[Changement de phase dans les noyaux planétaires]
The ubiquitous phenomena of crystallization and melting occur in various geophysical contexts across many spatial and temporal scales. In particular, they take place in the iron core of terrestrial planets and moons, profoundly influencing their dynamics and magnetic field generation. Crystallization and melting entail intricate multiphase flows, buoyancy effects, and out-of-equilibrium thermodynamics, posing challenges for theoretical modeling and numerical simulations. Besides, due to the inaccessible nature of the planetary deep interior, our understanding relies on indirect data from seismology, mineral physics, geochemistry, and magnetism. Consequently, phase-change-driven flows in planetary cores constitute a compelling yet challenging area of research. This paper provides an overview of the role of laboratory fluid dynamics experiments in elucidating the solid–liquid phase change phenomena occurring thousands of kilometers beneath our feet and within other planetary depths, along with their dynamic repercussions. Drawing parallel with metallurgy, it navigates through all scales of phase change dynamics, from microscopic processes (nucleation and crystal growth) to macroscopic consequences (solid–liquid segregation and large-scale flows). The review delves into the two primary planetary solidification regimes, top-down and bottom-up, and elucidates the formation of mushy and/or slurry layers in the various relevant configurations. Additionally, it outlines remaining challenges, including insights from ongoing space missions poised to unveil the diverse planetary regimes.
Les phénomènes de cristallisation et de fusion se produisent dans divers contextes géophysiques à diverses échelles spatiales et temporelles. Ils prennent notamment place dans le noyau en fer des planètes telluriques et des lunes, où ils jouent un rôle primordial dans leur dynamique et dans la génération de leur champ magnétique. La cristallisation et la fusion se caractérisent par des écoulements multiphasiques complexes, des effets de flottabilité et une thermodynamique hors équilibre, difficilement accessibles à la modélisation théorique et aux simulations numériques. De plus, les profondeurs planétaires demeurent inaccessibles aux observations directes, et notre compréhension repose sur des données indirectes provenant de la sismologie, de la physique minérale, de la géochimie et du magnétisme. Par conséquent, les écoulements induits par un changement de phase dans les noyaux planétaires constituent un domaine de recherche à la fois passionnant et stimulant. Cet article donne un aperçu du rôle qu’ont les expériences de laboratoire en dynamique des fluides pour élucider des phénomènes de changement de phase solide-liquide se produisant à des milliers de kilomètres sous nos pieds ou dans d’autres planètes, ainsi que de leurs conséquences dynamiques. En établissant des parallèles avec la métallurgie, cet article navigue à travers toutes les échelles de la dynamique du changement de phase, des processus microscopiques (nucléation et cristallisation) à leurs conséquences macroscopiques (ségrégation solide-liquide et écoulements de grande échelle). Nous décrivons en détail les deux principaux régimes de solidification planétaire, descendant et ascendant, ainsi que la formation de couches de solides interconnectées (“mush”) ou dispersées (“slurry”) dans les diverses configurations pertinentes. Enfin, cette revue décrit les défis restants, notamment dans le cadre de missions spatiales en cours qui dévoileront sans aucun doute la diversité des régimes planétaires.
Révisé le :
Accepté le :
Première publication :
Mots-clés : Cristallisation, Changement de phase, Écoulements diphasiques, Noyaux planétaires, Champ magnétique
Ludovic Huguet 1 ; Quentin Kriaa 2 ; Thierry Alboussière 3 ; Michael Le Bars 4
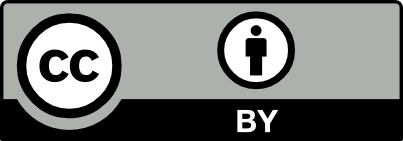
@article{CRPHYS_2024__25_S3_A11_0, author = {Ludovic Huguet and Quentin Kriaa and Thierry Alboussi\`ere and Michael ~Le Bars}, title = {Solid{\textendash}liquid phase change in planetary cores}, journal = {Comptes Rendus. Physique}, publisher = {Acad\'emie des sciences, Paris}, year = {2024}, doi = {10.5802/crphys.216}, language = {en}, note = {Online first}, }
TY - JOUR AU - Ludovic Huguet AU - Quentin Kriaa AU - Thierry Alboussière AU - Michael Le Bars TI - Solid–liquid phase change in planetary cores JO - Comptes Rendus. Physique PY - 2024 PB - Académie des sciences, Paris N1 - Online first DO - 10.5802/crphys.216 LA - en ID - CRPHYS_2024__25_S3_A11_0 ER -
Ludovic Huguet; Quentin Kriaa; Thierry Alboussière; Michael Le Bars. Solid–liquid phase change in planetary cores. Comptes Rendus. Physique, Online first (2024), pp. 1-39. doi : 10.5802/crphys.216.
