1 Introduction
This study emphasizes the importance of the quartz c-axis fabric and associated microstructural observations, to infer temperature conditions during shearing and to define contexts favouring the development of quartz ribbons during HT deformation of granitoids.
In polycrystalline quartz ribbons, in which grains are subrectangular and range from equant to elongate in the direction of the X-axis (type B3 of [7]), a strong crystallographic preferred orientation is generally observed. According to Mackinnon et al. [28], in straight gneisses that experienced non-coaxial deformation under granulite to amphibolite facies conditions, quartz in polycrystalline ribbons of type B3 exhibits a strong maximum parallel to Y. In a similar ribbon type from striped gneisses, Hippertt et al. [22] suggest that ribboning is accompanied by the strengthening of c-axis maxima parallel to Z, a fabric indicative of plastic flow by basal <a> glide under dry and HT (ca. 700 °C) conditions. In addition, the latter authors consider that the progressive ribboning was characterized by the stretching of quartz grains, due to crystal–plastic processes during deformation.
According to Garbutt and Teyssier [18], in both non-coaxial and coaxial deformation, prism [c] or basal <a> should initially be able to concentrate the c-axes in a point maxima at ca. 45° from X. During deformation, if shear is dominantly accommodated by prism [c] slip, a decreasing angle between the point maxima and the X-axis is expected. By contrast, if basal <a> slip dominates, the c-axes are expected to rotate toward the Z-axis. Hence, the activation of both <a> and [c] slip should result in four point maxima of c-axes, nearly symmetrically disposed with respect to X. As a consequence, according to Kruhl [24], the opening angle around Z in the XZ plane between two point maxima can serve as a deformation-related thermometer. The c-axis fabric for slip on the prism <a> system is identical for both coaxial and non-coaxial deformation, and it is typically characterized by the concentration of c-axes around Y [7,27,35].
Prism [c] has mainly been found as the dominant slip system at HTs (e.g. [17]), from ca. 650–700 °C up to 800 °C (e.g [4,6,29]). According to many authors the presence of water in the quartz lattice is a strong weakening contributor at geological strain rates. HT combined with a high partial pressure of water are required to further enhance [c] slip in quartz [4,5,29]. However, other authors suggest that [c] slip may be activated even at lower temperatures [18,30,32]. In particular, Okudaira et al. [32] estimate a temperature of 550–600 °C for the basal <a>-prism [c] transition in the presence of water in the quartz lattice.
Deformation taking place through dominant prismatic slip along <a> is observed at intermediate to HTs [37]. Coherently, Nicolas and Poirier [31] and Bouchez et al. [6] indicate that slip along both prism <a> and prism [c] are equally easy at temperatures of about 700 °C. Taking both temperature and pressure conditions into account, Kruhl [23] concludes that in dry conditions simultaneous prism [c] and basal <a> slips occur in the β-quartz stability field and that, in addition, prismatic slip occurs in the HT domain of the α-quartz field.
Late-Hercynian, HT shear deformation affects granitoids in the zone straddling the contact between the Sila Massif batholith and the host granulites (Fig. 1a). In this article, a segment of this regional HT shear zone is investigated close to the town of Mesoraca (Fig. 1b), where a continuous section allows to analyse the deformation in granitoids (Fig. 2).

a: geological sketch map of the Late-Hercynian units in the Sila Massif area; b: geological map and cross section of the southern Sila Massif, modified after [15,25]. The location of the studied section (Fig. 2) is indicated.
Fig. 1. a : carte géologique schématique des unités tardi-hercyniennes dans la région du massif de Sila ; b : carte et coupe géologiques du massif méridional de Sila (modifiée d’après [15,25]). La coupe étudiée (Fig. 2) est indiquée.
