1. Introduction
The International Panel on Climate Change (the IPCC is a body of the United Nations) confirms in its new report of 6 October 2018 (the famous "Summary for Policymakers") that nuclear power is part of the solution to limit global warming. It had already written this in its previous report presented on 27 September 2014.
The prevalence of nuclear energy in the French electricity mix makes us a top performer in terms of carbon-free electricity (Table 1).
Yet 69% of French people believe that nuclear power plants emit CO2. A responsible state must start by informing citizens, and should correct this misperception. The Ministry of the Environment has never said that nuclear power emits practically no CO2, and recently the Minister of the Environment announced that the state was fighting global warming by closing a coal-fired power plant, … and the nuclear power plant in Fessenheim! Yet, the figures are there, they are stubborn, they are known: the CO2 intensity of the different sources of electricity (Table 2) is 16g/kWh for nuclear power, comparable to wind (15) and biomass (18), lower than solar (22 to 48), and much lower than gas (491), oil (840) and coal (1024).
CO2 emissions per capita, all activities combined for G7 countries and China
Country | CO2/capita (tonnes) |
---|---|
France | 4.32 |
Italy | 5.29 |
UK | 6 |
Germany | 8.82 |
Japan | 9.35 |
USA | 15.57 |
Canada | 15.7 |
China | 6.59 |
CO2 emissions by electricity generation technology. Results obtained under the EU External Programme (Berger A. et al., Int. J. Global Energy Issues, Vol. 40, Nos. 1/2, 2017)
Method | Coal | Gas | Hydro | Wind | Solar | Nuclear |
---|---|---|---|---|---|---|
g CO2/kWh | 1,024 | 491 | 6 | 15 | 45 | 16 |
Number of deaths calculated for the production of 1000 TWh (twice the annual French consumption) from observed data for different electricity production methods (Berger A. et al., Int. J. Global Energy Issues, Vol. 40, Nos. 1/2, 2017)
Method | Coal | Oil | Gas | Biomass | PV | Wind | Hydro | Nuclear |
---|---|---|---|---|---|---|---|---|
Deaths/1000TWh | 170,000 | 36,000 | 4,000 | 24,000 | 440 | 150 | 1,400 | 90 |
It does not take a rocket scientist to understand that, in terms of reducing CO2 emissions, it makes no sense to replace nuclear electricity with electricity from photovoltaics or wind power, and that the use of gas or coal, as is done in Germany to stabilise power grids jeopardised by intermittency, is a cause of increased CO2 emissions.
One never builds solidly on lies. We can decide to "phase out of nuclear power" for a variety of reasons, mainly political, but we cannot say that we are doing so in the name of the fight against global warming.
In the first part, we shall endeavour to re-establish some scientific facts that decades of anti-nuclear propaganda have helped to obscure for the general public, in the media, and in the political class. We shall begin by recalling the principle of operation of nuclear power plants, the risks they entail, the issue of the fuel cycle, which is essential in the management of resources and waste, and the issue of dismantling power plants. In each of these issues, there are falsehoods circulating that must be denounced, so that the choices to be made can be made in full knowledge of the facts.
In a second part, we will address the "systemic" issues of surface land use, material balance, coexistence between different sources of electricity, and the issue of integrated cost.
2. Restoring scientific truth
2.1. Operation of nuclear power plants and associated risks
The principle of nuclear energy generation is simple: a naturally occurring fissile radioactive element (the isotope uranium-235 in uranium power plants) can be split into two fragments of lower atomic mass and emit two to three neutrons, which in turn can split other atoms: this is the principle of the chain reaction. The sum of the atomic masses of the fission products is lower than the mass of the initial fissile nucleus, and the "mass defect" is converted into energy, mainly heat. This heat heats a "heat transfer" fluid that produces steam which turns turbines that produce electricity. In order to maintain these "chain" reactions, it is necessary both to increase the quantity of fissile nuclei per unit volume ("enrich uranium") and to control the speed and quantity of neutrons likely to propagate fission (this is the role of the "moderator" and the "control rods" respectively). The variety of fuels, moderators and heat transfer fluids leads to different "types" of reactors, each with their own disadvantages and advantages, and each corresponding to adapted technological options. For this reason, it is not possible to think of a reactor type and a fuel production system separately.
By far the most widespread type in the world is the so-called PWR (Pressurised Water Reactors): water is used both as the moderator and as the heat transfer medium. It is pressurised so that the temperature of the liquid water is around 350 °C which allows the steam generators to run the power turbines with greater energy efficiency. This system also allows to separate the primary circuit, whose water passes through the reactor core, from the secondary circuit which will supply the turbines.
