1 Introduction
Chromium (Cr) and nickel (Ni) are present in high concentrations in soils developed on ultramafic rocks; up to 125,000 mg/kg of Cr [1] and more than 10,000 mg/kg of Ni [10,23] were observed, while the concentrations of these metals in other soils commonly range from 0 to 100 mg/kg [12,19]. The presence of Cr and Ni and low concentrations of nutrients like N, P, K and Ca are generally thought to be responsible for the low fertility of these soils. Moreover, Cr has different degrees of toxicity depending on its oxidation state: Cr (VI) is a strong oxidising agent, carcinogenic and thus highly toxic, while Cr (III) is a micronutrient and a non-hazardous species [19]. Generally, the highest geogenic Ni and Cr contents are observed in ultramafic parent rocks, such as peridotite, dunite and pyroxenite, where these elements are present in various Mg- and Fe-rich mafic minerals (spinels, pyroxenes, olivines…). In addition, inclusions of Ni-bearing sulphides can also be observed in these rocks [7]. These minerals are susceptible to weathering in cold continental and temperate climates, and the soils formed on this type of mafic bedrock are subsequently composed of inherited serpentinitic minerals (e.g., serpentine), and more generally clay minerals [4,14]. Under more intensive weathering conditions like those in the humid tropics, Fe- and Mn-oxides are the main scavengers of Ni and Cr [2,27,28,30] and garnieritic Ni-containing phases may also be formed [8]. The toxicity of these metals has been widely studied, but it is not clear if they have a toxic effect in these naturally enriched environments, as they are generally bound in resistant minerals like silicates, oxides or spinel [2,23,24], and are not very soluble under common Eh-pH conditions in soils. Nevertheless, recent studies showed a high variability in Ni availability, depending on the physicochemical, mineralogical and edaphic properties of these ultramafic soils [2,6,17,27]. Thus, the forms in which metals exist in soils are fundamental for predicting their availability.
More than the total concentration of metals, which is commonly used as a criterion of soil pollution, it is essential to determine their speciation and availability, which are related to the ecotoxicological risk. Metal availability depends on their origin [12], their partitioning, i.e. their distribution among the soil constituents, which control their mobility and availability for biota [6] and edaphic properties like the pH, Eh, and clay and organic matter contents [18]. In fact, only a fraction of the metals is generally available for organisms and is thus potentially toxic [25]. To the present time, no unique method exists to assess metal availability in soils and a battery of plant and ecotoxicological tests, chemical extraction protocols and also, more recently, isotopic exchange methods have been used for this purpose [6,21]. In the last tests, it has been shown that the DTPA extractable Ni was significantly correlated to isotopically exchangeable Ni [4,6]. Thus, DTPA can be considered to be suitable for assessment of the Ni availability in soils.
A large number of mantle-derived peridotites have been studied from the geochemical point of view in the Bohemian Massif (Czech Republic) [20]. However, the soil profiles developed on these ultramafic rocks have not been studied yet, with the exception of several samples used for the Ni-availability study in the work of Massoura et al. [17]. The aim of this paper is to describe the location of Cr and Ni in the bedrock/soil matrix, i.e. Ni- and Cr-bearing minerals, and their availability using a combination of mineralogical and geochemical methods in soil profiles derived from serpentinized peridotites from several Czech localities. This type of approach is essential to determine the binding mechanisms of these elements to the soil constituents and thus the environmental risk associated with their presence in the soils.
2 Materials and methods
2.1 Soil sampling
The soil profiles were sampled at three sites with ultramafic bedrock that is representative for the Czech Republic (Fig. 1). The soil was covered by a meadow at Nové Dvory (ND), a steppe-like grassland at Mohelno 1 (M1), where metal-accumulating plants also occurred (Alyssum sp.), and forest dominated by pine trees in all the other cases (Mohelno 2, M2; Holubov, H1, H2). The climate is cold semi-continental. Pits were opened at each selected site until stones predominated and the soils were described and sampled according to the natural development of soil horizons. The soil samples were air-dried and sieved to less than 2 mm prior to analysis. Samples of the underlying bedrocks were also collected from the base of each pedon.

Location of the sampling sites.
Localisation des sites d’échantillonnage.
2.2 Mineralogical analyses
X-ray diffraction analysis (XRD) of pulverized bedrocks and soils was performed on a PANalytical X’Pert Pro diffractometer (CuKα radiation, 40 kV, 45 mA, 2theta range 5–80°, step 0.02, counting time 150 s per step, X’Celerator multichannel detector). Qualitative analysis of the obtained diffractograms was carried out with the X’Pert HighScore software, version 1.0d (PANalytical, Netherlands) equipped with a PDF-2 database [11].
