1 Introduction
Lake archives currently provide us with amongst the most continuous record of climate changes affecting continental areas since the Last Glacial Maximum (LGM). These geosystems are both directly influenced by human impact and global climate change. Lacustrine sediments, therefore, enable us to distinguish between global and local climate change effects. Besides, even if Jurassian lakes have been largely investigated for climate record, these investigations were conducted most of the time on records taken from the littoral areas [13,14] often affected by sediment hiatuses. Few studies were carried out on sedimentological features of sequences taken from deep part of lakes [2,20,25], although they provide continuous records without hiatus and, through high-resolution sedimentological studies, document both environmental and climatic changes. Due to its morphological features, potential infill and sensitive location close to the summit area of Jura Mountains, Lake Saint-Point appears to be an appropriate site for this kind of investigation, and notably for high resolution detrital inputs (silicates and carbonates) studies.
2 Geographical and geological settings
Lake Saint-Point (46°48.7 N, 6°12.2 E; 850 m a.s.l.) is located in the high chain of Jura Mountains. This lake (7 km2) is divided into two basins separated by a sill located at a depth of 21.5 m (Fig. 1). Depths in the upstream and downstream basins respectively reach 41 m and 35.5 m. The lake’s shape is forced by the synclinal structure of the Doubs Valley and the retreat of the LGM ice cap [5]. Lake Saint-Point is fed by the Doubs River, which supplies the main part of the suspended matter. Altitudes of its catchment (247 km2) range from 850 to 1463 m a.s.l (Mont D’Or) (Fig. 1). In this area, detritism-supplying geological layers correspond to Late Jurassic and Upper Cretaceous carbonates formations. In this context, lacustrine sedimentation is clearly dominated by both detrital and authigenic carbonates [2].
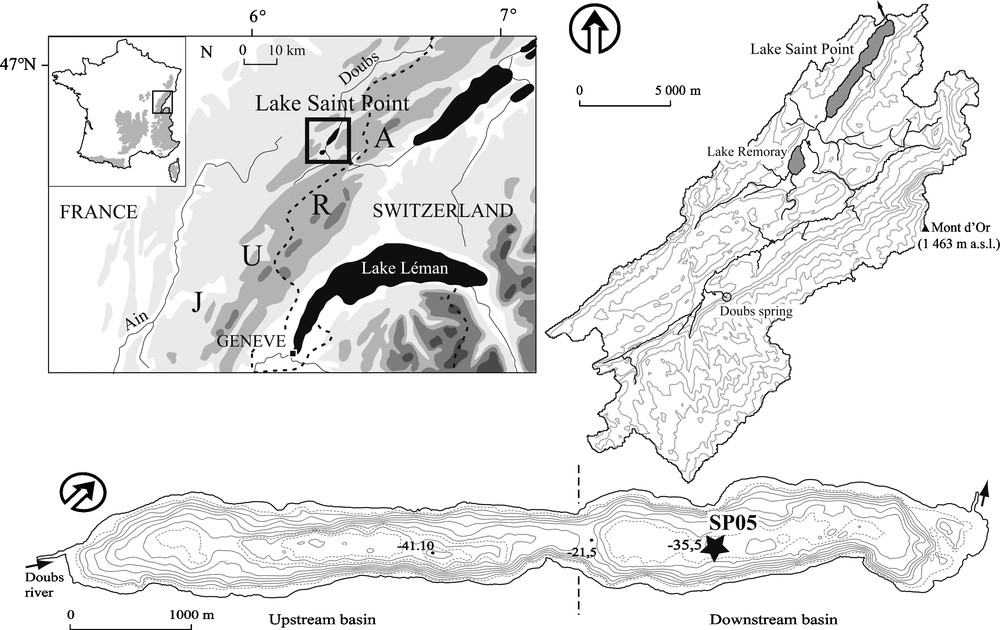
Location of Lake Saint-Point; topographic map of its catchment; bathymetric map and location of the core.
Localisation du Lac Saint-Point ; carte topographique du bassin versant ; carte bathymétrique et localisation du forage.
3 Material and methods
Prior to the coring, a seismic survey (seistec IKB high resolution) was carried out in order to highlight disturbance zones (slumps). Then, using seismic profiles, a continuous sequence (SP05; 12.5 m long) was cored in the central and deepest part of the downstream basin, at a depth of 35.5 m (Fig. 1). A coring platform (UWITEC system) was used to acquire the sequence SP05, which enabled us to extract 3-m long sections. Twin cores were taken in order to avoid problems linked to the transition between sections. Using these twin sections as well as correlations of obvious markers (lithofacies, laminae, tephra layers), a master core was reconstructed.
Chronology of the sequence is based on 24 AMS radiocarbon dates (Table 1) spread over the upper 7 m of the core. Under 7 m depth, no matter was available for radiocarbon datation. All the dates were determined thanks to leaves and wood fragment analyses, and calibrated using the program CALIB 5.1 [19]. The chronology was completed by pollen analysis made on punctual samples (regional pollen stratigraphy), particularly for the oldest part of the sequence (Oldest Dryas limits), and by the geochemical and mineralogical identification of the Laacher See Tephra (LST) [4,9,23,24]. Another tephra was observed during the Early Holocene but remains non-identified because of the size of the volcanic shards, which hampers any chemical analysis with electron microprobe techniques. Nevertheless, its chronological position could be compatible with the Early Holocene Vasset-Killian eruption, from the Massif Central, which has been recently described in Switzerland [3].