[1] Numerical modelling of the geodynamo: a systematic parameter study, Geophys. J. Int., Volume 138 (1999), pp. 393-409 | DOI
[2] Sustaining Earth’s magnetic dynamo, Nat. Rev. Earth Environ., Volume 3 (2022) no. 4, pp. 255-269 | DOI
[3] Tidal instability as the source for Io’s magnetic signature, Geophys. Res. Lett., Volume 25 (1998) no. 5, pp. 603-606 | DOI
[4] An impact-driven dynamo for the early Moon, Nature, Volume 479 (2011) no. 7372, pp. 215-218 | DOI
[5] A long-lived lunar dynamo driven by continuous mechanical stirring, Nature, Volume 479 (2011) no. 7372, pp. 212-214 | DOI
[6] Flows driven by libration, precession, and tides, Annu. Rev. Fluid Mech., Volume 47 (2015) no. 1, pp. 163-193 | DOI
[7] Fluid dynamics experiments for planetary interiors, Surv. Geophys., Volume 43 (2022) no. 1, pp. 229-261 | DOI
[8] Iron snow, crystal floats, and inner-core growth: modes of core solidification and implications for dynamos in terrestrial planets and moons, Prog. Earth Planet. Sci., Volume 2 (2015) no. 1, 39 | DOI
[9] Interiors of Earth-like planets and satellites of the solar system, Surv. Geophys., Volume 43 (2022) no. 1, pp. 177-226 | DOI
[10] Seismically determined elastic parameters for Earth’s outer core, Sci. Adv., Volume 4 (2018) no. 6, eaar2538 | DOI
[11] Seismic insights into Earth’s core, Nat. Commun., Volume 14 (2023) no. 1, 6029 | DOI
[12] Heterogeneity and anisotropy of Earth’s inner core, Annu. Rev. Earth Planet. Sci., Volume 42 (2014) no. 1, pp. 103-126 | DOI
[13] Seismic detection of the lunar core, Science, Volume 331 (2011) no. 6015, pp. 309-312 | DOI
[14] Very preliminary reference Moon model, Phys. Earth Planet. Inter., Volume 188 (2011) no. 1, pp. 96-113 | DOI
[15] et al. Lunar seismology: An update on interior structure models, Space Sci. Rev., Volume 215 (2019), pp. 1-47 | DOI
[16] et al. Seismic detection of the martian core, Science, Volume 373 (2021) no. 6553, pp. 443-448 | DOI
[17] et al. Geophysical evidence for an enriched molten silicate layer above Mars’s core, Nature, Volume 622 (2023) no. 7984, pp. 712-717 | DOI
[18] et al. First observations of core-transiting seismic phases on Mars, Proc. Natl. Acad. Sci. USA, Volume 120 (2023) no. 18, e2217090120 | DOI
[19] Solidification processing, Metall. Mater. Trans. B, Volume 5 (1974), pp. 2121-2134 | DOI
[20] Fundamentals of Solidification, Trans Tech Publications, 2023 | DOI
[21] On the existence and structure of a mush at the inner core boundary of the Earth, Phys. Earth Planet Inter., Volume 164 (2007) no. 1, pp. 36-49 | DOI
[22] Electric currents in molten metals and their interaction with a magnetic field, Proceedings of the I.U.T.A.M. Symposium “Metallurgical Applications of MHD”, 1982, pp. 234-245
[23] Influence of a magnetic field on the solidification of metallic alloys, C. R. Acad. Sci. Paris, Volume 313 (1991), pp. 749-755 | DOI
[24] Modification of interdendritic convection in directional solidification by a uniform magnetic field, Acta Mater., Volume 46 (1998) no. 11, pp. 4067-4079 | DOI
[25] Composition and state of the core, Annu. Rev. Earth Planet. Sci., Volume 41 (2013), pp. 657-691 | DOI
[26] The thermal evolution of Mercury’s Fe–Si core, Earth Planet. Sci. Lett., Volume 482 (2018), pp. 147-159 | DOI
[27] Structure of eutectic Fe–FeS melts to pressures up to 17 GPa: implications for planetary cores, Earth Planet. Sci. Lett., Volume 263 (2007) no. 1–2, pp. 128-139 | DOI
[28] The Fe-rich liquidus in the Fe–FeS system from 1 bar to 10 GPa, Geochim. Cosmochim. Acta, Volume 75 (2011) no. 8, pp. 2072-2087 | DOI
[29] The top-down crystallisation of Mercury’s core, Earth Planet. Sci. Lett., Volume 528 (2019), 115838 | DOI
[30] Melting phase relations in the Fe–S and Fe–SO systems at core conditions in small terrestrial bodies, Icarus, Volume 306 (2018), pp. 150-162 | DOI
[31] Melting phase relations in Fe–Si–H at high pressure and implications for Earth’s inner core crystallization, Sci. Rep., Volume 12 (2022) no. 1, 10000 | DOI
[32] Solidification of lunar core from melting experiments on the Fe–Ni–S system, Earth Planet. Sci. Lett., Volume 530 (2020), 115834 | DOI
[33] Liquidus determination of the Fe–S and (Fe, Ni)–S systems at 14 and 24 GPa: Implications for the Mercurian core, Earth Planet. Sci. Lett., Volume 599 (2022), 117865 | DOI
[34] Two-phase mixture of iron–nickel–silicon alloys in the Earth’s inner core, Commun. Earth Environ., Volume 2 (2021) no. 1, 225 | DOI
[35] et al. Phase relations in the system Fe–Ni–Si to 200 GPa and 3900 K and implications for Earth’s core, Earth Planet. Sci. Lett., Volume 512 (2019), pp. 83-88 | DOI
[36] High-pressure and high-temperature phase diagram for Fe0.9Ni0.1–H alloy, Phys. Earth Planet. Inter., Volume 228 (2014), pp. 192-201 (High-Pressure Research in Earth Science: Crust, Mantle, and Core) | DOI
[37] 8.02—Energetics of the core, Treatise on Geophysics (G. Schubert, ed.), Elsevier, Oxford, 2015, pp. 27-55 | DOI
[38] Structure of the F layer and reasons for convection in the Earth’s core, Doklady Akad. Nauk SSSR, Volume 149 (1963), pp. 8-10
[39] The strength and efficiency of thermal and compositional convection in the geodynamo, Phys. Earth Planet. Inter., Volume 91 (1995) no. 1–3, pp. 17-30 | DOI
[40] Iron snow in the Martian core?, Earth Planet. Sci. Lett., Volume 481 (2018), pp. 189-200 | DOI