Samples of bedrock were embedded in the resin and prepared as polished thin sections for the optical microscopy study (Leica MPS 60 polarizing microscope). A CamScan S4 scanning electron microscope (SEM) equipped with an Oxford Link ISIS energy dispersion spectrometer (EDS) was used for electron microscopy observation and analyses (the accelerating voltage was 20 kV). For the analyses, the EDS system was calibrated against the following set of standards: albite (Si, Al, Na), marcasite (Fe, S), pentlandite (Ni), rhodonite (Mn), cuprite (Cu), crocoite (Cr), diopside (Ca, Mg), rutile (Ti), sanidine (K), willemite (Zn), vanadinite (V), pure cobalt (Co).
Soil samples (0–2 mm) were examined by scanning electron microscopy (Philips XL30) equipped with an energy dispersive spectrometer (EDX-PGT Ge-detector) at the University Paris-Sud-XI.
2.3 Chemical analyses and extractions
The bulk analyses of bedrocks were performed according to standard methods [32]. The total element concentrations in the soil samples were determined after acid digestion (hot, concentrated HF, HClO4, HNO3 and HCl in Savillex® teflon vessels) of finely ground ash samples, to ensure complete dissolution of the soil samples.
The pH was determined in a 1/2.5 soil/water or soil/1 M KCl mixture (w/v). Organic carbon (OC) was quantified by dry combustion using an ELTRA CS 530 analyzer. The accuracy of the OC measurement was controlled using NIST 2710 standard reference material (Montana I soil) and was always better than 10%.
The phytoavailability of the metals was determined by extraction with 0.005 M DTPA + 0.01 M CaCl2 buffered at pH 5.3 (modified from [15]). The 1/5 soil/solution ratio (w/v) suspensions were mixed end-over-end for 2 h, centrifuged and filtered to 0.45 μm (Millipore®). Element partitioning within the soil matrix was investigated by selective sequential extractions. Even if no satisfactory physicochemical or chemical method exists to unambiguously determine the element partitioning amongst the solid phases, this procedure provides an operationally defined solid-phase fractionation, convenient for soil comparisons. Soil samples (1 g) were first extracted with 20 ml of 0.5 M NH4Cl to obtain exchangeable cations. Then samples were sequentially extracted with 20 ml of 0.25 M NH2OH·HCl in 0.25 M HCl at 60 °C, in order to dissolve Mn oxides and amorphous or poorly crystallised Fe-(hydr)oxides (labelled AMF), and with 1.0 M NH2OH·HCl in 25% CH3COOH at 90 °C, in order to dissolve crystalline Fe-oxides (labelled CRF) [5,9]. The elements in the extracts were determined by ICP-AES (Varian Liberty 200, Australia). Calibration was performed with standard solutions in the appropriate matrix and analysed at the beginning of the series and after each series of 15 samples.
3 Results and discussion
3.1 Bedrock composition
The bulk chemical analyses showed that the bedrocks are enriched in Cr and Ni, with contents exceeding 3000 mg/kg (Table 1). The XRD revealed that the bedrocks of all three localities were predominantly composed of serpentine (lizardite, [Mg, Al]3[Si, Fe]2O5[OH]4). Magnesium-bearing olivine (forsterite, MgSiO4) was also very commonly observed, representing a significant source of Ni (generally ∼ 0.4 weight percentage NiO). Both orthopyroxenes (enstatite, [Mg, Fe]SiO3) and clinopyroxenes (diopside, [Ca][Mg, Fe]Si2O6) were also detected as minor phases, the latter being absent in the bedrocks from Holubov. They are important sources of Cr (∼ 0.4–1.1 weight percentage Cr2O3) (Fig. 2a). This observation is in agreement with the geochemical data on peridotite minerals from Nové Dvory and Mohelno published by Medaris et al. [20] and the Cr concentration ranges reported for pyroxenes from other sites [23]. Trace amounts of amphiboles (glaucophane, Na2[Mg, Fe]3Al2Si8O22[OH]2, at Nové Dvory and Mohelno and antophyllite, [Mg, Fe]7Si8O22[OH]2, at Holubov) were also detected by XRD. Interestingly, spinels were not detected by XRD, but were, however, confirmed by SEM/EDS as significant hosts of Cr (up to 54 weight percentage Cr2O3) (Fig. 2a,c,f). Only low concentrations of Ni (< 0.5 weight percentage NiO) were detected in spinels by EDS (Fig. 2). Sulphides (mainly pentlandite, [Fe, Ni]9S8) are trace, but important primary Ni-bearing phases; they are generally observed as small inclusions with alteration rims (Fig. 2b). Serpentine generally fills the spaces between the residual grains of primary minerals or directly forms the alteration rims (Fig. 2). The serpentine formed by alteration of Cr- or Ni-bearing primary phases is commonly enriched in these two elements. Serpentine associated with the weathered olivine may contain up to 2 weight percentage NiO (Fig. 2d). Similarly, serpentine filling the fractures in Cr-bearing spinel may contain up to 3.4 weight percentage Cr2O3 (Fig. 2f). Fe-oxides are relatively rare in bedrocks, generally forming the alteration rims of (Fe, Ni)-sulphides and being slightly enriched in Ni (Fig. 2b).