AMS radiocarbon dates 137Cs results obtained from core SP05
Datations AMS radiocarbon et résultats du 137 Cs obtenus à partir de la carotte SP05
Daiations methods | Depth (cn) | Radiocarbon date (BP) | Ages 2σ range (y cal. BP) | Laboratory reference | Material |
137Cs | 6.5 | – | −36(1986) | – | Bulk sediment |
137Cs | 10.5 | – | −13(1963) | – | Bulk sediment |
14C | 125 | 1120 ± 30 | 956–1088 | Poz-17040 | Leaves |
14C | 126.5 | 1100 ± 30 | 952–1062 | Poz-17041 | Leaves |
14C | 127.5 | 1155 ± 30 | 979–1150 | Poz-17041 | Leaves |
14C | 157.4 | 1775 ± 35 | 1604–1817 | Poz-17043 | Leaves |
14C | 157.6 | 1785 ± 35 | 1613–1818 | Poz-17051 | Leaves |
14C | 185 | 2145 ± 35 | 2033–2305 | Poz-17055 | Leaves |
14C | 190.5 | 2230 ± 35 | 2152–2336 | Poz-17057 | Leaves |
14C | 192.5 | 2255 ± 35 | 2155–2344 | Poz-17068 | Leaves |
14C | 193.5 | 2195 ± 35 | 2124–2324 | Poz-17053 | Leaves |
14C | 198.5 | 2490 ± 30 | 2457–2725 | Poz-17054 | Twigs |
14C | 219.5 | 2800 ± 35 | 2839–2993 | Poz-17067 | Leaves |
14C | 220 | 2975 ± 35 | 3059–3319 | Poz-17065 | Leaves |
14C | 246.5 | 3360 ± 35 | 3483–3689 | Poz-17064 | Leaves |
14C | 250.5 | 3500 ± 35 | 3688–3871 | Poz-17062 | Leaves |
14C | 271.5 | 3740 ± 35 | 3982–4160 | Poz-17063 | Needle |
14C | 278.5 | 3935 ± 35 | 4281–4513 | Poz-17061 | Leaves |
14C | 290.5 | 4130 ± 35 | 4566–4821 | Poz-17060 | Leaves |
14C | 306.5 | 4460 ± 40 | 4960–5294 | Poz-17059 | Leaves |
14C | 337.5 | 4850 ± 40 | 5476–5657 | Poz-18354 | Leaves |
14C | 352.5 | 5150 ± 50 | 5748–5995 | Poz-18356 | Leaves |
14C | 483 | 6500 ± 50 | 7308–7507 | Poz-18353 | Leaves |
14C | 484 | 6470 ± 50 | 7276–7461 | Poz-18357 | Leaves |
14C | 501 | 6980 ± 50 | 7695–7932 | Poz-18315 | Leaves |
14C(LST)[9] | 671 | 11 230 ± 40 | 13 125 ± 560 | – | Terrestrial macrofossils |
14C | 683 | 11 730 ± 60 | 13 426–13733 | 60 | Leaves |
Concerning the recent period, 137Cs measurements [16,21] were processed on the 20 upper centimetres of the sequence. Pollen analysis is currently still in progress; therefore, only mid- and late Holocene data is presented in this paper.
Physical parameters (gamma density and magnetic susceptibility [MS]) were measured using a Geotek Multi Sensor Core Logger System with a step of 5 mm.
The bulk sediment was analysed for grain size analysis (laser granulometer, LS230 Beckman-Coulter), major elements contents (XRF) and mineralogy (DRX). The sampling step was adjusted to the depth-age model but due to the condensation of some periods, its temporal resolution ranges from 60 to 200 years/sample (Fig. 2) with a resolution lower than 100 years for the Holocene.