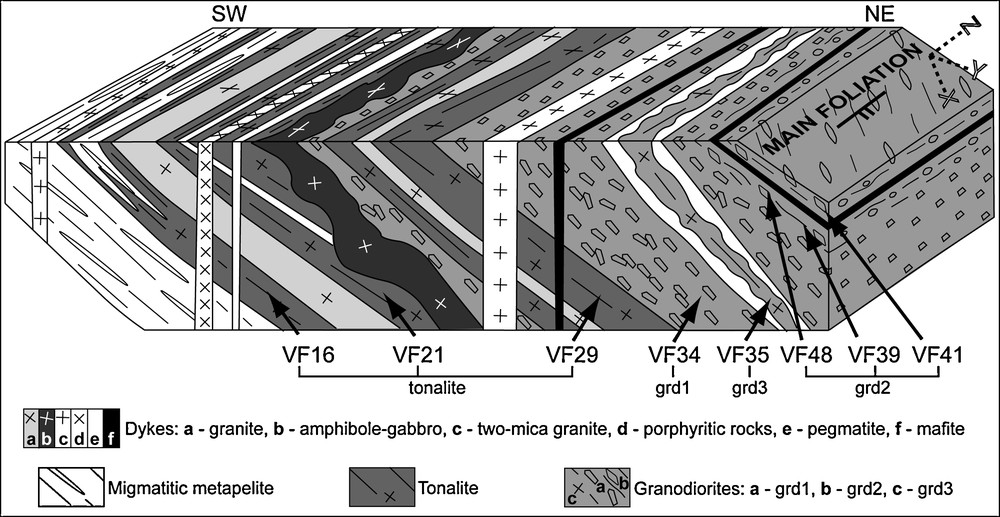
Block diagram showing the relationships among the different lithologies, as reconstructed along the section exposed close to the town of Mesoraca (see Fig. 1b for location). The length of the section is of about 1 km. Locations of the studied samples are indicated.
Fig. 2. Bloc-diagramme récapitulant les relations entre les différentes lithologies affleurant le long de la coupe étudiée (voir la Fig. 1b pour la localisation). La longueur de la coupe est d’environ 1 km. La localisation des échantillons étudiés est indiquée.
2 Geological setting
Two tilted, nearly complete Late-Hercynian continental crust sections are exposed both in the northern (Sila Massif) and central (Serre Massif) Calabria [8,14,33]. This continental crust is basically composed of three main parts, from bottom to top (Fig. 1a):
- • granulites, occupying the Hercynian lower crust;
- • Late-Hercynian granitoids, emplaced at mid-crustal levels;
- • low-grade metamorphic rocks belonging to the upper crust.
In the Sila Massif, these granulites, to the west (Fig. 1a and b), are mainly represented by migmatitic metapelite with intercalations of marble and metabasite, progressively more abundant toward the top [21]. The migmatitic metapelite equilibrated under granulite facies conditions, during a nearly isothermal decompression starting from peak P–T conditions at 600–400 MPa and 770–740 °C [19,20]; the age of peak metamorphism, obtained by U–Pb method on monazite, spans from 302 to 305 Ma [20]. These granulite facies conditions favoured dehydration reactions in the migmatitic metapelite, which involved both the white mica and the biotite [14,16,19].
The Sila Massif forms a composite Late-Hercynian batholith, whose composition spans mostly from monzogranite to tonalite. However, more mafic rocks, represented by norite, diorite and quartz diorite are also present [10]. Except for minor strongly peraluminous leucogranites, for which an origin by crustal anatexis is proposed [13], all the intrusive rocks come from hybrid magmas that received variable contributions from mantle and crustal sources [1,3,8]. Estimates of intrusion depths of some granitoids from the Sila Massif, obtained by Al-in-hornblende barometry, range from 8 to 18 km and confirm that the intermediate crust was a preferential site for magma emplacement [11,25]. In particular, P–T conditions of emplacement of granitoids in the Mesoraca area (Fig. 1b) are estimated at 490 ± 60 MPa and 710 ± 40 °C by Caggianelli et al. [11]. Using the 40Ar/39Ar method on hornblende and muscovite, ages ranging from 293 to 289 Ma have been derived for these plutonic rocks [3]. On the other hand, U–Pb dating on zircon and monazite indicates emplacement ages spanning from 304 to 298 Ma [20]. Finally, a white mica-whole rock Rb/Sr dating of an undeformed late pegmatite dyke provided an age of 265 Ma [25]. According to some authors, intrusion of magmas at mid-crustal levels took place during the Late-Hercynian, compressive tectonic regime [2,34], during the peak metamorphic event recorded in the migmatitic metapelite [19,20]. On the other hand, Caggianelli et al. [12] and Caggianelli and Prosser [9] suggest that intrusion of the granitoids is related to the extensional tectonics that took place after the thickening following the collisional stage of the Hercynian orogeny.