Bulk analyses of serpentinitic bedrocks of individual soil profiles
Analyses globales des roches-mères serpentiniques des profils étudiés
Location | Nové Dvory | Mohelno | Holubov | ||
Sample | ND1a | M1 | M2 | H1 | H2 |
Weight percentage | |||||
SiO2 | 41.38 | 43.32 | 41.68 | 39.54 | 41.54 |
TiO2 | 0.07 | 0.16 | 0.15 | 0.03 | 0.02 |
Al2O3 | 2.30 | 3.49 | 3.20 | 0.80 | 0.71 |
Fe2O3 | 7.86 | 8.02 | 7.31 | 7.31 | 5.81 |
FeO | 2.05 | 2.80 | 2.46 | 1.32 | 2.91 |
MnO | 0.11 | 0.13 | 0.12 | 0.10 | 0.10 |
MgO | 31.95 | 29.07 | 31.55 | 36.59 | 39.82 |
CaO | 1.88 | 3.03 | 2.43 | 0.24 | 0.53 |
Na2O | 0.16 | 0.19 | 0.17 | 0.02 | 0.02 |
K2O | 0.02 | 0.03 | 0.01 | 0.01 | 0.01 |
P2O5 | 0.02 | 0.03 | 0.02 | 0.03 | 0.01 |
LOIb | 11.31 | 8.99 | 10.36 | 13.50 | 7.95 |
Sum | 99.11 | 99.26 | 99.46 | 99.49 | 99.43 |
mg/kg | |||||
Cr | 2682 | 3206 | 2753 | 1802 | 2190 |
Co | 103.2 | 96.9 | 92.2 | 111.8 | 108.4 |
Ni | 3054 | 2198 | 2453 | 2824 | 2809 |
a The bedrock at Nové Dvory was identical for both profiles, being sampled several meters apart/roche-mère identique pour les deux profils échantillonnés à Nové Dvory.
b LOI – loss on ignition/perte au feu.

Scanning electron micrographs showing the principal phases and alteration features in bedrocks. a: amphibole (glaucophane) kelyphite and spinel inclusions within the matrix composed of individual altered orthopyroxene and clinopyroxene grains (Nové Dvory); b: altered pentlandite inclusion transformed to a complex Fe-Si mixture and Fe-oxide within the serpentine matrix (Nové Dvory); c: zoned Cr-bearing spinel grains associated with amphibole (antophyllite)-spinel kelyphite within the serpentinized matrix including individual altered olivine crystals (Holubov, profile 1); d: olivine boundary altered to serpentine (Holubov, profile 2); e: olivine crystal transformed to Fe- and Mg-serpentine (Holubov, profile 2); f: altered spinel with cracks filled with a zoned mixture of Fe- and Mg-serpentine (Mohelno, profile 2). Abbreviations: amph – amphibole, opx – orthopyroxene, cpx – clinopyroxene, spl – spinel, pn – pentlandite, serp – serpentine, ol - olivine; the indicative Cr and Ni contents obtained by EDS analytical system are given in weight percentage of Cr2O3 and NiO, respectively.
Microphotographies des phases principales et produits d’altération des roches-mères, obtenues par microscopie électronique à balayage. a : amphibole (glaucophane) avec inclusions de spinelle dans une matrice composée des grains altérés de clinopyroxène et orthopyroxène (Nové Dvory) ; b : pentlandite altérée dans un mélange complexe de Fe-Si et d’oxyde de Fe dans une matrice de serpentine (Nové Dvory) ; c : spinelles chromifères zonés, associés à une myrmékite d’amphibole (antophyllite) et spinelle dans une matrice serpentinisée avec de rares cristaux d’olivine (Holubov, profil 2) ; d : cristal d’olivine altéré en serpentine (Holubov, profil 2 ); e : cristal d’olivine transformé en serpentine et serpentine riche en Fe (Holubov, profile 2) ; f : spinelle altérée et fissurée, avec remplissages de serpentine. Abréviations : amph – amphibole, opx – orthopyroxène, cpx – clinopyroxène, spl – spinelle, pn – pentlandite, serp – serpentine, ol – olivine; les teneurs indicatives en Cr et Ni obtenues par les analyses EDS sont données en pourcentage de poids de Cr 2 O 3 et NiO.