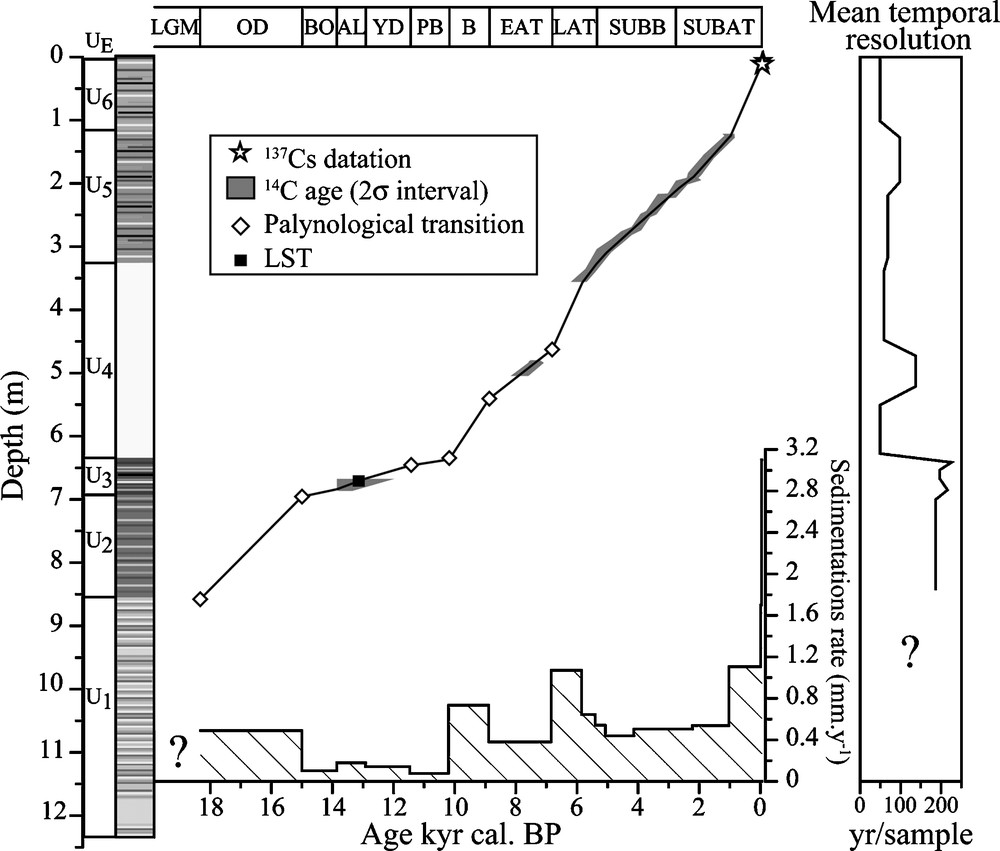
Age-depth model and sedimentation rates obtained from the different datation methods; sedimentological units; evolution of sample temporal resolution (LGM: Last Glacial Maximum [sensu lato]; OD: Oldest Dryas; BO: Bølling; AL: Allerød; YD: Younger Dryas; PB: Preboreal; B: Boreal; EAT: Early Atlantic; LAT: Late Atlantic; SUBB: Subboreal; SUBAT: Subatlantic).
Modèle âge-profondeur et taux de sédimentation obtenus à partir des différentes méthodes de datation ; unités sédimentologiques ; variation de la résolution temporelle des échantillons (LGM : dernier maximum glaciaire ; BO : Bølling ; AL : Allerød ; YD : Dryas récent ; PB : Préboréal ; B : Boréal ; EAT : Atlantique ancien ; LAT : Atlantique récent ; SUBB : Subboréal ; SUBBAT : Subatlantique).
4 Results
4.1 Depth-age model
The sequence documents the sedimentation from LGM (sensu lato) to current period (Fig. 2). Sedimentation rates greatly vary but stay between 0.07 mm years−1 (11 400–10 200 years cal. BP) and 3.1 mm years−1 (Modern period). The depth-age curve (Fig. 2) indicates quite similar sedimentation rates during the Late Holocene (from 6000 years cal. BP) as well as from 18 300–15 000 years cal. BP (Oldest Dryas). Very low sedimentation rates are observed from 15 000 to 10 200 years cal. BP (from the Bølling to the end of the Preboreal) prior to an increase beginning in 500 years cal. BP (the end of Late Atlantic pollen zone). The lowest sedimentation rates occur during the time interval 11 400–10 200 years cal. BP. Except for this particular period, sedimentation rates appear to be quite steady during the Holocene. Nevertheless, two increases are observed at 10 200 years cal. BP and 5400 years cal. BP. As for the highest sedimentation rates, they are recorded during the last century.
4.2 Sedimentological features
Based on sedimentological features, the sequence can be divided in six units showing very different colours (Fig. 2). Units 1 and 2 (U1 and U2) cover the end of LGM and the beginning of Late Glacial (till 15 000 years cal. BP-Oldest Dryas pollen zone). These well-laminated units are characterized by the presence of sandy levels which are not observed in other units. Unit 3 (U3) represents a very condensed sequence, spanning from 15 000 to 10 200 years cal. BP (from Bølling to the Boreal pollen zones). In this unit, the LST corresponds to a thin greyish level well known in other regional lacustrine records [4,11,24]. Unit 4 (U4) is a very particular facies made of unlaminated lacustrine chalk corresponding to the first half of the Holocene (8900–5400 years cal. BP). Units 5 and 6 (U5 and U6) can be eventually distinguished due to their organic matter content, which is higher than in the preceding units (Corg, Fig. 3).