The cultivated common fantasy is that a nuclear reactor can turn into an atomic bomb. This is simply not true: a 900 MW nuclear reactor core such as the ones we have in France contains 74 tonnes of low-enriched uranium (3%) in uranium-235. We are very far from the critical mass that can lead to a runaway chain reaction: a uranium atom can only cause the fission of one uranium atom, not several (which is linked to the number of possible interactions of neutrons with atoms before their elimination at the surfaces). The simplicity lies in this "neutron economy". Sometimes a uranium atom absorbs a neutron to produce a plutonium atom, but this only occurs in 1% of cases of uranium-235 neutron-atom collisions. In the event of a serious accident, it is simply not possible to "concentrate the fissile species" (uranium-235 and plutonium) and the transformation of a nuclear power plant into a nuclear bomb is pure nonsense.
While such a risk is impossible, it is nonetheless true that nuclear power is a demanding technology and that accidents are possible. The accidents in Windscale (UK), Chernobyl, Fukushima led to explosions and fires, but these were thermochemical causes and not an atomic explosion. The severity of these accidents is measured by the emission of radionuclides into the atmosphere. This is why current reactors have containment buildings to prevent the dispersion of radioactive products.
The taste for sensationalism, with a good dose of bad faith, has led to the posting huge numbers of deaths. Here again, the facts are stubborn and proven, validated by the International Energy Agency (which operates similarly to the IPCC) and by the WHO. The Windscale accident in the UK caused zero deaths, the Three Mile Island accident in the USA caused zero deaths, the Chernobyl accident in Ukraine caused 4,000 deaths and the Fukushima accident caused zero deaths. As this did not suit the dominant anti-nuclear ideology, the deaths from the Tsunami (20,000) were gradually attributed to the Fukushima accident and the radiation-induced deaths were inflated beyond all likelihood.
Even assuming the validity of the "linear no-threshold model" of the effect of radioactivity on humans, which attributes a number of deaths proportional to the radiation dose received, and which is probably an overestimate, the number of deaths per TWh of electricity produced remains very low, compared to gas, oil and coal (Table 3). If we take the number of serious diseases induced as an indicator (see for example A. Markandy, P. Wilkinson, The Lancet, (2007), 370, 9591, pp. 979–990 "electricity generation and health") we have quite similar results.
The setbacks of the French nuclear power industry have made the headlines (problems of faulty workmanship at the Creusot forges, welding at the Flamanville site, carbon segregation, etc.). We must not minimise these problems: they are a sign of a real loss of skill in France simply because we have not built power plants for 20 years. No industry would emerge unscathed from such austerity. No industry could live with the constant evolution and without technical justification of the regulations, in the very duration of a major project. We must seriously ask ourselves the question of the industrial viability of an activity subjected to such treatment, again for essentially political reasons. But it is important to know that none of the manufacturing errors have led to a real safety problem (other than what can be called "administrative safety"), simply because the safety margins are comfortable. And it is the existence of these margins that also leads the United States to extend their power plants beyond 60 years or even 80 years, while we stop ours at 40 years. All this has absolutely nothing to do with safety.
2.2. The fuel cycle: resources and waste (Figure 1)
It is a classic mistake to separate the issue of power reactors from that of fuel. The Westinghouse-licensed PWR type of reactor chosen by France instead of the graphite gas-type was linked to the capacity to manufacture enriched uranium. Similarly, the MOX reactor was developed after the SUPERPHENIX breeder reactor was abandoned, again for political reasons, in order to partially manage the plutonium produced and optimise the use of fissile material.

The fuel cycle in France.
Understanding the French nuclear power industry requires, first, taking into account the entire reactor fleet (those who may or may not accept MOX fuel), but also fuel fabrication plants, recycling of plutonium in MOX fuel, vitrification of final waste (La Hague site). If we shut down the reactors, starting with the oldest ones, we de facto shut down the MOX fuel industry, unless we make heavy investments in recent reactors to make them capable of using MOX.
It is often said that the unsolved problem of nuclear energy is its waste. Through constant repetition, it has become an accepted fact. Except that, once again, it is inaccurate. Nuclear waste is characterised by its radioactivity and half-life (i.e. the characteristic time for radioactive atoms to decay by half). Highly radioactive materials have a short half-life, and less radioactive materials have a long half-life.