3.2 Soil properties and bulk concentrations
The main morphological features of the studied profiles are summarized in Fig. 3. The soils have the following common characteristics: dark colour in the topsoil, high root density in the A horizons, loamy to sandy loam texture and serpentine stones in the C horizons. Some of the latter have a light olive-brown colour. Finally, soils are not deep and do not exceed a depth of 80 cm. Selected chemical and physicochemical characteristics of soils are presented in Tables 2 and 3. According to WRB-FAO, soils can be roughly classified as Regosol and Magnesic (or Hypermagnesic, see [4]) Cambisol with no evidence of clay illuviation or Fe segregation.

Macromorphological description of the soil profiles studied, with the main pedological features.
Description morphologique des profils de sols étudiés avec les principales caractéristiques pédologiques.
Main physicochemical parameters of soil samples
Principaux paramètres physicochimiques des sols étudiés
pH | OC | Sum of exchangeable cations | Exchangeable cations (cmolc/kg) | Ca/Mg | |||||
Water | KCl | g/kg | cmolc/kg | Ca | Mg | Na | K | Mol ratio | |
Nové Dvory | |||||||||
ND1 A1 | 6.8 | 6.0 | 153 | 15.3 | 5.1 | 8.2 | 0.8 | 1.2 | 0.620 |
ND1 A2 | 7.1 | 6.1 | 162 | 23.9 | 5.5 | 16.9 | 0.9 | 0.7 | 0.323 |
ND1 C1 | 7.4 | 6.3 | 192 | 26.4 | 2.3 | 16.8 | 7.2 | 0.1 | 0.138 |
ND1 C2 | 7.6 | 6.4 | 140 | 20.0 | 1.7 | 17.3 | 0.9 | 0.1 | 0.095 |
ND2 A | 6.4 | 5.8 | 133 | 15 | 4.9 | 8.0 | 0.8 | 1.4 | 0.605 |
ND2 C | 7.0 | 6.1 | 122 | 17.8 | 4.1 | 11.3 | 1.5 | 0.9 | 0.366 |
Mohelno | |||||||||
M1 A | 5.8 | 5.2 | 349 | 18.4 | 5.5 | 10.7 | 0.8 | 1.4 | 0.519 |
M1 C | 6.5 | 5.8 | 140 | 18.2 | 4.3 | 12.5 | 0.5 | 0.9 | 0.339 |
M2 A | 5.9 | 5.2 | 249 | 22.2 | 4.2 | 17.1 | 0.6 | 0.3 | 0.245 |
M2 B | 6.8 | 5.6 | 145 | 24.1 | 2.5 | 20.7 | 0.6 | 0.4 | 0.122 |
M2 C | 7.0 | 5.5 | 136 | 25.7 | 2.2 | 22.4 | 0.7 | 0.4 | 0.100 |
Holubov | |||||||||
H1 O | 4.7 | 4.2 | 398 | nda | nd | nd | nd | nd | nd |
H1 A | 6.2 | 5.5 | 155 | 11.7 | 0.6 | 10.5 | 0.5 | 0.1 | 0.058 |
H1 B | 7.2 | 6.0 | 125 | 34.2 | 0.1 | 12.7 | 21.2 | 0.2 | 0.009 |
H1 C | 7.4 | 6.0 | 150 | 21.5 | 0.7 | 16.5 | 4.3 | 0.1 | 0.042 |
H2 A | 5.8 | 5.2 | 219 | 13.1 | 1.1 | 11.2 | 0.5 | 0.3 | 0.099 |
H2 C | 7.1 | 6.2 | 153 | 16.5 | 0.6 | 15.2 | 0.6 | 0.1 | 0.038 |
a nd – not determined/non déterminé.