Late-Hercynian, HT shear deformation characterizes the zone straddling the contact between the foliated granitoids and the host granulites, near the town of Mesoraca (Fig. 1b). This shear zone, up to ≈ 5 km thick and ≈ 60 km long, strikes ≈ NW–SE and extends along the boundary between the granitoids and the underlying migmatitic metapelite [15] (Fig. 1a). Close to this boundary the meso- and micro-structures in the granitoids, indicate that deformation developed from melt-present to solid-state conditions. It is therefore likely that the deformation regime remained steady during magma crystallization and cooling in subsolidus conditions. A regional top-to-the-west sense of shear, in the present geographic coordinates, is recorded in the deformed granitoids [15,25,26].
3 Lithology, mesoscopic fabric and timing of emplacement
In the section examined (Fig. 2), as in other areas in Calabria, the transition from granitoids to granulites is represented by a migmatitic border zone. Away from the boundary, a wide spectrum of calc-alkaline intrusives crop out over about 1 km perpendicular to strike. They are mainly represented by granodiorites and tonalite with minor dykes of granite, amphibole–gabbro, porphyritic rocks, two-micas granite, pegmatite and mafite. The following distinctive textures allow to recognize three types of granodiorite:
- • carrying euhedral K-feldspar megacrystals up to 5 cm in length (grd1);
- • dark, heterogranular with coarse rounded plagioclases and K-feldspars in a fine-grained biotite-rich matrix (grd2);
- • with a nearly homogeneous grain size (grd3).
Tonalite is heterogranular with rounded to subhedral plagioclase grains up to 2 cm in length.
The granodiorite of types grd1 and grd2, and the tonalite are strongly foliated, whereas grd3, the granite and the gabbro are weakly deformed. The foliation of grd1 is pervasive, magmatic in origin and defined by the alignment of biotite flakes and euhedral K-feldspars surrounded by a fine-grained matrix made of millimetric grains of quartz and biotite (Fig. 3a). Similarly, in few cases the tonalite shows alignments of subhedral plagioclases in a fine-grained matrix made of millimetric biotite and quartz grains. The foliation of grd2 and most of the tonalites is spaced, represented by a layering highlighted by composition and grain-size variations. In particular, centimetre-thick leucocratic layers of stretched quartz and rounded feldspars, up to 1 cm in size, alternate with millimetre- to centimetre-thick layers enriched of fine-grained biotite (Fig. 3b). Both foliation types plunge 60° towards N60°, similarly to the foliation of the host granulites. The magmatic lineation is defined by the alignment of igneous K-feldspars or subhedral plagioclases, in grd1 and in a in few sites of the tonalite, respectively. A stretching lineation, given by elongate quartz grains and aggregates of quartz and feldspars, is present in grd2 and in most tonalites. Both types of lineation plunge on average 45° towards N70°.

Examples of foliations: a: well aligned euhedral K-feldspars defining a continuous, magmatic foliation in grd1; b: spaced foliation represented by layering highlighted by composition (dark biotite-rich layers alternating with quartz–feldspar-rich ones) and grain-size variations in grd 2; c: rim of pegmatite surrounding the grd3; d: chilled margin between the grd3 and the pegmatite rim.
Fig. 3. Exemples de foliations: a : cristaux automorphes de feldspath potassique définissant une foliation magmatique continue dans grd1 ; b : foliation espacée représentée par des variations de composition (niveaux sombres riches en biotite, qui alternent avec des niveaux riches en quartz et de feldspath) et de granulométrie dans grd 2 ; c : niveau de pegmatite autour de la grd3 ; d : « chilled margin » entre la grd3 et de la pegmatite.