Figure 2 shows the proportion of different types of waste after the use of 1 tonne of fuel (U02, enriched to 3%) for 3 years. The really problematic elements in the long term are actinides (plutonium and minor actinides).

Long-lived waste generated in one tonne of fuel after three years of operation.
The problem with waste is to effectively contain it in such a way that it cannot come into contact with humans until its radioactivity has been significantly reduced. Before reprocessing spent fuel, which is still thermally "hot", it is stored (for example at the La Hague site, or at Marcoule) for a few decades. Then they are "reprocessed" to make them "storable" waste for much longer periods of time, from a few hundred to a few tens of thousands of years. The technical solution exists: it consists in vitrifying the waste, with the vitrified waste packages easily containing the fission products which have a half-life of a few hundred years. Actinides (plutonium, americium, neptunium, curium … produced by the absorption of neutrons by the uranium nuclei) can be vitrified or separated for recycling, they have a longer half-life (of the order of a hundred thousand years). This corresponds to 20 times the duration of the oldest human civilisation on earth. It is therefore difficult to think of an engineering structure whose integrity can be guaranteed over such periods. This is why we rely on geology in non-seismic areas to ensure containment.
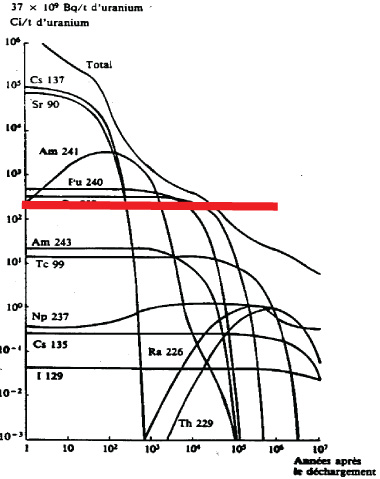
Decrease in radioactivity in Curie per tonne of waste stored as a function of time after unloading. The level of natural radioactivity is indicated by the horizontal line. This means that we must ensure the containment of the waste for periods of around 100,000 years.
The site of Bure in Meuse-Haute Marne, in the Paris Basin, with its clay cover ("callo-oxfordian") was selected for a deep geological repository. To be released from their glass "prison", the actinides must first diffuse out of the glass, but above all they precipitate when they enter the clay zone, i.e. they are trapped in small aggregates, or "stick" by sorption on the clays. Their migration is then very slow, so that the time required for them to “get out of their trap”, i.e. from the clay formation, is such that the radioactivity has fallen below its “natural” value (Figure 3).
From a public health perspective, it is wrong to say that the problem of waste is unsolved. From an economic perspective, it is wrong to say that it is exorbitant. For a volume of HLW (high-level waste) corresponding to a fleet of 58 plants over 40 years, the cost of waste disposal is a few euro cents per MWh.
Still, the main waste is plutonium. It alone constitutes 90% of the radioactivity of long-lived waste. And “conventional” nuclear power plants are constantly generating them. There is a solution to this problem that allows to "stabilise the plutonium inventory": these are the fast neutron reactors. They lead to "closing the fuel cycle" by burning plutonium (from waste to resource) with depleted uranium which is otherwise unusable. The plutonium produced is burnt with depleted uranium and is regenerated in the process. This increases fuel reserves by a factor 1000, which is important in terms of security of supply. The volume of waste is divided by 10, which is important for the management of the cycle downstream. The need for "fast" (high energy) neutrons for fast neutron reactors means that water has to be abandoned as a heat transfer fluid and has led to the use of liquid sodium, which is not an easy technology (especially in terms of nondestructive testing of equipments), but which is at an advanced stage of maturity (the Russians have two such reactors, the Chinese launched theirs at the same time as we shut down ours, Superphenix in 1998).
France had a clear technological lead on this subject, internationally recognised, which was scuttled twice, by the shutdown of Superphénix in 1998, and by the shutdown of the Astrid programme in 2018. Again, the reason is purely political. The one officially put forward is that fast neutron reactors are more expensive and will only be profitable if the price of uranium is tripled. This calculation ignores the disposal costs of plutonium and depleted uranium, which will require the development of much larger disposal sites. This choice also overlooks the increased scarcity of uranium that the development of the Chinese fleet suggests. The unmentionable reason is the will to eventually get out of nuclear power by limiting the resource and multiplying waste. This is without having any certainty that we will be able to do without nuclear power.