[41] The Earth’s inner core, Nature, Volume 172 (1953) no. 4372, pp. 297-298 | DOI
[42] Sulfur’s impact on core evolution and magnetic field generation on Ganymede, J. Geophys. Res., Volume 111 (2006) no. E9, pp. 2156-2202 | DOI
[43] High-pressure iron-sulfur compound, Fe3S2, and melting relations in the Fe–FeS system, Science, Volume 275 (1997) no. 5306, pp. 1621-1623 | DOI
[44] Eutectic melting in the system Fe–S to 44 GPa, Earth Planet. Sci. Lett., Volume 257 (2007) no. 1, pp. 97-103 | DOI
[45] Geodesy constraints on the interior structure and composition of Mars, Icarus, Volume 213 (2011) no. 2, pp. 451-472 | DOI
[46] Mercury’s inner core size and core-crystallization regime, Icarus, Volume 248 (2015), pp. 254-268 | DOI
[47] The Fe snow regime in Ganymede’s core: a deep-seated dynamo below a stable snow zone, J. Geophys. Res. Planets, Volume 120 (2015), pp. 1095-1118 | DOI
[48] Top-down freezing in a Fe–FeS Core and Ganymede’s present-day magnetic field, Icarus, Volume 307 (2018), pp. 172-196 | DOI
[49] Earth’s inner core nucleation paradox, Earth Planet. Sci. Lett., Volume 487 (2018), pp. 9-20 | DOI
[50] Simulation of supercooling and size distribution in frazil ice dynamics, Cold Reg. Sci. Technol., Volume 22 (1994) no. 3, pp. 221-233 | DOI
[51] A laboratory model for iron snow in planetary cores, Geophys. Res. Lett., Volume 50 (2023) no. 24, e2023GL105697 | DOI
[52] A nonequilibrium theory of a slurry, Continuum Mech. Thermodyn., Volume 4 (1992) no. 3, pp. 213-245 | DOI | Zbl
[53] A two-phase pure slurry model for planetary cores: one-dimensional solutions and implications for Earth’s F-layer, J. Fluid Mech., Volume 976 (2023), A5 | DOI
[54] Iron snow dynamo models for Ganymede, Icarus, Volume 247 (2015), pp. 248-259 | DOI
[55] A prismatic substructure formed during solidification of metals, Can. J. Phys., Volume 31 (1953) no. 1, pp. 15-39 | DOI
[56] Stability of a planar interface during solidification of a dilute binary alloy, J. Appl. Phys., Volume 35 (1964), pp. 444-451 | DOI
[57] On crystallization at the inner core boundary, Phys. Earth Planet. Inter., Volume 151 (2005) no. 1, pp. 37-51 | DOI
[58] The Theory of Transformations in Metals and Alloys, Elsevier, Newnes, 2002
[59] Non-ideal liquidus curve in the Fe–S system and Mercury’s snowing core, Geophys. Res. Lett., Volume 35 (2008) no. 7, L07201 | DOI
[60] The age of the inner core, Earth Planet. Sci. Lett., Volume 190 (2001), pp. 111-123 | DOI
[61] Structure of a mushy layer under hypergravity with implications for Earth’s inner core, Geophys. J. Int., Volume 204 (2016) no. 3, pp. 1729-1755 | DOI
[62] Structure of the Earth’s inner core, Nature, Volume 292 (1981), pp. 232-233 | DOI
[63] A regime diagram for the slurry F-layer at the base of Earth’s outer core, Earth Planet. Sci. Lett., Volume 560 (2021), 116791 | DOI
[64] Towards a theory of the structure and evolution of a dendrite layer, Stellar and Planetary Magnetism, Gordon & Breach Science Publishers Ltd, 1983 (ISBN-10: 0677164300, ISBN-13: 978-0677164304)
[65] Asteroid core crystallization by inward dendritic growth, J. Geophys. Res., Volume 97 (1992) no. E9, pp. 14727-14734 | DOI
[66] Evolution of Asteroidal Cores, University of Arizona Press, 2006, pp. 747-771
[67] Cristallisation et convection sous hyper-gravité, PhD thesis, Ecole Normale Supérieure de Lyon (2014)
[68] On origin of equiaxed zone in castings, Trans. Metall. Soc. AIME, Volume 236 (1966) no. 2, pp. 149-158
[69] Formation of crystal nuclei in liquid metals, J. Appl. Phys., Volume 21 (1950) no. 10, pp. 1022-1028 | DOI
[70] Undercooling phenomena in liquid metal droplets, J. Aust. Inst. Metals, Volume 10 (1965) no. 3, pp. 220-222
[71] Surface tension measurements on oscillating droplets of undercooled liquid metals and alloys, Undercooled Alloy Phases (E. W. Collings; C. C. Koch, eds.), The Metall. Soc. AIME, 1987, pp. 233-248
[72] Supercooling of liquids, Proc. R. Soc. Lond. A., Volume 215 (1952) no. 1120, pp. 43-46 | DOI
[73] First X-ray scattering studies on electrostatically levitated metallic liquids: demonstrated influence of local icosahedral order on the nucleation barrier, Phys. Rev. Lett., Volume 90 (2003) no. 19, 195504 | DOI
[74] Icosahedral short-range order in deeply undercooled metallic melts, Phys. Rev. Lett., Volume 89 (2002) no. 7, 075507 | DOI
[75] Ab initio simulations of geometrical frustration in supercooled liquid Fe and Fe-based metallic glass, Phys. Rev. B, Volume 77 (2008) no. 1, 014205 | DOI
[76] Icosahedral ordering in liquid iron studied via x-ray scattering and Monte Carlo simulations, Phys. Rev. B, Volume 80 (2009) no. 18, 180201 | DOI
[77] Metallic phase with long-range orientational order and no translational symmetry, Phys. Rev. Lett., Volume 53 (1984) no. 20, 1951 | DOI
[78] Short-range order in undercooled metallic liquids, Mater. Sci. Eng.: A, Volume 375 (2004), pp. 98-103 | DOI
[79] 2.22—Properties of rocks and minerals, high-pressure melting, Treatise on Geophysics (G. Schubert, ed.), Elsevier, Oxford, 2015, pp. 573-582 | DOI
[80] Influence of Cr on the nucleation of primary Al and formation of twinned dendrites in Al–Zn–Cr alloys: Can icosahedral solid clusters play a role?, Acta Mater., Volume 61 (2013) no. 19, pp. 7098-7108 | DOI