The chronological sequence of emplacement is given by the field cross-cutting relationships. Grd1 and grd2 granodiorites were the first magmatic bodies to be emplaced, followed by grd3 and the tonalities, which injected the former granodiorites while still containing a residual melt. In particular, the grd3 intrusion is characterized by a rim of a thick pegmatite layer (Fig. 3c) with chilled margins (Fig. 3d).
The whole sequence was subjected to a top-to-the-west, Late-Hercynian shearing, including the granulites wall rock, under similar kinematic conditions. The other rock-types namely the granite, amphibole-gabbro, pophyritic rocks, two-micas granite, pegmatite and mafite, belong to the dykes that cross-cut their host, namely the migmatitic metapelite, granodiorite and tonalite. Being mostly undeformed, they are naturally considered as post-tectonic.
4 Quartz microstructures and c-axis fabrics
Microstructural observations and c-axis measurements have been carried out on the granodiorite and the tonalite, which are the most representative and the most intensely deformed rock types of the section under study (Fig. 2). The axes of the finite strain ellipsoid (X: stretching lineation, Z: normal to foliation and Y: perpendicular to XZ) will be used as the reference frame.
In grd1, grd2 and in the tonalite, quartz grains are anhedral and mainly occur in the form of polycrystalline, straight ribbons 1 mm to 4–5 mm thick (Fig. 4a–c) and up to 10 cm in length, that often tend to wrap around coarse rounded feldspars (Fig. 4a and c). Grain boundaries are sutured or straight, often almost perpendicular to ribbon elongation (Fig. 4a–c). In some cases, these boundaries tend to be arranged in triple junctions with interfacial angles of approximately 120° (Fig. 4c). Grain shapes are subrectangular and range from equant to elongate in the X direction. According to the classification of Boullier and Bouchez [7], these polycrystalline ribbons could belong to their type B3.
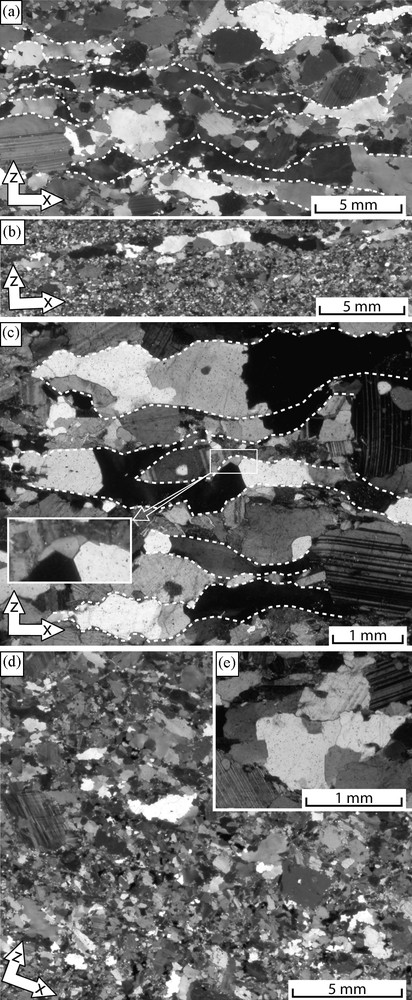
Microphotographs showing typical quartz microstructures in a series of samples located on Fig. 2. a: Grd1: polycrystalline quartz ribbons (sample VF34). Discontinuous white lines indicate their margins. Ribbons alternate with biotite- and feldspar-rich layers; b: Grd2: polycrystalline quartz ribbon in a fine-grained biotite- and feldspar-rich matrix (sample VF41); c: tonalite: polycrystalline quartz ribbons (sample VF21). Discontinuous white lines indicate their margins. Ribbons alternate with biotite- and feldspar-rich layers. Triple junction between quartz grains with interfacial angles of approximately 120° has been enlarged; d: Grd3: anhedral quartz occurring mainly as isolated crystals (sample VF35); e: Grd3: aggregates of grains with sutured boundaries (sample VF35).