In this section, we focused on the uranium-based cycle, which is the one that has been developed in France and around the world. There are other cycles, based on thorium, which are not at this level of maturity, but which have potential benefits, deserving to be explored for exploratory purposes.
2.3. Dismantling power plants
Another aspect of nuclear power that is repeated ad nauseam is our inability to dismantle end-of-life nuclear facilities. This assertion is simply not accurate. At the end of 2013, 149 nuclear reactors had been permanently shut down worldwide, 119 were in the process of being dismantled and 6 had been decommissioned with a "return to green" (i.e. the level of natural radioactivity in the soil). In France, the Brennilis reactor (a heavy-water type) has not yet completed its dismantling, but for legal reasons and a procedural obstinacy of anti-nuclear associations which has nothing to do with the technique. However, the Superphenix reactor could be decommissioned without any problems between 1998 and 2010. And the decommissioning of the Grenoble study reactors has been successfully completed in about ten years.
The question of dismantling must be broken down into three cases. The dismantling of "standard" facilities (generally power plants) which does not pose any serious technical problems (except perhaps for the management of graphite in the old graphite-gas plants) and requires above all rigour and method. The dismantling of "unique" facilities (usually research equipment), which requires ad hoc solutions that are costly and require technical innovations. The dismantling of facilities in accident situations (Chernobyl, Fukushima) for which we have, to date, only containment solutions and not dismantling.
Another cultivated myth is that dismantling would create jobs. It is either naive or deceitful to think that we are going to create jobs with a dismantling activity that employs ten times fewer people, and for less than half as long, than what is needed to operate a power plant. As for thinking that this dismantling skill will be sold for export, it amounts to assuming that those of our industrial competitors who built the plants will be less capable than us to dismantle them!
The intermediate conclusions of this article, following this summary of the facts, are as follows. Nuclear energy provides essentially carbon-free, controllable electricity. This electricity is safe from a public health perspective as evidenced by the number of deaths per TWh produced. The waste it generates is controlled and the technologies to process it are operational. Deep geological repository helps to manage the problem of long-lived waste. The dismantling of end-of-life nuclear power plants is not a technical problem, it is a regulatory problem.
3. Towards a systemic approach
The fight against global warming is a global and systemic problem. Global, because actions carried out in one part of the world contribute to climate behaviour throughout the world. This trivial observation implies both that the problem can only be dealt with in an international dimension, and that it is easy to dismiss by claiming that the little that a country like France can do is negligible in relation to the overall problem. It should be noted that this is ignoring the potential contribution of French technologies to energy issues in other countries of the world.
Nevertheless, it is true that each country, individually, must do its part of the effort. In France, where one third of greenhouse gas emissions come from transport, one third from building management and one third from industry (including electricity production), claiming to decarbonise an essentially carbon-free electricity system makes little sense.
Moreover, the claim to decarbonise this already carbon-free electricity by developing renewable energies, which are intermittent by nature, creates problems of network stability which require either strengthening the networks, or resorting to massive storage of electricity, or developing a more delocalised management of the electrical vector, or developing a vector other than the electrical vector, for example the hydrogen vector. In any case, these solutions to solve a problem that we create ourselves by rejecting a solution that we already have in place, have a cost.
The structure of electricity prices in France given in Table 4 shows that these issues are not negligible and that the development of strengthened networks is not an easy task.
Compositions of costs per kWh for private consumers
Cost Components | 2006 | 2018 |
---|---|---|
Energy share (cost of production | 43% | 36% |
and commercialisation) | ||
Network share (TRA tolls | 39% | 30% |
transmission and distribution) | ||
Share of taxes (including taxes | 18% | 34% |
to develop | ||
renewables) | ||
Total | 100% | 100% |
The technical aspects are also far from negligible. Currently, the only passive electricity storage solutions are raising the water level in hydroelectric dams, which are limited by the existence of suitable sites. Electrochemical storage has made considerable progress, but still seems better suited to the electrification of transport than to mass storage. There are ongoing studies on the ability of electric vehicles on the grid to smooth out consumption peaks, but this requires a massive development of the hybrid vehicle. Mass storage solutions using electrochemical processes other than batteries are not yet developed on an industrial scale. Hydrogen, which has been presented to us for decades as the miracle solution, faces a double challenge: how to produce it at a competitive price with methane reforming (but it is still of fossil origin and therefore its production emits CO2), how to transport it without excessive losses. If we move towards hot electrolysis from intermittent sources, how do we manage the thermal fatigue of electrolysis devices?
