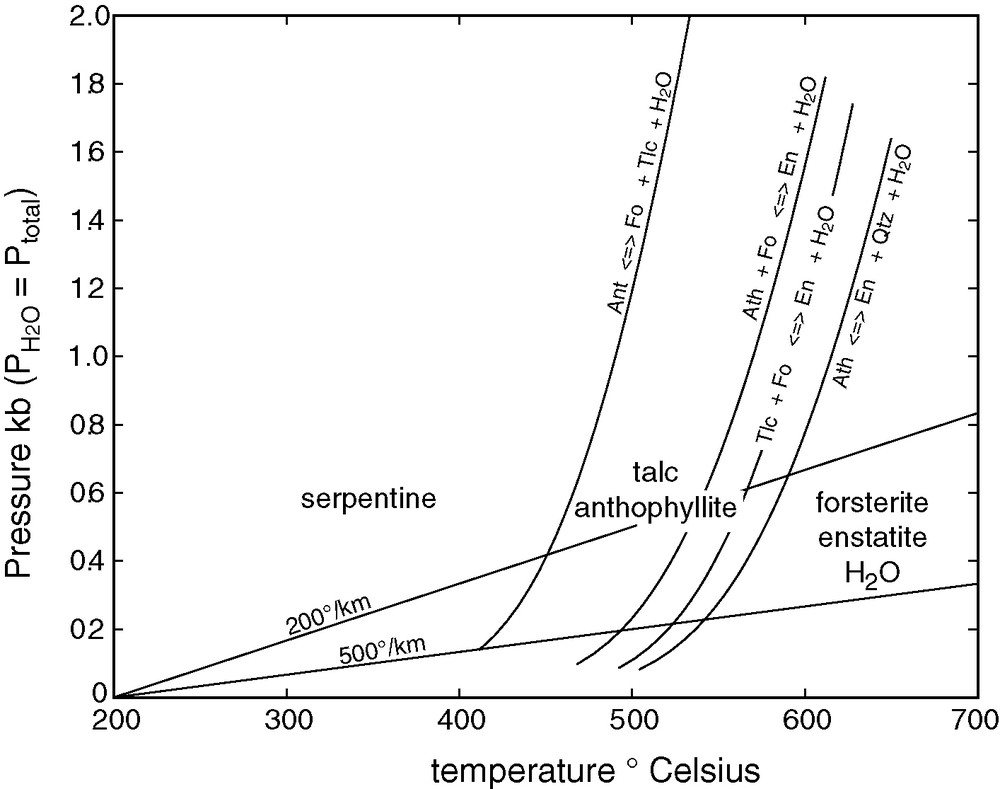
Stability fields of hydrous phases in mafic/ultramafic rocks overlying a magma ocean. Thermodynamic data from ref [45]. Ant = antigorite, Ath = anthophyllite, En = enstatite, Fo = forsterite, Qtz = quartz, Tlc = talc. Two extreme geotherms are shown to illustrate the thickness of the hydrous layer.
Fig. 6. Champs de stabilité des phases hydratées dans des roches mafiques/ultramafiques à la surface d’un océan magmatique. Les données thermodynamiques proviennent de la référence [45]. Ant = antigorite, Ath = anthophyllite, En = enstatite, Fo = forsterite, Qtz = quartz, Tlc = talc. Deux géothermes extrêmes sont représentés pour figurer l’épaisseur de la couche hydratée.
6 Planetary size and chemical evolution
Let us now draw the consequences of the introductory discussion which showed that a large radius grants a planet a strong pressure gradient and a large volatile content and compare some critical geochemical and mineralogical features of the various planetary bodies for which we have geological samples.
6.1 The Earth
This is the largest of the rocky planets and its strong gravity field has endowed it with a fully developed atmosphere and a liquid ocean. High δ18O in ∼4.4-Ga-old zircons from Jack Hills (western Australia) strongly indicates the presence of material altered under low-or medium-temperature hydrous conditions in the source of their parent granites and is considered as strong evidence for the early presence of a hydrosphere [37,56]. The mantle currently is wet with ∼100–300 ppm H2O if we consider the normal water content of a mid-ocean-ridge basalt and assume that it formed by 10% partial melting of its mantle source. If the water content of the mantle is homogeneous, approximately the equivalent of the mass of the modern oceans is buried in the mantle. Because of the higher affinity of wadsleyite for water, it has been suggested that the transition zone is particularly hydrous [7,10], but such a deep hydrous layer requires the undocumented existence of melting with melt segregation. For reference, and from the discussion above, this may be accounted for by the addition of 2–3‰ of CI chondrites to a dry proto-Earth, a figure consistent with those derived from the terrestrial concentration of highly siderophile elements and the ‘late-veneer’ assumption [16]. Under a lid of a few kilometres of a primordial crust made of hydrous minerals at the top and of dunite ± harzburgite at the bottom, very little plagioclase can crystallize. By the end of the crystallization of the magma ocean (>30 Myr after terrestrial accretion; see Bourdon et al, this volume), its last liquids together with melts from the uppermost mantle form a thin layer of differentiated rocks with very low Sm/Nd and Lu/Hf ratios due to garnet having crystallized as a minor phase at depth [12,22] (see also [9]).