Fig. 4. Microphotographies montrant les microstructures typiques du quartz dans une série d’échantillons localisée sur la Fig. 2. a : Grd1 : rubans de quartz polycristallin (échantillon VF34). Les pointillés blancs soulignent leurs contours. Les rubans alternent avec des lits riches en biotite et feldspath ; b : Grd2 : ruban de quartz polycristallin dans une matrice à grain fin riche en biotite et feldspath (échantillon VF41) ; c : tonalite : rubans de quartz polycristallin (échantillon VF21). Les pointillés blancs soulignent leurs contours. Les rubans alternent avec des niveaux riches en biotite et feldspath. Un exemple de point triple à environ 120° entre grains de quartz a été agrandi ; d : Grd3 : quartz xénomorphe formant des cristaux isolés (échantillon VF35) ; e : Grd3 : agrégats de grains avec bords suturés (échantillon VF35).
In type grd3, quartz mainly occurs either as millimetric isolated, anhedral grains or as aggregates of grains with sutured boundaries, and rarely forms millimetre-long ribbons. These ribbons, however, participate to define a weak foliation (Fig. 4d). The quartz grains that form isolated crystals or aggregates of recrystallized grains (Fig. 4e) exhibit a wide range of microstructures such as chessboard patterns, prismatic sub-boundaries, deformation bands, deformation lamellae and undulose extinction. Such grain types are rarely present in grd1, grd2 and tonalite.
Quartz c-axis measurements were performed from XZ sections prepared from 8 selected samples (located on the diagram of Fig. 2) using a universal stage. Quartz crystals, from either the ribbons of the three ribbon-bearing (tonalite, grd1, grd2), or from isolated or aggregated grains of grd3 were measured.
The resulting fabrics, plotted for each specimen in a lower hemisphere equal-area diagram, exhibit point maxima in-between X and Z (in the XZ plane). However, they are different from each other by their opening angles around Z, as shown on Fig. 5. On the one hand, point maxima with opening angles larger than 110° characterize the tonalite and grd3 (Fig. 5a–d); on the other hand, by contrast, values lower than 95° are characteristic of grd2 and grd1 (Fig. 5e–h). The lower intensity contour lines around these point maxima commonly delimit areas with an elongated shape between X and Z axes, covering angles up to 80°. Point maxima inclined at low angles (less than 45°) to X, are compatible with the dominance of prism [c] slip in tonalite and grd3 (Fig. 5a–d). Whereas, point maxima inclined at angles larger than 45° to X, are coherent with the dominance of basal <a> slip in most grd2 (Fig. 5e and f) and grd1 (Fig. 5h). A competition between prism [c] and basal <a> glide could be invoked for sample VF48 of grd2 (Fig. 5g), that records point maxima inclined at about 45° to X.