Bulk analyses and selected extraction results for soil samples
Analyses globales et résultats d’extraction
Total | AMFa | CRFb | |||||||||||||||
Al | Mg | Fe | Si | Mn | Cr | Ni | Fe | Si | Mn | Cr | Ni | Fe | Si | Mn | Cr | Ni | |
g/kg | mg/kg | mg/kg | mg/kg | ||||||||||||||
Nove Dvory | |||||||||||||||||
ND1 A1 | 29.3 | 66.5 | 46.9 | 262 | 1021 | 1380 | 885 | 9085 | 3249 | 848 | 68 | 491 | 11300 | 2768 | 132 | 120 | 378 |
ND1 A2 | 25.5 | 84.0 | 75.5 | 231 | 1387 | 1870 | 1421 | 14420 | 4045 | 1098 | 112 | 709 | 14975 | 2620 | 183 | 191 | 448 |
ND1 C1 | 13.0 | 132.1 | 79.4 | 189 | 792 | 1587 | 2114 | 14040 | 3438 | 530 | 85 | 1053 | 11925 | 2653 | 135 | 172 | 790 |
ND1 C2 | 14.4 | 144.2 | 79.5 | 203 | 843 | 1737 | 2037 | 15140 | 3426 | 650 | 70 | 1092 | 11825 | 2225 | 156 | 148 | 548 |
ND2 A | 39.0 | 79.5 | 54.3 | 245 | 1090 | 1409 | 859 | 10670 | 3195 | 439 | 63 | 439 | 10175 | 2335 | 142 | 133 | 361 |
ND2 C | 24.2 | 126.5 | 76.6 | 218 | 1094 | 1476 | 1693 | 13055 | 2843 | 756 | 61 | 701 | 12200 | 2138 | 165 | 201 | 710 |
Mohelno | |||||||||||||||||
M1 A | 25.4 | 53.2 | 49.5 | 191 | 938 | 705 | 515 | 10830 | 2713 | 769 | 27 | 248 | 13500 | 2375 | 123 | 125 | 309 |
M1 C | 19.7 | 127.4 | 80.2 | 210 | 1114 | 1054 | 1957 | 14620 | 3198 | 827 | 37 | 820 | 18125 | 2263 | 193 | 183 | 867 |
M2 A | 38.6 | 56.0 | 62.0 | 212 | 957 | 807 | 776 | 9840 | 3234 | 723 | 41 | 332 | 14325 | 1970 | 159 | 165 | 360 |
M2 B | 44.0 | 60.0 | 78.5 | 242 | 1100 | 998 | 1076 | 10710 | 3481 | 766 | 53 | 424 | 12400 | 1480 | 198 | 280 | 396 |
M2 C | 48.8 | 61.3 | 75.5 | 244 | 771 | 755 | 1183 | 11020 | 3789 | 496 | 58 | 446 | 18175 | 2908 | 168 | 279 | 575 |
Holubov | |||||||||||||||||
H1 A | 19.2 | 141.0 | 59.8 | 207 | 1214 | 1880 | 1049 | 11220 | 2345 | 755 | 38 | 317 | 8600 | 1835 | 95 | 66 | 273 |
H1 B | 15.6 | 161.6 | 70.6 | 204 | 742 | 1666 | 1977 | 12340 | 3051 | 523 | 45 | 709 | 10175 | 1950 | 99 | 118 | 472 |
H1 C | 23.6 | 165.3 | 51.5 | 204 | 952 | 1392 | 1534 | 10570 | 3092 | 709 | 51 | 600 | 8250 | 1940 | 109 | 172 | 302 |
H2 A | 11.6 | 135.8 | 54.7 | 202 | 993 | 637 | 1084 | 13375 | 2948 | 653 | 2 | 275 | 8400 | 3345 | 129 | 24 | 294 |
H2 C | 2.7 | 172.8 | 74.0 | 201 | 948 | 315 | 2520 | 17570 | 3267 | 628 | 22 | 937 | 11175 | 1840 | 134 | 44 | 544 |
a AMF – amorphous/less crystallised Fe-oxides/oxydes de Fe amorphes ou mal cristallisés.
b CRF – well-crystallised Fe oxides/oxydes de Fe bien cristallisés.
The pH of the soils ranges from 4.7 to 7.6 in water, and from 4.2 to 6.4 in KCl, with values increasing with depth. The OC content ranges from 31 to 115 mg/kg in the topsoil with higher concentrations in Holubov soils, and decreases with depth. The sum of NH4Cl-extracted cations varies from 13 to 34 cmolc/kg; these values are typical for ultramafic soils [4,10,17,24,29]. The highest values were determined in the mineral horizons. Magnesium strongly dominates the exchange complex due to its release during the incomplete hydrolysis of weatherable minerals, with exchangeable concentrations varying from 8 to 22.4 cmolc/kg, i.e. 57 to 95% of the sum of NH4Cl-extractable cations. This increase of exchangeable Mg with depth may result from the downward leaching of Mg or enrichment by soluble Mg-bearing phases in mineral horizons. Soils are very poor in exchangeable Ca, ranged from less than 0.1 to 5.5 cmolc/kg, i.e. 0.5 to 35% of CEC, as a result of the weathering of Ca-poor bedrock and subsequent leaching. The highest content of exchangeable Ca was found in topsoil, pointing to biological recycling of Ca by the vegetation (Table 2). The particularly low Ca/Mg molar ratios and the low K contents are typical for ultramafic soils and are responsible for the low fertility of these soils. This ratio is particularly low in the profiles from the Holubov area (Ca/Mg < 0.100) (Table 2).