[81] et al. X-ray and electrostatic levitation undercooling studies in Ti–Zr–Ni quasicrystal forming alloys, J. Non-Cryst. Solids, Volume 312 (2002), pp. 305-308 | DOI
[82] et al. Demonstration of the effect of stirring on nucleation from experiments on the International Space Station using the ISS-EML facility, NPJ Microgravity, Volume 7 (2021) no. 1, 31 | DOI
[83] et al. Electromagnetic levitation containerless processing of metallic materials in microgravity: rapid solidification, NPJ Microgravity, Volume 9 (2023) no. 1, 65 | DOI
[84] et al. Shock melting curve of iron: A consensus on the temperature at the Earth’s inner core boundary, Geophys. Res. Lett., Volume 47 (2020) no. 15, e2020GL087758 | DOI
[85] et al. Measuring the melting curve of iron at super-Earth core conditions, Science, Volume 375 (2022) no. 6577, pp. 202-205 | DOI
[86] Implications of the homogeneous nucleation barrier for top-down crystallization in Mercury’s Core, Mercury: Current and Future Science of the Innermost Planet, Volume 2047, Lunar and Planetary Institute, 2018, p. 6101
[87] Can homogeneous nucleation resolve the inner core nucleation paradox?, Earth Planet. Sci. Lett., Volume 614 (2023), 118176 | DOI
[88] Probing the nucleation of iron in Earth’s core using molecular dynamics simulations of supercooled liquids, Phys. Rev. B, Volume 103 (2021) no. 21, 214113 | DOI
[89] Two-step nucleation of the Earth’s inner core, Proc. Natl. Acad. Sci. USA, Volume 119 (2022) no. 2, e2113059119 | DOI
[90] Assessing the inner core nucleation paradox with atomic-scale simulations, Earth Planet. Sci. Lett., Volume 507 (2019), pp. 1-9 | DOI
[91] The fate of liquids trapped during the earth’s inner core growth, Geophys. Res. Lett., Volume 47 (2020) no. 2, e2019GL085654 | DOI
[92] Multiphase/-scale modeling of alloy solidification, Annu. Rev. Heat Transfer, Volume 6 (1995), pp. 115-198 | DOI
[93] Convection in mushy layers, Annu. Rev. Fluid Mech., Volume 29 (1997) no. 1, pp. 91-122 | DOI
[94] Sea ice is a mushy layer, Geophys. Res. Lett., Volume 33 (2006) no. 14, L14501 | DOI
[95] The origin of freckles in unidirectionally solidified castings, Metall. Trans., Volume 1 (1970) no. 8, pp. 2193-2204 | DOI
[96] Channel formation in Pb–Sb, Pb–Sn, and Pb–Sn–Sb alloy ingots and comparison with the system NH
[97] Experimental study of directional solidification of aqueous ammonium chloride solution, J. Fluid Mech., Volume 227 (1991), pp. 567-586 | DOI
[98] Compositional convection in a reactive crystalline mush and melt differentiation, J. Geophys. Res., Volume 97 (1992) no. B5, pp. 6735-6756 | DOI
[99] The transient behaviour of alloys solidified from below prior to the formation of chimneys, J. Fluid Mech., Volume 269 (1994), pp. 23-44 | DOI
[100] Natural convection, solute trapping, and channel formation during solidification of saltwater, J. Phys. Chem. B, Volume 101 (1997) no. 32, pp. 6132-6136 | DOI
[101] A laboratory model for solidification of Earth’s core, Phys. Earth Planet. Inter., Volume 153 (2005) no. 1–3, pp. 150-164 (Studies of the Earth’s Deep Interior SEDI 2004) | DOI
[102] Convection and channel formation in solidifying Pb–Sn alloys, Metall. Mater. Trans. A, Volume 28 (1997) no. 3, pp. 859-866 | DOI
[103] A study of conditions at the inner core boundary of the Earth, Phys. Earth Planet. Inter., Volume 24 (1981) no. 4, pp. 302-307 | DOI
[104] Instabilities of the liquid and mushy regions during solidification of alloys, J. Fluid Mech., Volume 237 (1992), pp. 649-669 | DOI | Zbl
[105] Maximal potential energy transport: A variational principle for solidification problems, Phys. Rev. Lett., Volume 105 (2010) no. 25, 254502 | DOI
[106] Brine fluxes from growing sea ice, Geophys. Res. Lett., Volume 38 (2011) no. 4, L04501 | DOI
[107] Nonlinear mushy-layer convection with chimneys: stability and optimal solute fluxes, J. Fluid Mech., Volume 716 (2013), pp. 203-227 | DOI | Zbl
[108] Fluxes through steady chimneys in a mushy layer during binary alloy solidification, J. Fluid Mech., Volume 714 (2013), pp. 127-151 | DOI
[109] Flow of Gases Through Porous Media, Academic Press, 1956
[110] Permeability prediction for flow normal to columnar solidification structures by large–scale simulations of phase–field and lattice Boltzmann methods, Acta Mater., Volume 164 (2019), pp. 237-249 | DOI
[111] Natural convection in a mushy layer, J. Fluid Mech., Volume 224 (1991), pp. 335-359 | DOI | Zbl
[112] Convective fluid motion within the interdendritic liquid of a casting, Metall. Mater. Trans. B, Volume 1 (1970) no. 6, pp. 1787-1788 | DOI
[113] Planet(oid) core crystallisation and fractionation-evidence from the Agpalilik mass of the Cape York iron meteorite shower, Phys. Earth Planet. Inter., Volume 29 (1982) no. 3, pp. 218-232 | DOI
[114] Chimneys on the Earth’s inner-outer core boundary?, Geophys. Res. Lett., Volume 21 (1994) no. 6, pp. 477-480 | DOI
[115] Suppression of channel convection in solidifying Pb–Sn alloys via an applied magnetic field, Metall. Mater. Trans. A, Volume 30 (1999) no. 7, pp. 1809-1815 | DOI
[116] The development of preferred orientations during the freezing of metals and alloys, Proc. R. Soc. Lond.. Series A. Math. Phys. Sci., Volume 269 (1962) no. 1339, pp. 560-573 | DOI
[117] Further generalization of Onsager’s cascade model for turbulent spectra, Phys. Fluids, Volume 7 (1964), pp. 1156-1159 | DOI