Cold crust eventually sinks and rapidly carries water to depths [30]: upon hydration, the viscosity of the mantle drops by several orders of magnitude [27], the lithosphere can bend, and the mantle can yield. Mantle hydration thus marks the onset of plate tectonics and the hallmark of this dynamic regime so characteristic to the Earth is granite: such rocks, which are essentially absent from terrestrial oceanic domains, the Moon, and the entire meteorite collection, can only form by wet melting at subduction zones. Taking the age of the granite that hosted the Jack Hills zircons [26] as starting point, we suggest that this transition happened at about 4.35 Ga [9].
6.2 The Moon
Our satellite was shaped into a dry and inactive planetary body by conditions that were very different from those that prevailed on Earth, but which boil down to a single parameter, gravity. The ‘giant impact’ is the broadly accepted theory accounting for the origin of the Moon. This event interfered importantly with the Moon's early thermal evolution. Because gravity was very weak and the protective effect of a magnetic field was absent, the common gases of the terrestrial atmosphere (N2, CO2) quickly escaped the planet. The amount of water in the Moon today is very small, probably of the order of a few ppm [23].
The existence of a lunar magma ocean was documented by the very first results of the Apollo missions [57]. The most remarkable feature is that the low gravity field of the planet and the absence of water stabilized plagioclase over ∼200 km: buoyant anorthosite and plagioclase-rich rocks ensured the insulation of a thick layer of melt (conductive boundary layer). The complementary Eu anomalies in (ferroan) anorthosites and mare basalts (melts from the cumulative interior), the ubiquity of ilmenite, which appears only after ∼90% crystallization of the melt and the presence right beneath the lithosphere of a KREEP component representing the ultimate last drops of liquid before complete magma ocean solidification, leave little doubt that a magma ocean was present on the Moon for tens and probably hundreds of millions of years underneath the anorthositic highlands.
The lunar magma ocean stage was followed by sparse magmatic activity involving the high-Mg suite and KREEP basalts until 3.9 Ga after which the mare basalts were emplaced, lasting from ∼3.8 to ∼3.4 Ga [50]. The persistence of strong reliefs in the highlands suggests that, by the time of the late heavy bombardment (3.8–3.9 Ga), the mantle had largely consolidated.
6.3 Mars
The situation on Mars is somewhat intermediate to the terrestrial and lunar scenarios. It is accepted that Mars initially had an atmosphere and probably also a hydrosphere and the uncertainties are concerned more with how long they each lasted [31]. The case for a magma ocean is weaker, mostly because sampling is limited to the few tens of the so-called SNC meteorites (shergottites, nakhlites, and chassignites) believed to originate from Mars. The notion of a Martian magma ocean has nevertheless recently been reinvigorated by the discovery of 142Nd anomalies in SNCs [21,25], which suggest that there was a magma ocean but that it lasted for only a few tens of million years. Among the SNC meteorites, those with a composition closest to basaltic have been deemed young (<200 Ma) for decades [41], making them essentially useless to discuss the early times of the planet. Bouvier et al. [11], however, recently reassessed the age of these rocks and suggested a magmatic emplacement of 4.1 Ga, thus reintroducing these samples as potential witnesses to the early history of Mars. If this estimate is correct, a strong argument can be derived from the Hf/Sm ratios of the different groups of Martian meteorites. In terrestrial basalts, this apparently exotic ratio does not vary by more than 15% (2 sigma) about the chondritic value of 0.75, which reflects that these two elements are incompatible and that their solid/liquid partition coefficients are not very different from each other. In contrast, the Hf/Sm ratio is 2 × chondritic in shergottites and 0.3 × chondritic in nakhlites and chassignites [8]: such an extreme and ubiquitous fractionation requires a particular mineralogy and the only acceptable mineral is ilmenite, which was already found earlier to fingerprint extreme crystallization in the lunar magma ocean. The Hf/Sm ratios of shergottites thus support the existence of a magma ocean on Mars as indicated by the 142Nd anomalies.
Mars has no highlands, no visible maria, no continents, and no plate tectonics, but it has a few tall and probably rather young volcanoes (Olympus Mons, Tharsis) standing well above the base level of the northern hemisphere. The southern hemisphere was too heavily cratered during the late heavy bombardment [4] for the subsurface lithologies to be identifiable. From the dominant mineralogy of SNC meteorites, it can be speculated that igneous rocks of mafic compositions must be abundant. The reason why Mars did not develop a plate tectonic regime is not clear but may be related to rapid removal of water by hydrodynamic escape, which simply did not leave enough hydrous rocks to founder into the mantle: the resulting low abundance of water combined with weaker gravity than on Earth worked against the bending and foundering of lithospheric plates and the planet instead took the dynamic route of developing a thick stagnant lid.
6.4 Venus
The fact that today our sister planet Venus has no liquid ocean – nor the atmospheric water vapour to form one – and, in spite of many features reminiscent of the Earth's relief, no active plate tectonics, suggests a dynamic evolution different from that on Earth. The hypsometric curves of the two planets clearly show that Venus does not have the terrestrial bimodality of elevations of oceanic and continental plates [46]. Impact cratering chronology further suggests that the planet was resurfaced some 500 Myr ago [42,47]. Both the apparently low viscosity of the Venus mantle and the lack of water in the atmosphere may indicate that the missing water was dragged down into the mantle during an earlier regime of plate tectonics, thereby decreasing mantle viscosity, while mantle CO2 was largely outgassed.
7 Conclusions
This short review shows that the most crucial dynamic features of a rocky planet on this side of the snow line and its ability to sustain plate tectonics and perhaps life boil down to a single parameter, its radius at the end of accretion.
Acknowledgements
Insightful reviews by Pierre Cartigny, Marc Chaussidon, and François Robert are gratefully acknowledged.