Table 3 lists the results of chemical analyses of soils. After Si, Mg is the predominant cation, followed by Fe and Al. Trace metals consist mainly of Cr (315 to 1880 mg/kg) and Ni (515–2520 mg/kg), pointing to their ultramafic origin (Table 3). The highest concentrations were found at Nové Dvory and at Holubov 1. The variations between soils reflect the variability of substitution of other cations (Fe, Mn, Mg) by Ni and Cr in the primary minerals. Their distribution does not exhibit the same trend in the studied pedons. Chromium concentrations in soils were lower than in bedrocks, except at Holubov 1, where a relative accumulation was observed, whereas the Ni contents in the soils were always lower than in the corresponding bedrocks at all the sites. Moreover, Ni increases with depth, whereas Cr trends are more variable with tendencies towards residual enrichment in the uppermost horizons. This highlights the higher weathering rate of Ni-bearing minerals in the topsoil and thus leaching of Ni to depth, whereas Cr can be considered to be less mobile.
Non-mafic minerals like quartz and feldspars are present mainly in topsoil; this observation probably corresponds to their eolian origin or to contamination by non-ultramafic material (nearby migmatitic/granitic orthogneiss or granulites of the Moldanubian zone of the Bohemian Massif, see [20]). Quartz is also present in C horizons in Mohelno soils that may correspond to a mixing by solifluction processes. The other identified minerals in soils are principally primary minerals derived from the bedrocks. Serpentine (lizardite) is the second commonest mineral unambiguously found in all profiles, particularly in mineral horizons. Chlorites (clinochlore) and amphiboles (glaucophane) are also present to a lesser extent. Occasionally, talc is detected in deeper soil horizons of profiles from Holubov. These minerals are known to be important scavengers of Ni in ultramafic soils [2,4,14,23,24]. Massoura et al. [17] found that amphibole, talc and serpentine were Ni-bearing minerals (0.2–0.3 atomic percentage) in the Mohelno samples (here M1), whereas other clay minerals did not contain Ni. Kierczak et al. [14] found Ni in serpentine minerals, which are enriched in Ni compared to bedrock, and also in smectite. Mafic minerals (olivine and pyroxene) exhibit different behaviour in soil profiles for all the studied localities. At Nové Dvory, olivine (forsterite) is present only in bedrock and disappears in the soil horizons, in contrast to pyroxenes (enstatite, diopside), which are present in all the soil horizons. The same phenomenon was noticed at Holubov with only enstatite being detected (diopside is not present). This was also observed by Oze et al. [24], who showed that olivine is probably less resistant to weathering, compared to other potentially Cr-bearing phases (e.g. spinels). On the contrary, at Mohelno, pyroxenes are not present in the topsoil and forsterite is unambiguously present in all the soil horizons, particularly in the deeper ones. This indicates differences in weathering sequences between individual localities, due to some differences between bedrocks and microclimatic differences between localities. Spinels, which are important hosts for Cr, were not detected by XRD but observed by SEM (Fig. 4). Interestingly, Fe-(hydr)oxides, generally considered as important hosts for Cr and Ni in serpentine soils [14,17,24,27], were also not detected by XRD, but sequential extractions revealed that 29 to 49% of the Fe is extracted in the AMF and CRF steps, i.e. present in Mn and Fe-oxides (Table 3). Moreover, SEM observations, coupled to EDS analysis, revealed the presence of Fe- or Fe-Mn-(hydr)oxides as coatings in all samples (Fig. 4). These minerals are present as discrete phases with low crystallinity and, thus, are not revealed by XRD. They are supposed to adsorb cations, particularly at the pH values reported here, and also to incorporate metals in their lattice. Indeed, the mixed Fe-Mn (hydr)oxides systematically contained Ni, whereas other minerals never contained Ni (at the EDS quantification limit).Thus, the metals extracted by the reactants used to differentiate oxides are probably associated with the same Mn and Fe-bearing minerals (Table 3). Fe is mainly extracted in the AMF step (FeAMF/FeCRF ≥ 1), except in the Mohelno soils. This observation highlights the moderate weathering intensity of these soils, which favours the formation of amorphous or poorly crystallised Fe-(hydr)oxides compared to well-crystallised and reducible ones. Moreover, manganese seems to form an independent and discrete phase in these soils with MnAMF/MnCRF greater than 3. Indeed, acidified hydroxylamine hydrochloride is commonly used to dissolve Mn-oxides [3,22], whose presence is assumed in the studied soils, although not directly confirmed by XRD. These minerals are known to participate in the metal dynamics, particularly for Ni and Co [16,28]. Moreover, one has to notice that silicates are poorly dissolved during these extractions, as less than 2% of Si was extracted during each step.