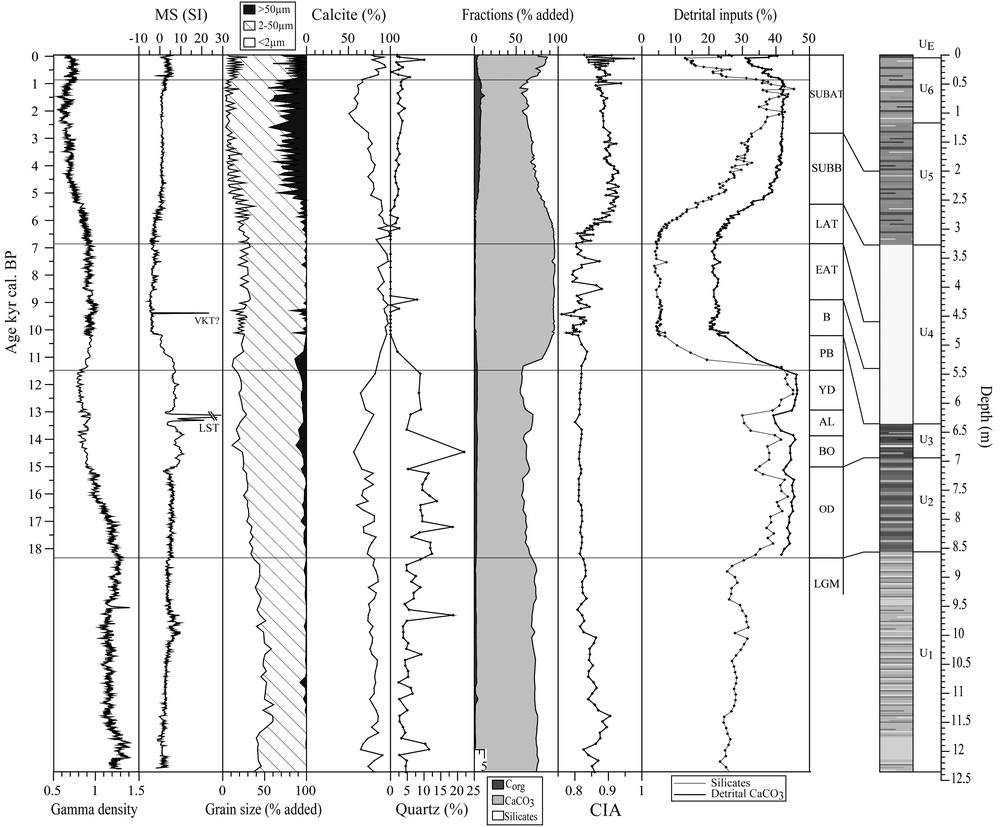
Sedimentological features: MS (for magnetic susceptiblility) and gamma density were measured using a Geotek Multi Sensor Core Logger System; calcite and quartz % resulted from DRX analysis; The carbonates, Corg and silicate contents were calculated thanks to major elements analyses results; CIA: Chemical Index of alteration; the detrital carbonates flux were worked out using Mg–Ca content. The horizontal lines indicate main sedimentological transitions.
Caractéristiques sédimentologiques ; MS et gamma densité ont été mesurés à l’aide d’un Geotek Multi Sensor Core Logger System ; les pourcentages en calcite et quartz résultent de l’analyse DRX sur roche totale ; les pourcentages en carbonates, Corg et silicates ont été calculés à partir des résultats de l’analyse des éléments majeurs ; CIA : indice d’altération chimique ; le flux de carbonates détritiques a été calculé à partir des teneurs en Ca–Mg. Les repères horizontaux indiquent les transitions sédimentologiques majeures. Masquer
Caractéristiques sédimentologiques ; MS et gamma densité ont été mesurés à l’aide d’un Geotek Multi Sensor Core Logger System ; les pourcentages en calcite et quartz résultent de l’analyse DRX sur roche totale ; les pourcentages en carbonates, Corg ... Lire la suite
The gamma density shows a progressive decrease with time, with two discreet shifts at the LGM-Late Glacial and Late Glacial-Holocene transitions (Fig. 3). The MS signal is characterized by two increasing trends separated by a strong decrease occurring during the interval 11 400–10 200 years cal. BP (Preboreal pollen zone) (Fig. 3). The two peaks observed correspond to tephra layers. Grain size analyses show that the thin (< 50 μm) fraction is dominant (Fig. 3). The coarser fraction (> 50 μm) sometimes appears during the Late Glacial but is observed all along from 6800 years cal. BP to the current period, with a progressive increase in U5 unit followed by a slight decrease at the top of U6 unit corresponding to the present time.
The mineralogy (Fig. 3) is clearly dominated by calcite (50 to 99%) associated to quartz and clay minerals, dolomite, plagioclase and feldspars only featuring as accessory minerals (less than 3%). From the LGM to 11 400 years cal. BP, calcite evolution (Fig. 3) appears to remain quite steady and increases from 11 400 years cal. BP on (Preboreal). The first half of the Holocene (11 400–5400 years cal. BP) is characterized by the highest calcite content. As for its second half, it shows a decreasing trend followed by another calcite content rise during the last millennium. These main periods are also emphasized by quartz content evolution (Fig. 3). From the LGM to 11 400 years cal. BP, quartz content shows pulses of high quartz inputs reaching about 20%. Quartz content is higher during Late Glacial than during the LGM. By contrast, no quartz input occurs during the first half of the Holocene (apart from two peaks at around 9000 years cal. BP). From 6500 years cal. BP on, quartz content increases but its proportion (∼3%) remains lower than those observed during the previous periods, except for the most recent period.