[118] The structure of iron in Earth’s inner core, Science, Volume 330 (2010) no. 6002, pp. 359-361 | DOI
[119] The stability of bcc-Fe at high pressures and temperatures with respect to tetragonal strain, Phys. Earth Planet. Inter., Volume 170 (2008) no. 1–2, pp. 52-59 | DOI
[120] Stabilization of body-centred cubic iron under inner-core conditions, Nat. Geosci., Volume 10 (2017) no. 4, pp. 312-316 | DOI
[121] Preferred crystal orientations due to melt convection during directional solidification, J. Geophys. Res.: Solid Earth, Volume 107 (2002) no. B9, ECV-6 | DOI
[122] Measurements of electric anisotropy due to solidification texturing and the implications for the Earth’s inner core, Nature, Volume 389 (1997) no. 6646, pp. 60-63 | DOI
[123] Estimates of the Earth’s inner core grain size, Geophys. Res. Lett., Volume 25 (1998) no. 10, pp. 1593-1596 | DOI
[124] A model for sedimentary compaction of a viscous medium and its application to inner-core growth, Geophys. J. Int., Volume 124 (1996) no. 2, pp. 502-524 | DOI
[125] Core solidification and dynamo evolution in a mantle-stripped planetesimal, J. Geophys. Res., Volume 121 (2016) no. 1, pp. 2-20 | DOI
[126] The top-down solidification of iron asteroids driving dynamo evolution, J. Geophys. Res., Volume 124 (2019) no. 5, pp. 1331-1356 | DOI
[127] Stability analysis on convection in directional solidification of binary solutions (invited review paper), Proc.-Nat. Sci. Council Republic of Chin. Part a Phys. Sci. Eng., Volume 25 (2001) no. 2, pp. 71-83
[128] Dynamique de la cristallisation de la graine: expériences et modèles, PhD thesis, Université Joseph-Fourier-Grenoble I (2009)
[129] Simultaneous optical measurement of temperature and velocity fields in solidifying liquids, Exp. Fluids, Volume 61 (2020), pp. 1-19 | DOI
[130] Grain refinement induced by electromagnetic stirring: A dendrite fragmentation criterion, Metall. Mater. Trans. A, Volume 35 (2004), pp. 3201-3210 | DOI
[131] On the coupling mechanism of equiaxed crystal generation with the liquid flow driven by natural convection during solidification, Metall. Mater. Trans. A, Volume 49 (2018), pp. 1708-1724 | DOI
[132] Viscosity of hcp iron at Earth’s inner core conditions from density functional theory, Sci. Rep., Volume 10 (2020) no. 1, 6311 | DOI
[133] Laboratory experiments of slab break-off and slab dip reversal: insight into the Alpine Oligocene reorganization, Terra Nova, Volume 20 (2008) no. 4, pp. 267-273 | DOI
[134] Dynamics of slab detachment, Geochem. Geophys. Geosyst., Volume 13 (2012) no. 3, Q03020 | DOI
[135] Abrupt tectonics and rapid slab detachment with grain damage, Proc. Natl. Acad. Sci. USA, Volume 112 (2015) no. 5, pp. 1287-1291 | DOI
[136] Frazil Ice Dynamics, 84, US Army Corps of Engineers, Cold Regions Research & Engineering Laboratory, 1984
[137] The velocity profile at the base of the liquid core from PKP(BC+Cdiff) data: An argument in favour of radial inhomogeneity, Geophys. Res. Lett., Volume 18 (1991) no. 11, pp. 2023-2026 | DOI
[138] Independent estimate of velocity structure of Earth’s lowermost outer core beneath the northeast Pacific from PKiKP- PKPbc differential traveltime and dispersion in PKPbc, J. Geophys. Res.: Solid Earth, Volume 120 (2015) no. 11, pp. 7572-7586 | DOI
[139] Preliminary reference Earth model, Phys. Earth Planet. Inter., Volume 25 (1981) no. 4, pp. 297-356 | DOI
[140] Melting-induced stratification above the Earth’s inner core due to convective translation, Nature, Volume 466 (2010) no. 7307, pp. 744-747 | DOI
[141] Lopsided growth of Earth’s inner core, Science, Volume 328 (2010) no. 5981, pp. 1014-1017 | DOI
[142] Melting of the Earth’s inner core, Nature, Volume 473 (2011) no. 7347, pp. 361-363 | DOI
[143] Crystallization and layering induced by heating a reactive porous medium, Geophys. Res. Lett., Volume 31 (2004) no. 13, L13605 | DOI
[144] Dissolution-driven convection in a reactive porous medium, J. Fluid Mech., Volume 535 (2005), pp. 255-285 | DOI | Zbl
[145] Partial melting of a Pb–Sn mushy layer due to heating from above, and implications for regional melting of Earth’s directionally solidified inner core, Geophys. Res. Lett., Volume 42 (2015), pp. 7046-7053 | DOI
[146] Formation of double-diffusive layers in the directional solidification of binary solution, J. Cryst. Growth, Volume 179 (1997) no. 1–2, pp. 277-286 | DOI
[147] Lituya Bay landslide impact generated mega-tsunami 50 th Anniversary, Tsunami Science Four Years after the 2004 Indian Ocean Tsunami: Part II: Observation and Data Analysis, Birkhäuser, Basel, 2009, pp. 153-175 | DOI
[148] High-resolution simulations of particle-driven gravity currents, Int. J. Multiphase Flow, Volume 28 (2002) no. 2, pp. 279-300 | DOI | Zbl
[149] Turbidity currents propagating down a slope into a stratified saline ambient fluid, Environ. Fluid Mech., Volume 19 (2019) no. 5, pp. 1143-1166 | DOI
[150] GEODAR data and the flow regimes of snow avalanches, J. Geophys. Res.: Earth Surf., Volume 123 (2018) no. 6, pp. 1272-1294 | DOI
[151] Multiphase Flows with Droplets and Particles, 2, CRC Press, Boca Raton, 2011 | DOI
[152] Two-way coupling Eulerian numerical simulations of particle clouds settling in a quiescent fluid, Phys. Rev. Fluids, Volume 8 (2023) no. 7, 074302 | DOI