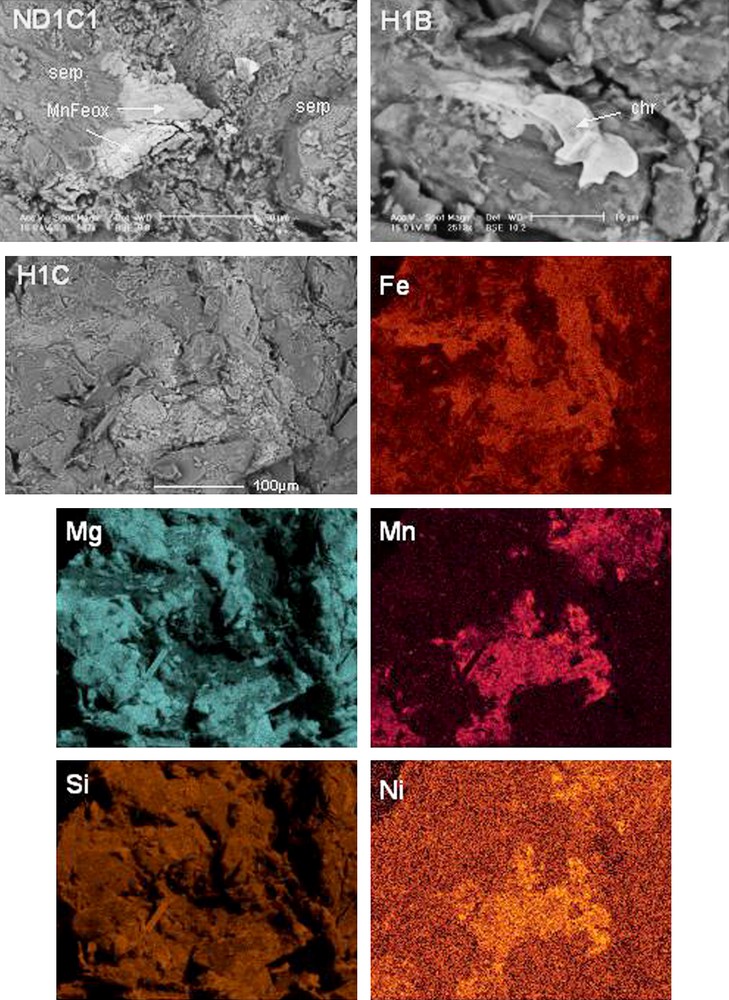
Backscattered electron microscopy images of soil samples and element mapping (serp: serpentine, MnFeox: Mn-Fe(hydr)oxide, chr: chromite).
Images en électrons rétrodiffusés des échantillons de sol et cartographie élémentaire (serp : serpentine, MnFeox : (hydr)oxyde mixte de Mn et Fe, chr : chromite).
The fraction of Ni extracted in the AMF and CRF steps is very high, ranging from 60 to 95% of Nitot. The residual Ni thus appears very low, suggesting transfer of Ni from primary to secondary minerals during weathering. To the contrary, 75 to 95% of Cr is not extractable and is thus mainly associated with recalcitrant minerals like silicates or trace spinels. It should be pointed out that the fraction of Cr and Ni associated with Fe-oxides is lower in soils from the Holubov area, suggesting that the weathering of the Cr- and Ni-bearing primary minerals was lower there. Nickel is associated more with amorphous Fe-(hydr)oxides (NiAMF/NiCRF ≥ FeAMF/FeCRF), whereas extractable Cr occurs mainly in well-crystallised Fe-oxides (CrAMF/CrCRF ≥ FeAMF/FeCRF) (Table 3).
3.3 Metal availability
The total metal concentration in soils provides a poor criterion for the assessment of the metal availability. As a result, we used the DTPA extractant as the best chelating agent to evaluate the phytoavailability of metals, particularly of Ni [6,15]. The Ni availability varies significantly amongst the samples, both in quantity and proportion (Fig. 5). The quantity of Ni extracted by DTPA ranged from 13 to 90 mg/kg, with the highest values in the surface layers (except for Mohelno 1). These concentrations are in the same order as measured in the Vosges ultamafic soils [4] and in soils from Taiwan [10], but lower than in some soils from New Caledonia [26]. The proportion of Ni extracted by DTPA was always higher in the surface horizons and reached 10% of Nitot in the Mohelno topsoil. The DTPA-extractable Ni was slightly higher in the Mohelno soils compared to other localities. Thus, the presence of adapted plants is likely in these natural areas, as is the presence of Ni-hyperaccumulators. According to Pearson’s coefficient, obtained from the correlation matrix of all the physicochemical data, NiDTPA is significantly and positively correlated (p < 0.05) to the Mn (0.707), MnAMF (0.540) and MnCRF (0.555) contents, to FeDTPA (0.492) and to MnDTPA (0.564). It is also significantly and negatively correlated with pHwater (−0.600). The latter is not surprisingly as the pH is a key parameter in controlling the metal mobility and availability [18,19]. In contrast to other studies [4,10,17], no correlation between NiDTPA and well-crystallized Fe-oxides was observed. This is not really surprising, the Ni bound in the well crystallised Fe-oxide lattices is probably not readily available. Nevertheless, Ni associated with amorphous Fe-oxides is mainly complexed at the surface and extracted by DTPA. These amorphous phases participate in Ni availability in soils where clay minerals are scarce. Moreover, manganese oxides, which are extracted in the same step, control Ni availability mainly during their bacterial reduction [28]. Thus, the role of Mn-oxides in the Ni availability should be further studied in detail.