4.3 Geochemical features
The relations between Ca and Sr concentrations were used to characterize carbonates assemblages (Fig. 4). Their relations point out two different carbonated populations associated respectively to LGM/Late Glacial period and to the Holocene. The LGM/Late Glacial carbonates are characterized by high Sr values, whereas Holocene carbonates present lower Sr content. The correlations observed between Al2O3 and SiO2 also shows the occurrence of two silicated populations, associated to the same periods as observed in the carbonate fractions (Fig. 5). From the LGM to the Preboreal (11 400 years cal. BP), samples are richer in silica and poorer in alumina than Holocene ones.
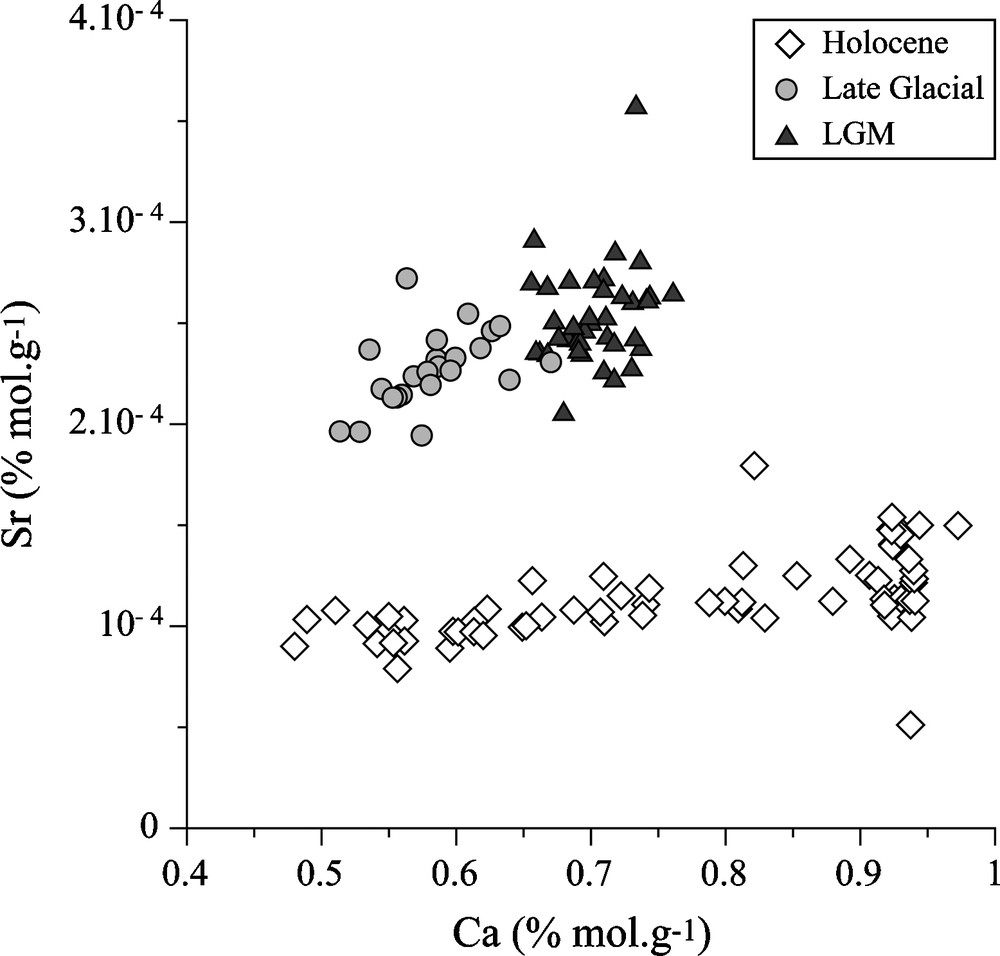
Relation between Ca and Sr concentrations (bulk sediment) expressed by periods (Sr-values were acquired by ICPMS analysis on bulk sediment).
Relation entre les concentrations en Ca et Sr (roche totale) selon les périodes (les valeurs Sr ont été obtenues par analyse ICPMS sur roche totale).

Linear relations between Al2O3 and SiO2 (bulk sediment) expressed by periods.
Corrélations linéaires entre les teneurs en Al2O3 et SiO2 (roche totale) selon les périodes.
The proportions of the different fractions (carbonates, silicates, organic carbon) were reconstructed from major elements analyses and LOI. Assuming that the Ca is mainly associated to the carbonate fraction, it is possible to work out the total carbonates content. The LOI. corresponds both to CaCO3 and organic matter, thus organic carbon can be worked out from LOI. measurement after correction of CaCO3 content. Silicate fraction was eventually calculated as the remaining fraction. The worked-out carbonate evolution is consistent with the calcite variations (Fig. 3) and shows a major transition at around 11 000 years cal. BP. The organic fraction rises up at 6000 years cal. BP but still remains lower than 5%. The silicate fraction is comprised between 4 and 45% (Fig. 3) and characterized by the same main variations as that of quartz content.
Major elements analyses were also used to calculate the CIA (chemical index of alteration, CIA = Al/(Al + K + Na + Ca*) in which Ca* corresponds to the plagioclase, negligible in this context) [15]. This indicator of pedogenetic alteration underlines three main periods (Fig. 3). From the LGM to the Preboreal (11 400 years cal. BP), the CIA slightly decreases and shows high variations from 10 200 years cal. BP to 6800 years cal. BP. A continuous increase occurs from 6800 years cal. BP prior to a slow decrease until the Modern period. From the last millennium on, its variation appears quicker and more important.