[153] Entrainment into particle-laden turbulent plumes, Phys. Rev. Fluids, Volume 6 (2021) no. 12, 123502 | DOI
[154] Settling of localized particle plumes in a quiescent water tank, Phys. Rev. Fluids, Volume 8 (2023) no. 2, 024301 | DOI
[155] The effect of double diffusion on entrainment in turbulent plumes, J. Fluid Mech., Volume 884 (2020), A6 | DOI
[156] Dynamic behaviour of particle clouds, 11th Australasian Fluid Mechanics Conference University of Tasmania, Hobart, Australia, 1992
[157] Dynamics of sediment clouds related to open-water sediment disposal, PhD thesis, MIT (2000)
[158] Particle clouds in homogeneous and stratified environments, J. Fluid Mech., Volume 489 (2003), pp. 29-54 | DOI | Zbl
[159] Effect of air release height on the formation of sediment thermals in water, J. Hydraul. Res., Volume 50 (2012) no. 5, pp. 532-540 | DOI
[160] Two-phase modeling of sediment clouds, Environ. Fluid Mech., Volume 13 (2013) no. 5, pp. 435-463 | DOI
[161] Experiments on the fragmentation of a buoyant liquid volume in another liquid, J. Fluid Mech., Volume 749 (2014), pp. 478-518 | DOI
[162] Bubble-laden thermals in supersaturated water, J. Fluid Mech., Volume 924 (2021), A31 | DOI
[163] Effects of particle size and background rotation on the settling of particle clouds, Phys. Rev. Fluids, Volume 7 (2022) no. 12, 124302 | DOI
[164] Experiments on turbulent metal-silicate mixing in a magma ocean, Earth Planet. Sci. Lett., Volume 310 (2011) no. 3, pp. 303-313 | DOI
[165] Incompressible Rayleigh–Taylor turbulence, Annu. Rev. Fluid Mech., Volume 49 (2017) no. 1, pp. 119-143 | DOI
[166] Influence of convective sedimentation on the formation of widespread tephra fall layers in the deep sea, Geology, Volume 25 (1997) no. 9, pp. 839-842 | DOI
[167] The influence of particle concentration on the formation of settling-driven gravitational instabilities at the base of volcanic clouds, Front. Earth Sci., Volume 9 (2021), 640090 | DOI
[168] Particle-generated turbulence in homogeneous dilute dispersed flows, Int. J. Multiph. Flow, Volume 18 (1992) no. 3, pp. 397-412 | DOI | Zbl
[169] Vertical density currents: a review of their potential role in the deposition and interpretation of deep-sea ash layers, J. Geol. Soc., Volume 161 (2004) no. 6, pp. 947-958 | DOI
[170] Particle-like and fluid-like settling of a stratified suspension, Eur. Phys. J. E, Volume 35 (2012) no. 1, 1 | DOI
[171] Numerical simulation of concentration interface in stratified suspension: continuum–particle transition, Int. J. Multiph. Flow, Volume 73 (2015), pp. 71-79 | DOI
[172] Cell formation in the convective mixed layer, Fluid Dyn. Res., Volume 3 (1988) no. 1, pp. 395-399 | DOI
[173] Large-scale simulations of bubble-induced convection in a liquid layer, Phys. Rev. Lett., Volume 82 (1999) no. 24, pp. 4827-4830 | DOI
[174] Buoyancy-driven bubbly flows: scaling of velocities in bubble columns operated in the heterogeneous regime, J. Fluid Mech., Volume 952 (2022), A10 | DOI
[175] Gravity-driven bubbly flows, Annu. Rev. Fluid Mech., Volume 37 (2005) no. 1, pp. 393-423 | DOI
[176] Convection driven by collective buoyancy of microbubbles, Fluid Dyn. Res., Volume 39 (2007) no. 1, pp. 68-97 | DOI | Zbl
[177] Equiaxed dendritic solidification with convection: Part III. Comparisons with NH
[178] Simultaneous observation of melt flow and motion of equiaxed crystals during solidification using a dual phase particle image velocimetry technique. Part I: Stage characterization of melt flow and equiaxed crystal motion, Metall. Mater. Trans. A, Volume 44 (2013), pp. 650-660 | DOI
[179] Effect of melt convection and solid transport on macrosegregation and grain structure in equiaxed Al–Cu alloys, Mater. Sci. Eng.: A, Volume 347 (2003) no. 1–2, pp. 186-197 | DOI
[180] Modeling equiaxed solidification with melt convection and grain sedimentation-I: Model description, Acta Mater., Volume 57 (2009) no. 19, pp. 5621-5631 | DOI
[181] Modelling of macrosegregation: applications and future needs, Int. Mater. Rev., Volume 47 (2002) no. 5, pp. 243-261 | DOI
[182] Two-dimensional large-scale phase-field lattice Boltzmann simulation of polycrystalline equiaxed solidification with motion of a massive number of dendrites, Comput. Mater. Sci., Volume 178 (2020), 109639 | DOI
[183] Multi-phase-field lattice Boltzmann model for polycrystalline equiaxed solidification with motion, Comput. Mater. Sci., Volume 197 (2021), 110658 | DOI
[184] Large-scale phase-field simulations for dendrite growth: A review on current status and future perspective, IOP Conference Series: Materials Science and Engineering, Volume 1274, IOP Publishing, 2023 | DOI
[185] Experimental study of free growth of equiaxed NH4Cl crystals settling in undercooled NH
[186] Growth of equiaxed dendritic crystals settling in an undercooled melt, Part 1: Tip kinetics, J. Cryst. Growth, Volume 309 (2007) no. 2, pp. 197-215 | DOI
[187] Dendrite fragmentation and the effects of fluid flow in castings, Jom, Volume 49 (1997) no. 3, pp. 18-20 | DOI
[188] Massive formation of equiaxed crystals by avalanches of mushy zone segments, Metall. Mater. Trans. A, Volume 48 (2017), pp. 2927-2931 | DOI
[189] Spectral random masking: a novel dynamic masking technique for PIV in multiphase flows, Exp. Fluids, Volume 60 (2019), pp. 1-6 | DOI