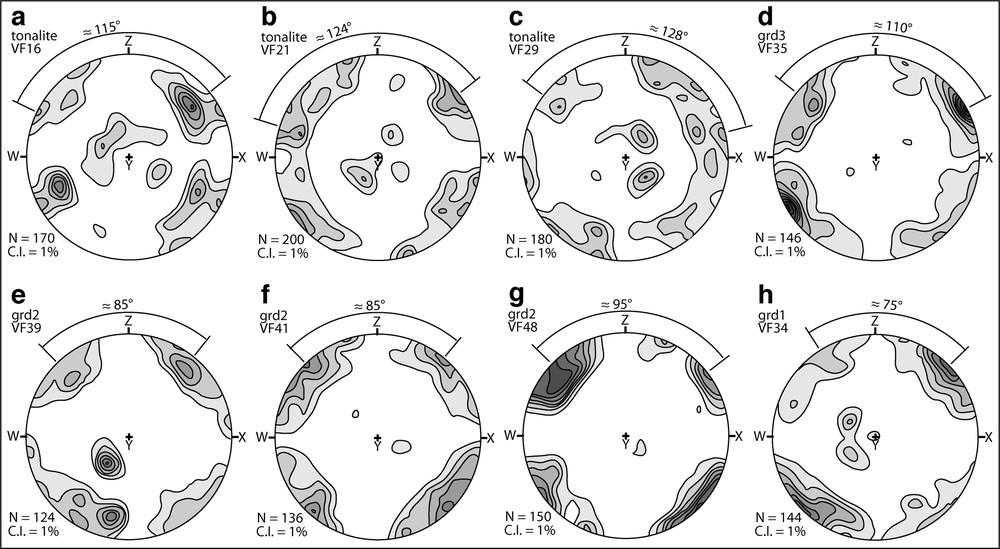
Quartz c-axis preferred orientation diagrams (Schmidt projection, lower hemisphere) of: a–c: tonalite (samples VF16, VF21, VF29); d: grd3 (sample VF35); e–g: grd2 (samples VF39, VF41, VF48); h: grd1 (sample VF34). Opening angles between maxima on sides of Z are indicated on the top of each diagram.
Fig. 5. Orientations préférentielles des axes c du quartz (projection de Schmidt, hémisphère inférieur) dans : a–c : tonalite (échantillons VF16, VF21, VF29) ; d : grd3 (échantillon VF35) ; e–g : grd2 (échantillons VF39, VF41, VF48) ; h : grd1 (échantillon VF34). L’angle d’ouverture entre les maxima situés de part et d’autre de Z est indiqué sur chaque diagramme.
C-axis concentration around Y, which point to prism <a> slip, are present in all c-axis fabrics, although they seem to be weaker in the grd3 than in the tonalite, grd2 and grd3 (Fig. 5)
5 Discussion
5.1 Deformation under granulite and amphibolite conditions
Temperatures of deformation were tentatively defined using the diagram of Kruhl [24] through the opening angle of the quartz fabrics (Fig. 6). According to this author, a linear increase of the opening angle with temperature is observed for greenschist and amphibolite facies conditions, during which <a> slip is dominant. The increase in opening angle becomes dramatic at temperatures of the granulite facies, during which prism [c] slip is activated. The best-fit relationship from the greenschist to the granulite facies (Fig. 6; in grey) reflects the ±50 °C uncertainty in the temperature of deformation. Although temperature plays the most important role in dictating the amount of [c] slip versus <a> slip in quartz [24], this degree of uncertainty covers the range of strain rates, hence of stress magnitudes applied, but also of hydrolytic weakening likely encountered in natural environments. As a consequence, the observed opening angles larger than 110° (tonalite and grd3) strongly suggest that deformation took place in granulite facies conditions. Instead, the opening angles lower than 95°, characteristic of grd2 and grd1, point to deformation in the amphibolite facies conditions (Fig. 6).

Opening angle versus temperature. The thick shaded line represents the best-fit relationship of Morgan and Law [30], modified from the diagram of Kruhl [24].
Fig. 6. Angle d’ouverture en fonction de la température de déformation. La zone en grisé représente le meilleur ajustement selon Morgan et Law [30], par modification du diagramme de Kruhl [24].
By contrast, Morgan and Law [30] argue that at temperatures higher than ≈ 650 °C, the opening angle thermometer may be invalidated if deformation took place in hydrous conditions, which favour the dissolution of water into the crystal structure of quartz and promote [c] slip at the expense of <a> slip. This would cause a drastic increase of the opening angles, that could be taken for temperatures of deformation higher than the real ones. In the case of our granitoids, hydrous conditions could be related to water-rich fluids released by micas breakdown, which promoted partial melting in the host migmatitic metapelite [14,16,19]. These hydrous fluids dissolved in the granitoid magmas, which emplaced in the middle crustal level. In the formerly emplaced magmas dissolved water was responsible for the growth of K-feldspar megacrysts in the grd1 (Fig. 3a), and for the massive crystallization of biotite in the dark grd2 (Fig. 3b). On the other hand, in subsequently emplaced magmas, hydrous fluids possibly escaped before full crystallization and gave origin to pegmatites characteristically associated to grd3 (Fig. 3c). Therefore, the elevated quartz opening angle recorded in later granitoids was not determined by the presence of fluids during deformation. In our opinion it was related to deformation under granulite facies conditions according to the opening angle thermometer of Kruhl [24].