4.4 Detrital carbonate input calculation
Mg is associated to the detrital carbonates coming from catchment (Doubs water composition only enables the precipitation of pure calcite or very low-Mg calcite [6]) and, as a consequence, it is used as an indicator of detrital carbonates input.
Since the plagioclase and dolomite inputs are negligible from the Late Glacial onwards, the detrital carbonate input was worked out using the linear regressions observed between Ca and Mg (Fig. 6). The Ca content of theoretical authigenic carbonates was calculated for the different periods. Assuming that the detrital carbonate pool corresponds to the highest Mg contents, the composition of the detrital carbonate pool was calculated using chemical data together with chemical analyses of the catchment rocks [1] (Fig. 6). Then, compositions of theoretical authigenic (Caa) and detrital carbonates (Cad) pools, and Ca content in samples (Cas), were used to determine proportions of each fraction through a two components mixture calculation: Cas = f × Caa + (1– f) × Cad where f is the proportion of Caa in the mixture [12].
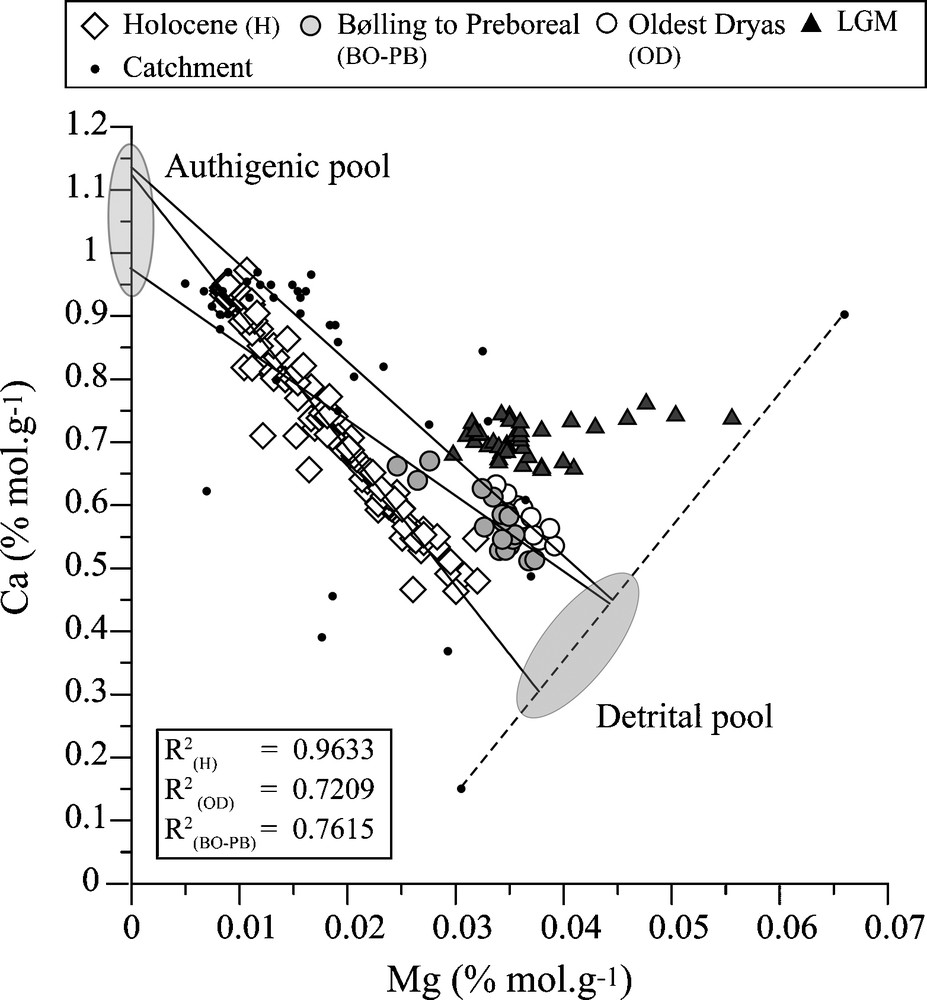
Linear correlations between Ca and Mg expressed by periods. The equations of linear regression supply the Ca-values of theoretical authigenic pools. The detrital pools were determined as the cross section between the linear regressions and the Ca–Mg limit composition from catchment samples.
Corrélations linéaires entre Ca et Mg suivant les périodes. Les équations des régressions linéaires fournissent les valeurs Ca des pôles de carbonates théoriques. Les pôles détritiques ont été déterminés comme étant les points d’intersection entre les régressions linéaires et la composition limite en Ca–Mg des échantillons du bassin versant.
The inputs of silicates and detrital carbonates (Fig. 3) underline the major transition previously observed. During the LGM and the Late Glacial, detrital inputs are high and represent around 80% of the sedimentation. These fluxes increase at the beginning of Late Glacial (18 300 years cal. BP). At 11 400, detrital inputs steeply drop, but the silicate input remains lower than the detrital carbonate one. At least, detritism rises up from 6800 years cal. BP reaching ca 70% before decreasing again during the last millennium.