[190] A Boussinesq slurry model of the F-layer at the base of Earth’s outer core, Geophys. J. Int., Volume 214 (2018) no. 3, pp. 2236-2249 | DOI
[191] On the motion of an iron-alloy core containing a slurry: I. General theory, Geophys. Astrophys. Fluid Dyn., Volume 9 (1977) no. 1, pp. 289-321 | DOI
[192] Laboratory experiments on rain-driven convection: implications for planetary dynamos, Earth Planet. Sci. Lett., Volume 457 (2017), pp. 403-411 | DOI
[193] Melting and dissolving of a vertical solid surface with laminar compositional convection, J. Fluid Mech., Volume 687 (2011), pp. 118-140 | DOI | Zbl
[194] Thin film modeling of crystal dissolution and growth in confinement, Phys. Rev. E, Volume 97 (2018) no. 1, 012802 | arXiv | DOI
[195] Rayleigh–Bénard convection with a melting boundary, J. Fluid Mech., Volume 858 (2019), pp. 437-473 | DOI
[196] Complete dissolution of spherical particles in free-fall, Chem. Eng. Sci., Volume 34 (1979) no. 6, pp. 847-852 | DOI
[197] Transpiration effects in solids dissolution, Chem. Eng. Sci., Volume 37 (1982) no. 10, pp. 1465-1469 | DOI
[198] Mass and heat transfer during solid sphere dissolution in a non-uniform fluid flow, Heat Mass Transf., Volume 41 (2005) no. 5, pp. 442-448 | DOI
[199] Dissolution of a solid sphere in an unbounded, stagnant liquid, Chem. Eng. Sci., Volume 61 (2006) no. 2, pp. 775-778 | DOI
[200] Dynamics of a reactive spherical particle falling in a linearly stratified fluid, Phys. Rev. Fluids, Volume 5 (2020) no. 11, 114803 | DOI
[201] Core mechanisms of drag enhancement on bodies settling in a stratified fluid, J. Fluid Mech., Volume 875 (2019), pp. 622-656 | DOI | Zbl
[202] Sedimentation of a single soluble particle at low Reynolds and high Péclet numbers, Phys. Rev. Fluids, Volume 9 (2024) no. 4, 44502 | DOI
[203] Flows induced by the settling and phase change of particles: iron rain and iron snow in planetary interiors, PhD thesis, Aix-Marseille Universite (2023)
[204] Convective crystal dissolution, Contrib. Mineral. Petrol., Volume 121 (1995) no. 3, pp. 237-246 | DOI
[205] A physical picture for the acoustic resonant drag instability, Mont. Not. R. Astron. Soc., Volume 529 (2024) no. 1, pp. 688-701 | DOI
[206] Texture of the uppermost inner core from forward-and back-scattered seismic waves, Earth Planet. Sci. Lett., Volume 258 (2007) no. 3, pp. 442-453 | DOI
[207] Earth’s solid inner core: Seismic implications of freezing and melting, J. Earth Sci., Volume 24 (2013) no. 5, pp. 683-698 | DOI
[208] Multiscale model of global inner-core anisotropy induced by hcp alloy plasticity, Geophys. Res. Lett., Volume 43 (2016) no. 3, pp. 1084-1091 | DOI
[209] Elastic and attenuation anisotropy in directionally solidified (hcp) zinc, and the seismic anisotropy in the Earth’s inner core, Phys. Earth Planet. Inter., Volume 117 (2000) no. 1, pp. 139-151 | DOI
[210] Experimental crystallization of gallium: ultrasonic measurements of elastic anisotropy and implications for the inner core, Phys. Earth Planet. Inter., Volume 129 (2002) no. 3–4, pp. 325-346 | DOI
[211] Structure and dynamics of Earth’s inner core, Earth Planet. Sci. Lett., Volume 333 (2012), pp. 211-225 | DOI
[212] Dynamic patterns of compaction in brittle porous media, Nat. Phys., Volume 11 (2015) no. 10, pp. 835-838 | DOI
[213] Laboratory measurement of compaction-induced permeability change in porous rocks: Implications for the generation and maintenance of pore pressure excess in the crust, Pure Appl. Geophys., Volume 143 (1994), pp. 425-456 | DOI
[214] Propagating compaction bands in confined compression of snow, Nat. Phys., Volume 13 (2017) no. 3, pp. 272-275 | DOI
[215] Development of a point plume in the presence of background rotation, Phys. Fluids, Volume 10 (1998) no. 9, pp. 2369-2383 | DOI
[216] Hydrothermal plume dynamics on Europa: Implications for chaos formation, J. Geophys. Res., Volume 109 (2004) no. E3, E03008 | DOI
[217] Anticyclonic precession of a plume in a rotating environment, Geophys. Res. Lett., Volume 44 (2017) no. 18, pp. 9400-9407 | DOI
[218] et al. Plumes in rotating fluid and their transformation into tornados, J. Fluid Mech., Volume 924 (2021), A15 | Zbl
[219] Effects of background rotation on the dynamics of multiphase plumes, J. Fluid Mech., Volume 915 (2021), A2 | DOI | Zbl
[220] The motion of a turbulent thermal in the presence of background rotation, J. Atmos. Sci., Volume 51 (1994) no. 13, pp. 1989-1994 | DOI
[221] Thermals with background rotation and stratification, J. Fluid Mech., Volume 259 (1994), pp. 265-280 | DOI
[222] Influence of planetary rotation on metal-silicate mixing and equilibration in a magma ocean, Phys. Earth Planet. Inter., Volume 352 (2024), 107168 | DOI
[223] Two-phase magnetohydrodynamics: Theory and applications to planetesimal cores, Phys. Earth Planet. Inter., Volume 300 (2020), 106432 | DOI
[224] Little earth experiment: an instrument to model planetary cores, Rev. Sci. Instrum., Volume 87 (2016) no. 8, 084502 | DOI
[225] Experimental study of the convection in a rotating tangent cylinder, J. Fluid Mech., Volume 843 (2018), pp. 355-381 | DOI
[226] Convection and mixing in magma chambers, Earth-Sci. Rev., Volume 23 (1986) no. 4, pp. 255-352 | DOI