Summing up, the available data support that shearing deformed grd1 and grd2 during their cooling, until their equilibration under amphibolite facies conditions. The same tectonic event deformed younger and hotter grd3 and tonalite, and protracted until their equilibration in higher temperature conditions, at the time of amphibolite facies equilibration of older grd3 and tonalite.
5.2 The development of quartz-ribbons: significance of c-axis fabrics
Assuming that the deformation imprint was recorded by sequentially emplaced granitoids at different cooling stages, quartz microstructures in tonalite and grd3 should develop under higher grade conditions; by contrast, grd1 and grd2 types may be characterized by deformation under lower grade conditions.
Hippertt et al. [22] proposed that the change from scattered isolated grains to polycrystalline ribbon is the result of quartz segregation and subsequent coalescence. The anhedral isolated crystals or aggregates of recrystallized grains of grd3 (Fig. 4d and e) could record the stage in which ribboning started to develop by shearing under granulite facies temperatures (Fig. 7a). The few short polycrystalline ribbons could reflect an incipient ribboning in these rocks (Fig. 7a). In the corresponding c-axis fabrics, dominant prism [c] slip would explain the point maxima inclined at low angle (less than 45°) to X (Fig. 7a), in agreement with the HTs widely accepted for the deformation by prism [c] slip (e.g. [17,23,29]). Tonalite may record a more advanced stage of deformation under granulite facies conditions. The elongation of quartz grains parallel to X reduces the distance to the nearest grains, and it favours grain coalescence at the origin of ribbon development [22]. During ribbon development in the tonalite, quartz recrystallization and grain boundary migration (Fig. 4c) remained active attesting to HT conditions. In addition to the known influence of prism [c], the c-axis fabric of the tonalite points to prism <a> slip at the origin of a point maximum around Y (Fig. 7b), in agreement with relatively HTs of deformation [6,23,31]. Both slip systems (prism slip along <a> and [c] directions) could have taken place in the tonalite under granulite facies conditions, but we cannot exclude that the Y-maxima appeared during the amphibolite facies event, as now argued for grd1 and grd2 types.
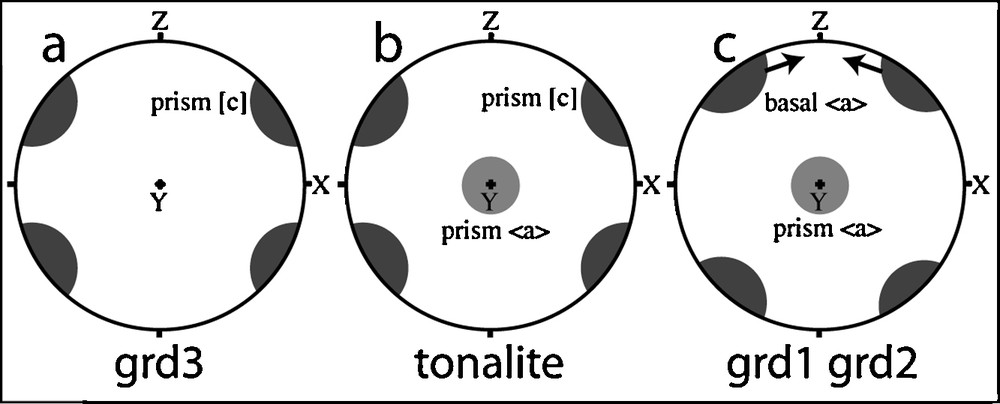
Model illustrating the development of quartz c-axis fabrics. a: incipient ribbons start to develop under granulite facies conditions. Prism [c] slip dominate and promote point maxima inclined at less than 45° to X. Grd3 may represent this case; b: quartz ribbons development under granulite facies conditions with the dominant activation of prism [c], helped by prism <a> slip. This situation is well preserved in the tonalite; c: basal <a> slip contribution increases in quartz during deformation under amphibolite facies conditions. The early-formed maxima due to prism [c] are rotated toward Z (arrows). Prism <a> slip system remains active. This situation is represented mainly by types grd1 and grd2.