4.5 Pollen data (from 7500 years cal. BP to recent period)
Presently, the first results in pollen analysis (Fig. 7) document the last 7500 years. Arborean pollen (AP) appears to be dominant (around 90%) throughout the whole sequence. Nevertheless, the proportion of AP pollen decreases from 3000 years cal. BP with a main drop from 1400 years cal. BP on. At 6800 years cal. BP, a change in vegetation assemblage is underlined through a change in the dominant species corresponding to the transition between early Atlantic and late Atlantic. Indeed, Quercus mixed decreases in favour of Abies and Picea. The drop of AP is synchronous with the main increasing phases underlined by anthropic indicators (AI, defined as the sum of Cerealia, Apophytes, Juniperus and Cannabis) (Fig. 7).
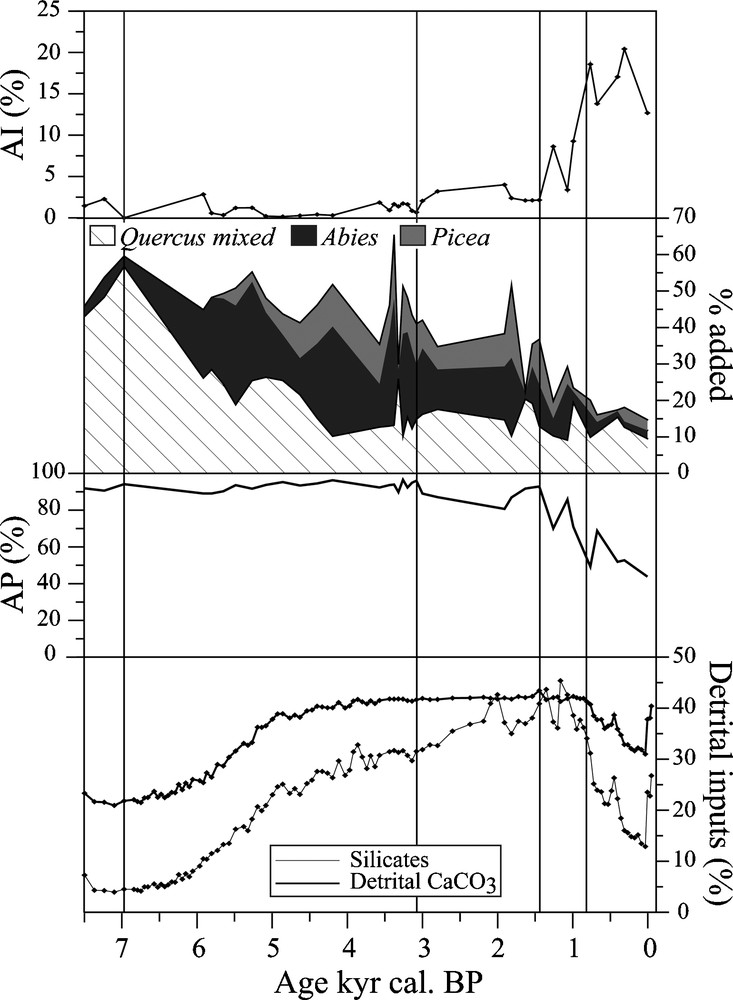
Detrital inputs compared to pollen data spanning from 7500 years cal. BP to Modern period; AP: arborean pollen; AI: anthropic pollen indicators (Cerealia, Apophytes, Juniperus and Cannabis).
Apports détritiques comparés aux données palynologiques couvrant les derniers 7500 ans cal. BP ; AP : pollen d’arbres ; AI : indice pollinique d’anthropisation (Cerealia, Apophytes, Juniperus and Cannabis).
5 Discussion
5.1 The LGM-Late Glacial transition (18 200 years cal. BP)
Prior to the Holocene, the carbonate signature is dominated by the catchment bedrock (Fig. 4). High quartz input may be partly inferred to bedrock insoluble residue and allochtonous inputs (Fig. 3). Indeed, previous studies [18,25] highlighted aeolians inputs in the Jura and in central Europe until the end of the Oldest Dryas. Considering no soil development affected the catchment during LGM due to the presence of the ice cap, the high CIA values indicate Lake Saint-Point was fed by aeolian soil particles reworked and mixed to the products of bedrock erosion. The slight decrease in CIA values from the LGM to the Late Glacial, may be explained as the erosion of older soils formed during the previous interstadial. From 18 300 years cal. BP, the increases in quartz content and silicate inputs are the consequences of the deglaciation through a change in water input and the outcropping of new areas where aeolian particles deposited. According to Buoncristiani and Campy [5], we infer that a transition from a subglacial position to a free of ice position of Lake Saint-Point took place at ca 18 300 years cal. BP.