None of these issues are physically impossible, but there are certainly technical difficulties that take time to resolve, and major economic challenges. Embarking on profound transformations of our energy mix without having solved these problems is daring. It is clear that the mere mention of reductions in production costs of such and such an energy source is a very partial view of the issue. Not to mention the fact that we continue to heavily subsidise so-called competitive energies...
Table 5 gives some estimates (Cap Gemini) of the current costs of electricity produced by different technologies. Again, these data are only indicative given the great complexity of the problem. For example, it seems likely that the price of solar photovoltaics will fall to 25 Euros per MWh, but neither storage costs nor grid costs are included. The price of the new nuclear does not take into account the series effects and depends very strongly on the chosen discount rate.
Some tariffs for electricity produced by different technologies
Production method | Price |
---|---|
Former nuclear | 45–80 |
Onshore wind | 50–110 |
Solar (PV) | 55–160 |
Coal | 60–105 |
Offshore wind | 100–160 |
New nuclear | 100–170 |
Hydroelectricity | 40–120 |
(Source Cap Gemini, 2018) in euros per MWh.
But while the economic dimension is essential, there are other aspects that deserve our attention. For a long time, Vaclav Smil in a series of books on energy transitions has insisted on the "implicit content" of different energy sources. Unlike economic estimates, which have a certain degree of arbitrary, the needs in terms of surface area, material and energy required to produce 1 kWh of electricity are physical quantities which can be estimated with a certain degree of reliability, either by feedback or on the basis of their operating principles. Physics forbids wind turbines to be too close together for the simple reason of the conservation of the amount of movement carried by the wind (it was a well-known rule of the Dutch in the 17th century, that a windmilll should not be placed too close to another windmill). The energy content of photovoltaic panels is easy to estimate and feedback gives their lifespan. It is easy to know the amount of steel needed for a nuclear power plant and for a wind turbine tower. And these quantities must be related to the amount of electricity produced by the device during its lifespan. As an illustration, Table 6 shows the area requirements for different energy sources to produce 500TWh, which is the French annual consumption.
Area requirements for different energy sources
Method | Nuclear | Fossil | Solar | Wind | Biomass |
---|---|---|---|---|---|
Footprint km2 | 4 | 10 | 200 | 1200 | 5000 |
Without any surprise to the physicist, we note that nuclear energy is economical in terms of area. This simply means that, despite the gigantic size of the equipment, the energy released comes from the interaction between nucleons which, in terms of energy density, is quite unlike chemical, electro-magnetic or gravitational energies which power other means of electricity production. This concentration of energy also has a consequence: it makes it possible to meet concentrated needs (such as those of cities and heavy industry) without being constrained to overly extensive distribution networks. The same reason explains why the quantity of material (steel, aluminium, copper, concrete, etc.) needed to produce a kWh is, for nuclear energy, 10 times lower than for photovoltaics (200 t per MW of electricity) and 35 times lower than for concentrated solar energy. And when we assess the EROI (Energy Return on Investment), i.e. the energy produced in relation to the energy needed to produce it, the EROI of nuclear energy is 30 times greater than that of photovoltaics.
These few remarks simply highlight the fact that simplistic arguments about the "bare" cost of energies, regardless of the systems they must fit into, without taking into account the needs in terms of space and materials, can be sales pitches, they are not arguments for reflection that are sufficient to make informed decisions.
4. Conclusions
The problem of climate change is global, the means of action are local. The most appropriate solutions may vary from one country to another, from one need to another.
The urgency of the problem requires both the deployment of solutions already available and the launch of coherent research programmes for future solutions.
The goal of decarbonising the economy is a necessary step in the fight against global warming. Of course, energy-saving measures must be put in place (in homes for example, or in transport). An effective strategy is the electrification of the economy from carbon-free electricity.
Nuclear energy already provides essentially carbon-free electricity in France. It is a form of energy that consumes few resources, matter, space and embodied energy for its production. It meets the energy needs of industrial sectors requiring dense and stable energy resources. The coexistence of natural renewable energy and nuclear energy raises questions of "flexibility of the fleet", which implies adaptations of networks, storage facilities and management of power plants.
In order to develop in a sustainable manner, nuclear energy requires a long-term strategy of public investors, as the time scale is not that of the financial markets. And this will only be possible if we drop the ideologically motivated case, unsupported by scientific evidence, that deprives our country of an essential asset in the face of one of the major challenges of our time.