Fig. 7. Schéma illustrant le développement des orientations préférentielles des axes C du quartz. a : la formation des rubans de quartz commencée dans les conditions du faciès granulitique, essentiellement par activation du système de glissement prismatique [c], qui favorise les maxima inclinés à moins de 45° de X. Grd3 pourrait représenter ce cas ; b : les rubans se développent principalement par activation du système de glissement prismatique [c], mais aussi prismatique <a> dans le facies granulitique. Cette situation est bien préservée dans la tonalite ; c :la déformation de plus basse température dans le faciès amphibolite favorise l’activation du système de glissement basal <a> entraînant un déplacement des maxima vers Z. Le système prismatique <a> (maximum Y) persiste. Cette situation est représentée principalement par grd1 et grd2.
A similar evolution of ribboning reasonably took place for the older grd1 and grd2 (Fig. 4a and b), when they deformed under highest temperatures. However, their fabric imprint suggests that the basal <a> slip system dominated during their deformation under amphibolite facies conditions (Fig. 7c). Garbutt and Teyssier [18] and Okudaira et al. [32] have described similar cases in which the early-formed prism [c] maxima rotated toward Z during the transition from prism [c] to basal <a> due to deformation at decreasing temperatures. Under these conditions, prism <a> slip, that forms the concentration of c-axes around Y (Fig. 7c), was still active, as also documented by Schulmann et al. [36] and Stipp et al. [37] in their research studies of basement rocks from the Bohemian Massif and from the Alps.
The c-axis fabrics showed in this study differ from some documented occurrences of ribbon-bearing high-grade rocks, in which the presence of a strong Y-maximum [28,36] or a strong Z-maximum [22] points to dominant prism <a> or basal <a> slip, respectively. Since we demonstrate that [c] slip was present at the origin of formation of the quartz ribbons; the absence of strong Y- or Z-maximum could be related to a deformation that was not intense enough at decreasing temperature for <a> slip systems to modify substantially the fabric.
6 Concluding remarks
C-axis fabrics and microstructural observations in syn-tectonic granitoids of the HT shear zone straddling the contact between the Sila batholith and the host metapelites support the following conclusions. Firstly, shearing favoured the formation of polycrystalline quartz ribbons in deformed granodiorite and tonalite. Secondly, slightly younger and hotter grd3 and tonalite experienced non-coaxial deformation under granulite facies conditions, whereas older and colder grd1 and grd2 recorded deformation at lower temperature, amphibolite facies conditions. Thirdly, the c-axis fabrics of the quartz ribbons exhibit weak point maximum around Y and strong point maxima in-between X and Z (in the XZ plane), differing from each other by their opening angles around Z: larger than 110° for tonalite and grd3 types, and lower than 95° for grd2 and grd1. As a consequence, the quartz ribbons, resulting from crystal–plastic segregation during non-coaxial shearing, were originally formed by prism [c] slip plus eventually prism <a> slip systems, under granulite facies conditions, then, under amphibolite facies conditions, by basal <a> plus prism <a> slip systems. The short duration of deformation under <a> slip (amphibolite facies conditions), attributed to a high cooling rate, explains the importance of the prism [c] slip imprint.
Acknowledgements
This study was supported by MIUR–PRIN 2007 research funds (to R. Spiess, Università di Padova). I am grateful to J.L. Bouchez whose suggestions and comments helped me to greatly improve the original manuscript. Critical review by an anonymous reviewer is also appreciated. J. Touret is acknowledged for his precious editorial work and helpful suggestions. Finally, I wish to thank A. Caggianelli for his encouragements in writing this paper.