5.2 The Late Glacial-Holocene transition (11 400 years cal. BP)
The second part of the Late Glacial (from 15 000 to 11 400 years cal. BP) is characterized by the lowest sedimentation rates (Fig. 2) probably due to a very low authigenic production and the depletion of the deglaciation products, although detrital contributions remain high (80%, Fig. 3). In contrast with this preceding period, the beginning of the Holocene corresponds to a steep drop in detritism fluxes and an increase in sedimentation rates (Figs. 2 and 3). These evolutions involve a huge increase in authigenic carbonate production at ca 10 200 years cal. BP. The low-Sr values in carbonates (Fig. 4) observed during the Holocene confirm this assumption. The high frequency CIA variation (Fig. 3) and the enrichment in Al2O3 (Fig. 5) indicate more efficient and active pedogenetic processes in the catchment. This evolution is the consequence of the forest development directly subsequent to the beginning of the Holocene warming. Indeed, previous palynological studies [7,17], mentioned a rapid transition from a steppe (Younger Dryas) to a forest composed by mesothermophile species (e.g. Pinus and Betula) at the Preboreal (11 400–10 200 years cal. BP). As a consequence, the erosion became less efficient [22]. Moreover, this period corresponds to an important hydrologic crisis in different Jurassian lakes with low lake-levels [13] and reduction of detritism [2]. The huge decrease in quartz flux (Fig. 3) is probably the consequence of the aeolioan depletion. This transition appears as a main change in environmental and climatic conditions.
5.3 The Late Holocene variations (6800 years cal. BP to the actual period)
The environmental change occurring at 6800 years cal. BP appears more continuous than those observed at the beginning of Late Glacial and Holocene periods (Fig. 3). Both detrital inputs rise up from 6800 years cal. BP on. However, silicate input evolves faster than detrital carbonate input (Fig. 3). This evolution is obviously present in the CIA variation, which indicates a more efficient chemical alteration in the catchment through an increase in the index. A change in pollen assemblage occurred whereas pollen data do not indicate any change in forest importance (AP > 80%, Fig. 7). Abies and Picea develop at the expense of mixed oak forest (Quercus mixed, Fig. 7); this modification cannot be solely attributed to human activities as demonstrated by the low and punctual AI values (< 2.5%) (Fig. 7), but is probably the consequence of cooler climatic conditions [8]. Some previous palynological study in the area [7] at the same altitude, notably at Lake Remoray (Fig. 1), actually pointed out no large-scale human impact on the environment at the same period. This detritism evolution is the consequence of the change from a deciduous to a resinous forest through a change in soil production. Abies and Picea generate larger and more acidic soils enhancing carbonates dissolution, which enables the residual quartz concentration in soil and, thus, explains the increase in quartz content (Fig. 3). As a consequence, the carbonate dissolution efficiency and therefore detrital carbonates flux depend on the erosion rate. This explains the contrast observed between variations in detrital carbonate and silicate inputs which follows the Abies development. Besides, an increase in silicate input occurs and goes on after 3100 years cal. BP. According to a lake level and glacier fluctuation study [10], this period is characterised by an advance of alpine glaciers at ca 3000 years cal. BP consistent with high lake level in Jura Mountains, indicating an increase in hydrologic flux. This climatic reversal enhances detritism flux. Nevertheless, AI and AP (Fig. 7) start to rise up and to drop respectively at the same period. Deforestation and agricultural exploitation are also factors enhancing detritism. In this context, the increase in silicate input is the consequence of both anthropic and climatic effects.
Although the first continuous indicators for human activities pointed out by AP and AI appear at 3000 years cal. BP, they indicate a huge human development only from 1400 years cal. BP (Fig. 7). This anthropic impact progressively induces the covariation of both detrital fluxes and their decrease. This particular evolution of detrital input corresponds to a land use change through the development of pasturelands at the expense of cultivated areas (increase in Apophytes). Hence, we may consider human activities as the main factor for environmental change during the last millennium.
6 Conclusion and perspectives
The Lake Saint-Point provides a continuous sequence enabling the characterisation of the main climatic and environmental changes from the LGM to the actual period. This sequence underlines three different evolutions of detrital inputs directly/indirectly triggered by climate change and/or anthropic effect.
The Late Glacial transition corresponds to an increase in hydrologic fluxes due to the deglaciation. As a consequence, sedimentation is characterized by high detritism and an important contribution of eaolian particles (huge quartz content). The beginning of the Holocene indicates a drop of detrital carbonates and silicate inputs. This period corresponds to the huge forest cover development observed in different Jurassian areas directly subsequent to the Holocene warming. The 6800 years cal. BP transition highlights the rise up of detrital inputs associated to a change in pollen assemblage. This period corresponds to the development of Abies at the expense of Quercus, thereby inducing a change in pedogenetic processes and thus a change in silicate production. Considering the low value of AI, the Abies development is probably the consequence of cooler climatic conditions. The last millennium is the most affected by human activities (as demonstrate by AI increase) despite the overall decrease in detrital inputs. This particular evolution is the consequence of a land use change through the development of pasturelands over previously cultivated areas.
Considering the different results, vegetation changes appear as the main factors of change in sedimentation feature through their natural or anthropic modification. The reconstruction of detrital carbonates requires taking into account pedogenetic processes. Silicates detritism flux eventually must be carefully interpreted in carbonates system.
Acknowledgments
This work was supported by the City of Besançon and the CNRS JurAlp project. We thank M. Desmet and the Edytem Laboratory (Chambéry) for supplying the coring platform. We also thank P. Capiez for XRF analyses and J.-P. Simonnet for his precious help in granulometry analysis.