[227] The fluid dynamics of evolving magma chambers, Philos. Trans. R. Soc. Lond.. Ser. A, Math. Phys. Sci., Volume 310 (1997) no. 1514, pp. 511-534 | DOI
[228] Crystal settling and convection in the Shiant Isles main sill, Contrib. Mineral. Petrol., Volume 172 (2017), 7 | DOI
[229] Magma chambers versus mush zones: constraining the architecture of sub-volcanic plumbing systems from microstructural analysis of crystalline enclaves, Philos. Trans. R. Soc. A: Math. Phys. Eng. Sci., Volume 377 (2019) no. 2139, 20180006 | DOI
[230] Crystallization of interstitial liquid and latent heat buffering in solidifying gabbros: skaergaard intrusion, greenland, J. Petrol., Volume 55 (2014) no. 7, pp. 1389-1427 | DOI
[231] The influence of crystal size distributions on the rheology of magmas: new insights from analog experiments, Geochem. Geophys. Geosyst., Volume 18 (2017) no. 11, pp. 4055-4073 | DOI
[232] Polynya dynamics: a review of observations and modeling, Rev. Geophys., Volume 42 (2004) no. 1, RG1004 | DOI
[233] Frazil ice in rivers and oceans, Annu. Rev. Fluid Mech., Volume 13 (1981) no. 13, pp. 379-397 | DOI
[234] Lagrangian modelling of frazil ice in the ocean, Ann. Glaciol., Volume 56 (2015) no. 69, pp. 373-382 | DOI
[235] Frazil-ice growth rate and dynamics in mixed layers and sub-ice-shelf plumes, The Cryosphere, Volume 12 (2018) no. 1, pp. 25-38 | DOI
[236] High-resolution simulations of interactions between surface ocean dynamics and frazil ice, The Cryosphere, Volume 14 (2020) no. 11, pp. 3707-3729 | DOI
[237] A laboratory study on supercooling and frazil ice production processes in winter coastal polynyas, J. Geophys. Res.: Oceans, Volume 98 (1993) no. C11, pp. 20321-20328 | DOI
[238] Inner structure of anchor ice and ice dams in steep channels, Cold Reg. Sci. Technol., Volume 106–107 (2014), pp. 194-206 | DOI
[239] Understanding frazil ice: the contribution of laboratory studies, Cold Reg. Sci. Technol., Volume 189 (2021), 103334 | DOI
[240] Turbulent stratified interfaces, Phys. Fluids A: Fluid Dyn., Volume 3 (1991) no. 5, pp. 1278-1285 | DOI
[241] Dynamics of downdraughts and cold pools: an experimental and numerical study, PhD thesis, University of Cambridge (2020)
[242] The Eulerian description of dilute collisionless suspension, Europhys. Lett., Volume 78 (2007) no. 1, 14001 | DOI
[243] Layer formation in sedimentary fingering convection, J. Fluid Mech., Volume 816 (2017), pp. 268-305 | DOI | Zbl
[244] Modelling settling-driven gravitational instabilities at the base of volcanic clouds using the lattice boltzmann method, Front. Earth Sci., Volume 9 (2021), 713175 | DOI
[245] Numerical study of particle-induced Rayleigh–Taylor instability: effects of particle settling and entrainment, Phys. Fluids, Volume 28 (2016) no. 4, 043302 | DOI
[246] Two-fluid formulation of the cloud-top mixing layer for direct numerical simulation, Theoret. Comput. Fluid Dyn., Volume 24 (2010) no. 6, pp. 511-536 | DOI | Zbl
[247] Numerical simulation of concentration interface in stratified suspension: continuum–particle transition, Int. J. Multiphase Flow, Volume 73 (2015), pp. 71-79 | DOI
[248] Cloud-top entrainment in stratocumulus clouds, Annu. Rev. Fluid Mech., Volume 49 (2017) no. 49, pp. 145-169 | DOI | Zbl
[249] Physicochemical Hydrodynamics, Prentice-Hall International Series in the Physical and Chemical Engineering Sciences, Prentice-Hall, Englewood Cliffs, NJ, 1962
[250] Radiative transfer, Atmospheric Science (J. M. Wallace; P. V. Hobbs, eds.), Academic Press, San Diego, 2006, pp. 113-152 | DOI
[251] Microphysics of Clouds and Precipitation, Atmospheric and Oceanographic Sciences Library, 18, Springer, Netherlands, Dordrecht, 2010 | DOI
[252] Stratocumulus Clouds, Mon. Weather Rev., Volume 140 (2012) no. 8, pp. 2373-2423 | DOI
[253] Grand challenges in climate research, Front. Environ. Sci., Volume 1 (2013), 1 | DOI
[254] Atmospheric buoyancy-driven flows, Buoyancy-Driven Flows (C. Cenedese; E. P. Chassignet; J. Verron, eds.), Cambridge University Press, Cambridge, 2012, pp. 312-337 | DOI
[255] et al. Spontaneous aggregation of convective storms, Annu. Rev. Fluid Mech., Volume 54 (2022) no. 1, pp. 133-157 | DOI
[256] The phase diagram and transport properties for hydrogen-helium fluid planets, Astrophys. J. Suppl. Ser., Volume 35 (1977), pp. 221-237 | DOI
[257] An Exploration of double diffusive convection in Jupiter as a result of Hydrogen–Helium phase separation, Mont. Not. R. Astron. Soc., Volume 447 (2015) no. 4, pp. 3422-3441 | DOI
[258] Rainy downdrafts in abyssal atmospheres, Astron. Astrophys., Volume 674 (2023), A177 | DOI
[259] Convective storms and atmospheric vertical structure in Uranus and Neptune, Philos. Trans. R. Soc. A: Math. Phys. Eng. Sci., Volume 378 (2020) no. 2187, 20190476 | DOI
[260] Storms and the depletion of ammonia in Jupiter: I. Microphysics of “Mushballs”, J. Geophys. Res.: Planets, Volume 125 (2020) no. 8, e2020JE006403 | DOI
[261] 3D mixing in hot Jupiters atmospheres—I. Application to the day/night cold trap in HD 209458b, Astron. Astrophys., Volume 558 (2013), A91 | DOI
[262] Exoplanet clouds, Annu. Rev. Earth Planet. Sci., Volume 47 (2019), pp. 583-606 | DOI
[263] Clouds and hazes in exoplanet atmospheres, Comp. Climatol. Terr. planets, Volume 1 (2013), pp. 367-391 | DOI
Cité par Sources :
Commentaires - Politique