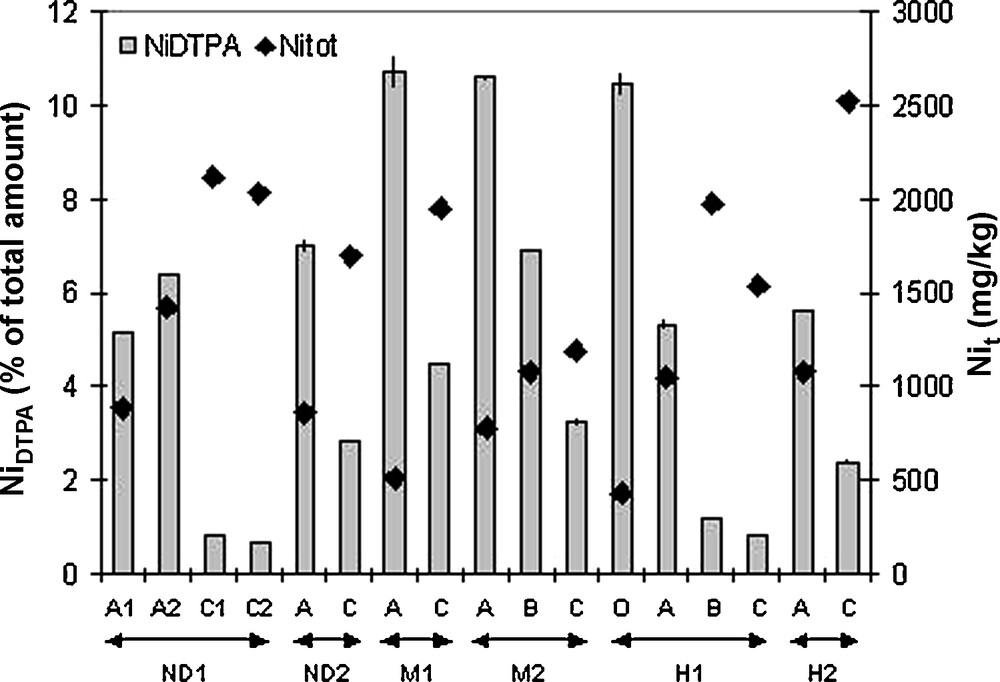
Nickel extractability with DTPA in the studied ultramafic soils.
Extractibilité de Ni par le DTPA dans les sols ultramafiques étudiés.
Unlike Ni, the DTPA-extractable contents of Cr were below the detection limit, and thus Cr can be considered not to be phytoavailable in these environments. Chromium is not extracted by DTPA, as it is mainly included in resistant mineral phases, which do not dissolve significantly during this extraction. Organic matter may have an impact on the Cr availability and mobility, particularly through the production of complexing agents that quite efficiently promote the dissolution of minerals and the formation of Cr (III) complexes [13,31], but this requires further investigations.
4 Conclusions
The limited weathering of serpentinised rocks in the Czech Republic has resulted in the formation of relatively shallow soils. These soils have fairly high pH values and high CEC dominated by Mg. The nickel and chromium contents are high, as commonly observed in ultramafic soils, but their availability and mobility are very different. Chromium is almost non-extractable and is mainly occluded in the structure of resistant minerals like spinels, silicates and well-crystallised Fe-oxides. Therefore, it is assumed to be relatively immobile and not phytoavailable. In contrast, Ni can be highly phytoavailable, particularly in topsoil, where hyperaccumulating flora can be found. The Ni dynamics appears to be partly controlled by pH and Mn-oxides.
Acknowledgements
This study was supported by the Barrande project (PAI KONTAKT 2-06-24). Institutional support of Charles University was provided by Ministry of Education of the Czech Republic (MSM0021620855).We wish to thank numerous colleagues for their assistance: Radek Procházka and Rémy Pichon (SEM/EDS), Emil Jelínek (fieldwork). Dr. Madeleine Štulíková is acknowledged for improving the English. Two anonymous reviewers significantly helped to improve the original version of the manuscript.